DOI:
10.1039/C7QM00055C
(Research Article)
Mater. Chem. Front., 2017,
1, 1641-1653
Exfoliated metal free homojunction photocatalyst prepared by a biomediated route for enhanced hydrogen evolution and Rhodamine B degradation†
Received
6th February 2017
, Accepted 11th April 2017
First published on 4th May 2017
Abstract
g-C3N4 has been widely used as a smart material for photocatalytic applications. Due to insufficient solar-light absorption and fast electron–hole recombination, the photocatalytic activity of g-C3N4 remains a challenge for industrial applications. In this study, we prepared an exfoliated g-C3N4 homojunction in a single-step biomediated route, thereby addressing the major issues of g-C3N4. With a well exfoliated and layered sheet structure, g-C3N4 exhibits exceptional physical properties such as ultrahigh intrinsic carrier mobility and pronounced changes in the energy band structure due to weak van der Waals forces between C3N4 layers. The formation of well exfoliated sheets of g-C3N4 was confirmed by XRD, FESEM, TEM and AFM results. More importantly XPS confirmed the synthetic process by the biomediated route, in which some electron acceptor (–CN) groups were easily introduced at the edge of g-C3N4, aiding the in situ p–n homo-junction formation. The g-C3N4 homojunction was confirmed by Mott–Schottky analysis. This homojunction structure was proven by EIS and TRPL analysis to be highly proficient in charge transfer and separation processes. The exfoliated g-C3N4 homojunction showed significantly enhanced photocatalytic activity, compared to that prepared through the conventional route, due to the unique structural advantages, electron transfer, light harvesting, lower recombination rate of charge carriers and the richly available reaction sites during the photocatalytic reaction. g-C3N4 prepared through the one step biomediated route was able to evolve 87 μmol h−1 of H2 and degrade 90.2% of 200 ppm RhB solution, which is significantly higher than bulk g-C3N4 prepared through the conventional route.
Introduction
The voyage of the scientific community to find suitable semiconductor based photocatalysts for energy and environmental sustainability in harnessing solar energy is a noble approach in the present research scenario. Various photocatalysts such as mixed metal oxide, metal oxide nanocomposites, metal sulphides and conjugated organic polymers have been developed for hydrogen production.1–5 Among the various developed semiconductors, the conjugated organic polymer, metal-free g-C3N4, is a suitable candidate for the production of H2/O2 through the water redox reaction and the degradation of organic contaminants under visible light irradiation due to its suitable band edge potential;5 however, fast rates of charge recombination, high exciton binding energy and low surface area are the major obstacles towards it becoming the best photocatalyst.6–11 To mitigate the above problems, various researchers have modified g-C3N4 by non-metal doping, noble metal deposition, forming heterojunctions with other semiconductors and the preparation of nano/porous g-C3N4.12–14
Recently, researchers have focused on two-dimensional (2D) nanosheet materials with few atomic layers because of their unique properties15,16 and prospective applications in the areas of sensors,17 electronics,18 catalysis19 and energy storage.20 With the influential discovery of graphene, the delamination of layered bulk materials into two-dimensional (2D) sheets through the exfoliation technique is the most advantageous route, which induces unique physicochemical properties including increased surface area, ultrahigh charge carrier mobility and definite changes in energy band structure.21–23 In this regard, tremendous study has been carried out by various researchers towards the development of covalent g-C3N4 nanosheets.24,25 Graphitic carbon nitride also possesses some interesting properties similar to graphene, hence g-C3N4 is a potential candidate and can be used as a layered material for the photocatalytic reaction. Compared to bulk g-C3N4, layered and exfoliated g-C3N4 has the potential for application in the field of photocatalysis owing to its large surface area, ultimate two-dimensional anisotropy, excellent optoelectronic properties and short bulk diffusion length for reducing the recombination probability of photo excited charge carriers.26 The synthesis of bulk g-C3N4 is very easy since it can be directly synthesized by the thermal polycondensation of organic precursors containing both carbon and nitrogen, such as cyanamide,27 melamine,28etc. However, the synthetic procedure for exfoliated and layered g-C3N4 is still a challenging task. Yang et al. have synthesized exfoliated g-C3N4 by the liquid exfoliation method.29 For the first time, Zhang et al. reported ultrathin graphitic-phase C3N4 nanosheets for bio-imaging by a “green” liquid exfoliation route from bulk g-C3N4 in water.30 Very recently, Han et al. reported a monolayered g-C3N4 nanomesh by a sonication process, using porous g-C3N4, IPA and water.11 To date, the reported method for the synthesis of exfoliated g-C3N4 is mostly a two-step method that requires a template, is very time consuming and requires a complex reaction procedure. It is very important to develop a facile one step method for the preparation of exfoliated g-C3N4 to fulfil the requirements of the photocatalytic community.
To enhance the charge separation, g-C3N4 mostly combines with other photocatalysts, which enables the migration of photogenerated charge carriers in opposite directions.31,32 Since heterojunction based photocatalysts are fabricated using different types of semiconductors, it is very difficult to employ them in practical application since the formation of heterojunctions is not only governed by the position of respective energy bands but is also drastically affected by their crystal structures, morphology, light absorption properties and surface/interface/bulk properties of the individual semiconductors. In this context the formation of homojunction photocatalysts composed of the same semiconductor materials exhibits more advantages than heterojunction based photocatalysts.32,33 It has been reported that C3N4 is a typical n-type material due to the presence of terminal electron donor amine groups in the structure.34,35 The replacement of electron acceptor cyano groups with terminal amine can convert n-type g-C3N4 to p-type g-C3N4. Very recently, Liu et al. prepared p–n homojunction g-C3N4 by treatment of graphitic carbon nitride with NaBH4.32 They reported that the composition on both sides of the interface in such homojunctions gives rise to a continuous band bending, which facilitates charge transfer across the interface and enhances the hydrogen evolution ability. Similarly, Yeh et al. prepared visible light driven nitrogen doped graphene oxide quantum dots exhibiting both n and p-type behaviour for overall water splitting.36 All the abovementioned facts inspired us to construct exfoliated as well as p–n homojunction g-C3N4 in a single step, which should solve many problems hindering the practical application of g-C3N4.
Our group has reported a highly active g-C3N4 with advanced optical, electronic properties in 2013.37 We have also successfully synthesized various g-C3N4 based photocatalysts for hydrogen energy generation.15,38,39 Since bulk g-C3N4 prepared by direct polycondensation from its suitable precursor suffers from low exposed area and poor photocatalytic activity, it is necessary to prepare an efficient g-C3N4 with high optical and electronic features for its better utilization in photocatalysis.
Herein, we developed a novel, one step biomediated synthesis path for the construction of p–n homojunction and exfoliated g-C3N4 from melamine by a simple thermal condensation method using oyster shells. It is shown that g-C3N4 prepared over oyster shells not only exhibits extensive light absorption properties, but also has a well exfoliated sheet like structure and both n- and p-type conductivities in g-C3N4, leading to the creation of a p–n homojunction structure. To the best of our knowledge, this is the first successful one step synthesis of p–n homojunction and exfoliated g-C3N4in situ.
Experimental
Preparation method
The polymeric graphitic C3N4 photocatalysts were individually synthesized from the melamine precursor by the following methods.
(a) Simple thermal condensation (silica crucible).
A calculated quantity of melamine was taken in a covered alumina crucible and was then subjected to calcination at 550 °C for 2 h at a heating rate of 5 °C min−1. The prepared graphitic carbon nitride was simply designated as g-C3N4.
(b) Bio-mediated route (oyster shell).
Two oyster shells with average wt of 7.26 gm each and sample holding capacity of 4 gm (melamine) were cleaned in distilled water and kept in acetone solution overnight. Their surfaces were dried with tissue paper and the concave section of one oyster shell was scratched with a sharp edged tool. For the preparation of g-C3N4, a stoichiometric amount of melamine was kept in the scratched shell while the other completely covered it; then, it was calcined in air using a muffle furnance at 550 °C for 2 h. The above treated product was ground to obtain the particles of g-C3N4. The prepared sample is represented as g-C3N4 (oyster shell), and its synthesis is diagrammatically presented in Scheme 1.
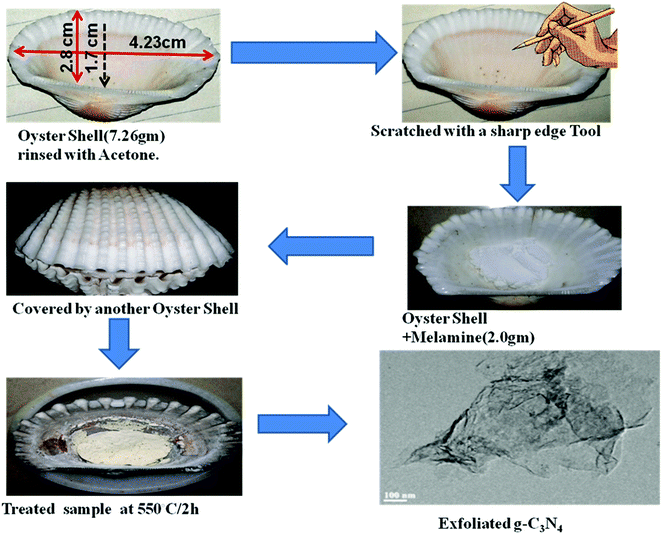 |
| Scheme 1 Synthesis of g-C3N4 over oyster shells. | |
Photocatalytic experiments
The photocatalytic hydrogen production experiment was performed in an inner irradiation type reactor. The reaction was carried out by taking 0.05 g of powder photocatalyst in 50 mL of 10 vol% of triethanol amine solution (TEA solution). The solution was continuously stirred with a magnetic stirrer for proper dispersion in aqueous media and to prohibit the particles from settling at the bottom of the reactor. Before light irradiation, the reactant solution was first evacuated several times by purging with nitrogen to ensure complete removal of air. A 125 W medium pressure Hg visible lamp was used as the light source and 1 mol L−1 of NaNO2 solution was introduced into the water jacket as a UV filter to carry out the reaction beyond 400 nm. The evolved gas was collected by the water displacement technique and analyzed by GC-17A using a 5 Å molecular sieve column with a thermal conductivity detector (TCD).
The photocatalytic degradation of Rhodamine B was carried out in a quartz reactor by taking 0.02 g of prepared g-C3N4 in 20 mL of aqueous Rhodamine B solution under solar light irradiation for 1 h. After the experiment, the residual Rhodamine B concentrations were analyzed periodically by a JASCO 750 UV-vis spectrophotometer at 556 nm.
Analytical characterisation
The product was characterised by powder X-ray diffraction (PXRD), using a Rigaku Miniflex (set at 30 kV and 15 mA) with CuKα radiation (λ = 1.54 Å). The diffraction patterns of the photocatalysts were recorded in the range 10° < 2θ < 60° with a scan rate of 2° min−1. FTIR spectra of the samples were recorded on a Bruker ALPHA FTIR spectrometer. The carbon and nitrogen contents were determined using elemental analysers (TruSpec, CHNS analyser). The DRUV-vis spectra of all the photocatalysts were obtained on a JASCO 750 UV-vis spectrophotometer in the region 200–800 nm, with boric acid as the reference. Transmission electron microscopy (TEM) studies were conducted using a TEM-JEOL-2010 200 kV instrument. The photoluminescence (PL) spectra of the prepared samples were obtained using a JASCO-FP-8300 fluorescence spectrometer. For PL analysis, the samples were excited at 300 nm at room temperature. FESEM images were obtained using a Carl Zeiss, Neon 40 instrument. AFM images of the sample were obtained using a Park NX10 instrument. X-Ray photoelectron spectroscopy (XPS) measurements were carried out using a VG Microtech Multilab ESCA 3000 spectrometer with Mg-Kα as the X-ray source. Fourier transform infrared (FT-IR) spectra of the samples were obtained using a Bruker ALPHA FT-IR spectrometer. TGA analysis was performed using a NETZSCH TG 209F analyser at a scan rate of 5 °C min−1. Electrochemical measurements were conducted using a three electrochemical cell consisting of a platinum wire as the counter electrode, Ag/AgCl electrode as reference electrode and a prepared electrode as the working electrode. The cell was filled with an aqueous solution of 0.1 M Na2SO4. The electrolyte was saturated with nitrogen gas prior to electrochemical measurements. The photoelectrochemical measurements were performed using an Ivum multichannel potentiostat under illumination conditions (λ ≥ 400 nm). Irradiation was performed using a 300 W Xe lamp with a 400 nm cutoff filter.
Results and discussion
XRD
Fig. 1 presents the XRD pattern of g-C3N4 prepared by a simple thermal condensation method and biomediated route. The obtained XRD patterns of both g-C3N4 are well supported by the reported articles for the formation of a stacked graphitic C3N4 layer. The peak at around 2θ = 13.4 is due to the in-plane structural packing framework of tristriazine units and is denoted as the (100) plane, whereas, the strong peak located at 2θ = 27.10 is attributed to the interlayered stacking reflection of conjugated aromatic segments and corresponds to the (002) plane.15,37
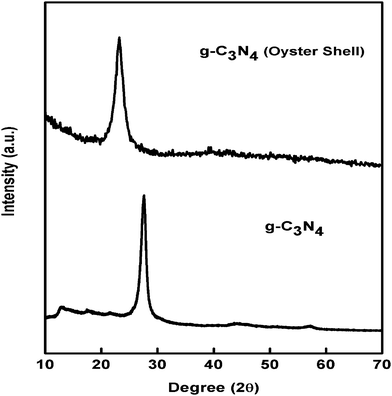 |
| Fig. 1 X-ray diffraction patterns of the g-C3N4 and g-C3N4 (oyster shell). | |
From the XRD pattern, it has been observed that the most intense (002) plane in the case of g-C3N4 prepared by the biomediated route is significantly broadened and also shifted towards a lower angle. The overall intensity of the 002 plane is also minimized. The shifting of the peak towards a lower angle clearly signifies the increase in the interlayer distance between the conjugated aromatic systems in the case of g-C3N4 prepared using oyster shells, compared to g-C3N4 prepared by the traditional method.37 The increase in the interlayer distance resulted in the formation of exfoliated g-C3N4 sheets with higher interlayer distances. This result is further confirmed by TEM analysis, which is discussed in a later section.
Elemental analysis of both samples was performed to identify the C, N atomic ratio. The C, N ratio in the case of g-C3N4 prepared by the thermal condensation method was found to be 0.701, whereas that of the g-C3N4 prepared over oyster shells was observed to be 0.755. The results signify some preferential loss of N atoms during the formation of the g-C3N4 prepared over oyster shells.32
FTIR spectra
The molecular structure of the prepared materials was also confirmed by FTIR spectra. The FT-IR spectra of both the materials are presented in Fig. S1 (ESI†). The absorption band at around 808 and 875 cm−1 are attributed to the vibrational mode of the triazine units.37,40 The peaks observed at 1258–1480 cm−1 are mostly due to typical vibrations of C–N, and the bands at around 1560–1638 cm−1 are mostly due to the vibration of C
N bonds in the aromatic ring. It is very interesting that the signal for –CN is more intense in the case of g-C3N4 (oyster shell), from which it is concluded that the –CN functionalities are quite enhanced. The broad vibrational band at around 3100–3400 cm−1 is due to the presence of uncondensed amine groups and water molecules during the synthesis of the photocatalysts.37 All these characteristic vibrational bands showed the well-formed g-C3N4 prepared via the condensation of melamine over oyster shells.
DRUV-vis spectra
The prepared samples were characterized using a DRUV-vis spectrometer to determine their optical absorption properties. Fig. 2 shows the light absorption behavior of g-C3N4 synthesized by the conventional thermal method and that obtained through the biomediated route. From the optical absorption spectra, it has been observed that g-C3N4 prepared by both methods was able to absorb visible light. The most interesting observation found in the case of g-C3N4 prepared through the bio-mediated route was that the intensity of light absorption was higher than in g-C3N4 prepared by the traditional thermal condensation method. The absorption edge of g-C3N4 (oyster shell) was slightly shifted towards higher wavelength, which is a most important criterion for an active photocatalyst. The unmarked area in the optical absorption spectra shows the extra amount of light absorbed by the g-C3N4 prepared through the bio-mediated route. The higher intensity of light absorption is mostly due to the efficient separation of the interlayer spacing of the conjugated aromatic system. The efficient separation of the interlayer leads to the penetration of more light into the bulk of the semiconductor photocatalysts. Moreover, the higher C/N ratio value in the case of g-C3N4 (oyster shell), as observed from elemental analysis, is also another reason for the efficient absorption of visible light as described by Liu et al.32 The absorbance of a greater amount of light energy played a significant role in the formation of more charge carriers in the semiconductor photocatalysts.
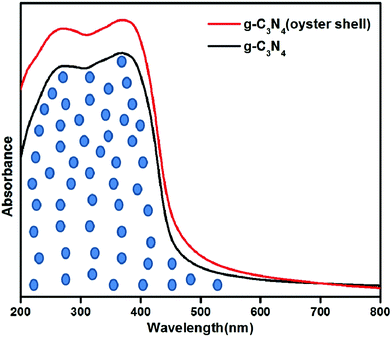 |
| Fig. 2 Optical absorbance spectra of g-C3N4 and g-C3N4 (oyster shell). | |
The optical bandgap energy of the photocatalysts was computed using the following equation:
where
α,
n,
A, and
Eg are the absorption coefficient, light frequency, proportionality constant, and bandgap energy, respectively. The features of the transition in a semiconductor depends on the value of
n;
i.e. n = 1/2 for a direct transition and
n = 2 for an indirect transition.
2,37 In this case, the optical absorption is directly favoured. The bandgap energy of g-C
3N
4 changes from 2.73 eV to 2.69 eV using oyster shells (Fig. S2, ESI
†). The slight decrease in band gap energy significantly favors the photocatalytic activity of g-C
3N
4.
FESEM
The surface morphologies of all the photocatalysts were investigated using FESEM. The FESEM image of all the samples are represented in Fig. 3; the two dimensional layered morphology is clearly observed in both samples. The layered, agglomerate sheet like morphology was observed in the case of g-C3N4 prepared from melamine by the traditional route, whereas in case of the g-C3N4 prepared from melamine over the oyster shell surface, the 2D layers are clearly separated and well visible. The EDX study of g-C3N4 (oyster shell) showed that the sample consists of only carbon and nitrogen, which proves that there is no other contamination in the case of g-C3N4 prepared over oyster shells (Fig. S3, ESI†).
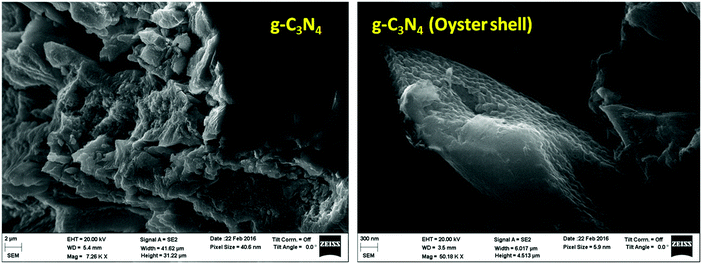 |
| Fig. 3 FESEM images of g-C3N4 and g-C3N4 (oyster shell). | |
Transmission electron microscopy
TEM imaging was carried out to compare the morphological and crystalline character of g-C3N4 prepared by both methods. Fig. 4(a) shows the TEM images of g-C3N4 synthesized by the conventional thermal condensation method and by the thermal condensation over oyster shells. From the TEM micrograph, it can be observed that the g-C3N4 prepared by the conventional thermal condensation method consists of a 2D congregated layered wafer like morphology. The layered structures of the aromatic conjugated planes are mostly aggregated and the 2D layers of the g-C3N4 planes are not clearly visible. However, the one synthesized using oyster shells is well scaled off into horizontal, exfoliated nanosheet like structure as depicted in Fig. 4(a). The thermal condensation of melamine over oyster shells not only helps in the formation of exfoliated sheets but also makes the surface porous. The porosity of the surface can be observed from the TEM micrographs at high magnification (Fig. 4(b)). This porous network is desirable for the foreseeable photocatalytic performance due to efficient mass transfer and the harvesting of more visible light.41
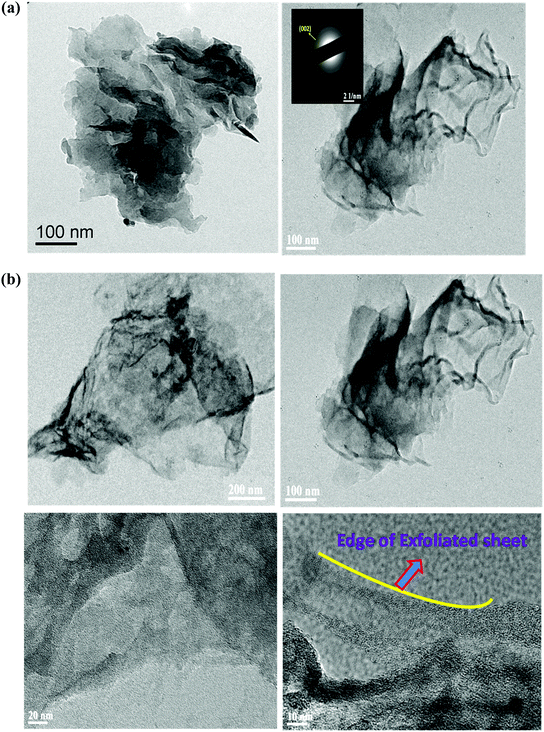 |
| Fig. 4 (a) TEM images of g-C3N4 and g-C3N4 (oyster shell). (b) TEM images of g-C3N4 (oyster shell) at different magnifications. | |
Since the planes of sheets are well disarticulated and distinguished, this mode of preparation can be further explored for tuning the efficiency of various semiconductors towards photocatalytic application. The two-dimensional layer structure of g-C3N4 (oyster shell) is clearly observed by obtaining the TEM images at various magnifications. By varying the magnification, the elongated and exfoliated 2D layered structure with porous morphology of g-C3N4 is clearly observed, as shown in Fig. 4(b). It is worth noting that the exfoliation process does not require any advance techniques or complex apparatus; therefore, the thermal condensation of melamine over oyster shell is one of the easiest, most inexpensive one step processes for preparing well exfoliated g-C3N4 nanosheets without any template, compared to all reports to date (Table S1, ESI†). Table S1 in the ESI† summarizes the studies regarding methods of exfoliation of g-C3N4. The prepared g-C3N4 nanosheet can be easily modified further with various classes of semiconductors.
The crystalline character was also confirmed by the SAED pattern, shown in the inset in the TEM image of g-C3N4 (oyster shell), which revealed bright continuous concentric rings, attributed to diffraction from the 002 planes of graphitic C3N4, consistent with the results obtained from XRD.
AFM
In order to confirm the 2D layered structure of g-C3N4 prepared using oyster shells, the side view of the sample was observed by atomic force microscopy (AFM), which revealed that the obtained g-C3N4 has a rough surface (Fig. S4, ESI†). The AFM image indicated that the surface of the g-C3N4 (oyster shell) is composed of 20 or more layers of g-C3N4 sheets with height of 3 μm.
IV measurement
The photoelectrochemical behaviour of the prepared g-C3N4 and g-C3N4 (oyster shell) was analysed by IV measurement. The IV curves of the prepared photocatalysts in dark as well as light conditions are presented in Fig. 5(a) and (b). From the IV curve, it was observed that g-C3N4 generated photocurrent in the anodic direction with the applied potential, which is the typical behavior of n-type semiconductors.37 Surprising results were observed: the g-C3N4 prepared with oyster shells showed an increment in current density along the cathodic direction with negative bias and anodic current, with positive bias under visible light irradiation, which clearly indicates the co-existence of both p-type and n-type characters in the single material. The IV result of g-C3N4 (oyster shell) suggests that the co-existence of n- and p-type constituents in g-C3N4 (oyster shell) would lead to the formation of p–n type homojunctions in a single material, which facilitates the flow of charge carriers in the opposite direction.32 The formation of homojunctions was further confirmed by Mott–Schottky analysis by studying the respective band edge positions of the n- and p-type constituents in g-C3N4 (oyster shell).
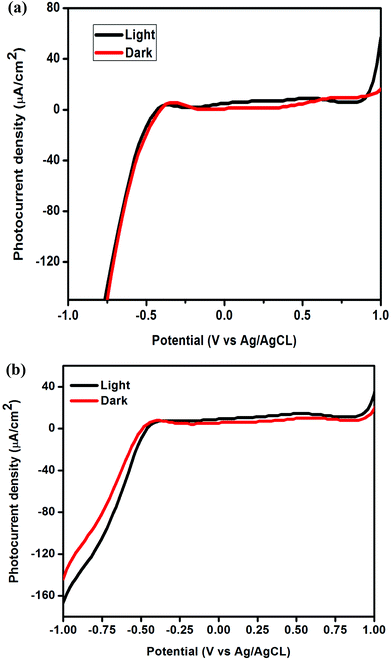 |
| Fig. 5 (a) IV plot of g-C3N4. (b) IV plot of g-C3N4 (oyster shell). | |
EIS analysis
The EIS analysis is used for the investigation of charge transfer resistance of the prepared g-C3N4 and g-C3N4 (oyster shell) photocatalysts. The Nyquist impedance plots (imaginary part, Z′, versus real part, Z′′) for g-C3N4 and g-C3N4 (oyster shell) were analysed at an applied potential of 0.0 V, using 0.5 M Na2SO4 solutions (Fig. 6). The plots are composed of small semicircles in the high frequency region and a line in the lower frequency region. The large semicircle observed for the neat g-C3N4 is indicative of high interfacial charge-transfer resistance, relating to the poor electrical conductivity along the electron pathway in the electrode. The small semicircle observed for g-C3N4 (oyster shell), mostly due to the lower interfacial charge transfer resistance, signifies the higher electrical conductivity of the materials.42,43 The high electrical conductivity supports the easy flow of electrons at the electrode–electrolyte interface. The impedances at high frequency reflect the equivalent series resistance in the electrode/electrolyte system, which is contributed from both the electrolyte resistance and electronic resistance of the photoelectrodes. From the plot, it was confirmed that g-C3N4 (oyster shell) has the lower equivalent series resistance because of its good electrical conductivity, as reported in earlier studies.44
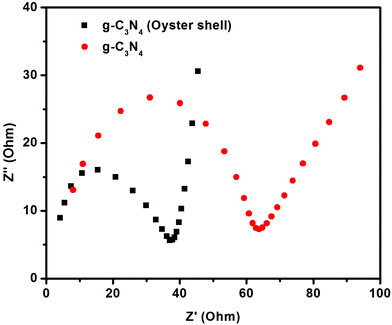 |
| Fig. 6 EIS of g-C3N4 and g-C3N4 (oyster shell). | |
A comparison of the figures for both prepared electrodes, i.e., g-C3N4 and g-C3N4 (oyster shell) indicates that there is a significant change in charge-transfer resistance. From the diameter of the semicircle, the charge-transfer resistances of g-C3N4 and g-C3N4 (oyster shell) electrodes were found to be 60 Ω and 40 Ω, respectively. The low electrical conductivity of g-C3N4 results in significant charge-transfer resistance for g-C3N4. In contrast, g-C3N4 (oyster shell) shows a smaller charge-transfer resistance due to its good electrical conductivity. The minimum charge-transfer resistance value of g-C3N4 (oyster shell) is proof of the higher photocatalytic ability of the photocatalysts. Furthermore, the reduction in the charge-transfer resistance values of g-C3N4 (oyster shell) electrodes could be the prime reason for the enhancement in the rate of charge transfer of the ions at the g-C3N4 (oyster shell) photoelectrode electrolyte interface. Moreover, the straight line of the Nyquist plot at high frequency is related to the Warburg resistance resulting from the frequency dependence of ion diffusion/transport in the electrolyte.43,45 The large Warburg region of g-C3N4 electrodes shows the greater variation in ion diffusion path lengths and increased obstruction of ion movement.44 g-C3N4 (oyster shell) exhibited a short diffusion path length for ions in the electrolyte, which could be seen from the low resistance of the capacitive part of the Nyquist plot.43,44
MS analysis
Mott–Schottky analysis was performed in order to identify the type of semiconductor and band edge potential, which ultimately helps in designing the photocatalytic mechanism pathway of the samples. As shown in Fig. 7(a), the straight line with positive slope drawn to the potential axis in the case of g-C3N4 prepared through the traditional route suggests its intrinsic n-type character. From the intercept on the potential axis, the conduction band edge (ECB) of n-type g-C3N4 was found at −1.10 V. Interestingly, for g-C3N4 (oyster shell) the two straight lines were extrapolated with positive and negative slopes located in different potential regions, which signifies the co-existence of both n- and p-type features in the same material (Fig. 7(b)).32,46 The positive slope found at −1.16 V shows the typical characteristics of the conduction band edge of n-type g-C3N4 and the negative slope located at +0.25 V shows the corresponding valance band edge (EVB) of the p-type g-C3N4. It can be claimed that the co-existence of n- and p-type domains in a single g-C3N4 (oyster shell) indicates the formation of p–n type homojunctions, in which the Fermi-level (EF) alignment of the p- and n-type g-C3N4 occurs, thereby creating a built-in field at the interface. The built in electric field enables the photogenerated electrons and holes to migrate in opposite directions, which effectively inhibits the recombination of charge carriers. This process results in significant improvement in photocatalytic activity.32,47
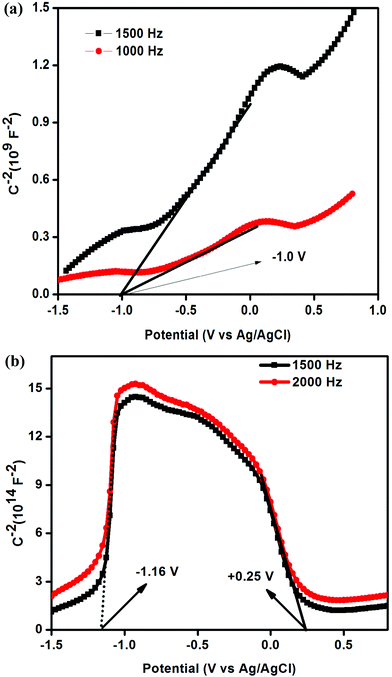 |
| Fig. 7 (a) Mott–Schottky plot of g-C3N4. (b) Mott–Schottky plot of g-C3N4 (oyster shell). | |
To the best of our knowledge, this is the first report where the exfoliated and p–n homojunction g-C3N4 photocatalysts are prepared in a single step through a bio-mediated route.
XPS spectra
Fig. S4 and S5 (ESI†) show the XPS spectra of C 1s, and N 1s in g-C3N4 andg-C3N4 (oyster shell). The spectrum of C 1s in both materials represents a broad peak in the region of 284.9 eV and another at 288 and 288.3 eV. The peak located at 284.9 eV is assigned to the C–C bond, in contrast to the XPS peaks at 288 to 288.3 eV, due to the carbon atom bonded to nitrogen atom.37,38 The N 1s peak in both materials is broad and deconvoluted into three different peaks. In the case of g-C3N4, the deconvoluted peaks were found at 398.6, 399.3 and 400.7 eV. The broad peak of N 1s found at 398.6 was deconvoluted into three peaks with binding energy values of 398.6 eV, 399.3 eV and 400.7 eV, respectively.37 The peak at 397.7 eV is due to C–N–C bonding, while the peaks at 398.6 eV and 400.7 eV are ascribed to the tertiary nitrogen N–(C)3 and N–H. In contrast, the deconvoluted N 1s peaks of g-C3N4 (oyster shell) are found at 397.7, 398.7 and 400 eV. The abovementioned binding energy values indicate that in the case of g-C3N4, (oyster shell) more nitrogen atoms are directly attached to carbon. From the XPS spectra, it is observed that in the case of g-C3N4 prepared over oyster shells, the peak-area ratio of two-coordinated nitrogen to three-coordinated N shows a decreasing trend, suggesting that the two-coordinated N atoms are preferentially lost and three coordinate nitrogen atoms are formed during the course of formation of the material.32
Mechanism for the formation of exfoliated and p–n homojunction g-C3N4
The surface of oyster shells mainly consists of CaCO3 with some inorganic minerals and organic moieties.48 During the synthesis of g-C3N4 over oyster shells, the thermal condensation process leads to the decomposition of moisture and organic content (2.5%) attached to the surface of the oyster shell. The TGA data of the oyster shell is provided in the ESI† (Fig. S7). The decomposition of organic content and moisture results in the evolution of some gaseous carbon species as well as water vapor. In bulk g-C3N4, the energy of the van der Waals forces existing between the bonded C3N4 layers is equivalent to 70 mJ m−2, and in another study, Zhang et al. theoretically calculated the surface energy of g-C3N4 to be about 115 mJ m−2. Therefore, the chemistry behind the preparation of exfoliated g-C3N4 dictates that the van der Waals forces as well as the surface energy of the C3N4 network must be minimized so that we can prepare well exfoliated g-C3N4. Yang et al. reported that the bulk g-C3N4 structure can be exfoliated into a layered nanosheet structure by the liquid-exfoliation process.29 It has been reported that the surface energy of g-C3N4 bulk must match that of the solvent to minimize the enthalpy of the mixture.29,31 In our present study, we have prepared exfoliated g-C3N4 by a simple thermal condensation process of melamine over oyster shells. Our claim is that the thermal condensation process over oyster shell leads to the decomposition of organic contents and moisture. The decomposition of organic contents and moisture can result in the evolution of some gaseous carbon species and water vapor. The slow and uniform evolution of gases from the surface of the oyster shell mostly decreases the van der Waals forces as well as the surface energy of the C3N4 network in graphitic carbon nitride layers. This decrement of the van der Waals force and the surface energy of the g-C3N4 layers is the prime factor for the formation of exfoliated sheets. The slow and uniform liberation of gaseous carbon species also helps in making the surface porous, as is evident from the TEM micrographs. To support our observation, we also carried out another experiment, i.e., we prepared g-C3N4 over a calcined oyster shell; we did not observe the exfoliated sheet of g-C3N4 (Fig. S8, ESI†). Therefore, we concluded that the slow evolution of gases from the surface of oyster shells is mainly helpful for nanosheet formation. It was noted that the carbon nitride framework was not altered in the presence of the gas template, as only 2.5% of the oyster shell was decomposed during the course of the reaction. The TGA of g-C3N4 (oyster shell) is provided in Fig. S9 of the ESI.† It is well known that carbon monoxide is a very good reductant and is used as a reducing agent in various reactions. During the thermal condensation of melamine over oyster shells, the evolution of carbon monoxide reduces the terminal uncondensed amine groups and evolves ammonia. The reduction of uncondensed amine groups introduces nitrogen defects into the system. The direct reaction of gaseous carbon species with g-C3N4 is the prime reason for the formation of defects in g-C3N4. It is well known that g-C3N4 is a typical n-type material because it contains several NH/NH2 groups as electron donors in the structure.34 As reported by Liu et al., replacing NH/NH2 electron-donor groups on the g-C3N4 sheet edge with some electron acceptor –CN functional groups can readily transform g-C3N4 into an n–p homojunction type semiconductor.32 In this investigation, the interaction of gaseous carbon species with the uncondensed amine group attached to the edge of g-C3N4 introduces some electron acceptor –CN groups, which help in the formation of an in situ p–n homojunction system. The introduction of –CN groups was also proven by the XPS spectroscopy. From the XPS spectra, it is observed that in case of g-C3N4 prepared over oyster shells, the peak-area ratio of two-coordinated nitrogen to three-coordinated N shows a decreasing trend, suggesting that the two-coordinated N atoms were preferentially lost, and three co-ordinate nitrogens were formed during the course of formation of the material. Moreover, from the elemental analysis, it has been confirmed that the C, N ratio in the case of g-C3N4 prepared over the oyster shell was observed to be 0.755, which is higher than that of g-C3N4 prepared by the thermal condensation method, i.e., 0.701. The higher value of the C, N ratio showed that there is some loss of nitrogen atoms during the synthesis of the material. This is also possibly due to the reduction of uncondensed amine groups to electron acceptor –CN groups.32 From FTIR spectra, it is also observed that the band of the –CN group is enhanced in the case of g-C3N4 (oyster shell), indicating the presence of more –CN groups than that of g-C3N4 prepared by the thermal condensation method.
PL spectra
The photoluminescence spectra of g-C3N4 and g-C3N4 (oyster shell) are shown in Fig. 8. The PL spectra are useful to disclose the migration, transfer and electron–hole recombination in semiconducting materials.2,37,49 From the PL spectra, it is observed that both samples show only one type of luminescence behavior. The emission peaks of both samples are broad and centered at around 450 nm, which is similar to our previous report.37 This strong luminescence peak is due to the band–band PL phenomenon, which is approximately equal to the band gap energy of C3N4.37 This emission peak is ascribed to the direct recombination of excitons through an exciton–exciton collision process, which plays a pivotal role in the recombination process of charge carriers.
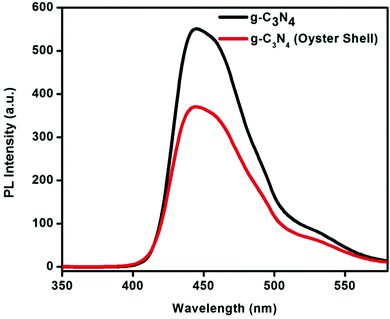 |
| Fig. 8 PL spectra of g-C3N4 and g-C3N4 (oyster shell). | |
A comparative study of the luminescence peaks of both samples indicates that C3N4 prepared using oyster shells shows minimum PL intensity. Therefore, C3N4 prepared by the biomediated route is very effective for the separation of charge carriers. Due to the strong inhibition of charge carrier recombination in g-C3N4 (oyster shell), there is higher photocatalytic activity, compared to C3N4 prepared by the traditional route. Due to strong recombination of charge carriers, the concentration of free charge carriers is high during the photocatalytic process, which is the prime reason for higher photocatalytic activity.
Time-resolved photoluminescence spectra
In order to confirm the stronger recombination of charge carriers in g-C3N4 (oyster shell), compared to g-C3N4 prepared by the traditional route, we performed time-resolved PL experiments.38Fig. 9 shows the time-resolved PL spectra of g-C3N4 and g-C3N4 (oyster shell). To determine the lifetimes of the charge carriers, the resulting PL decay emission plots were well fitted to the triexponential functions using eqn (1). | Fit = A1·exp(−t/T1) + A2·exp(−t/T2) + A3·exp(−t/T3) | (1) |
After fitting the curves to a tri-exponential function, the decay components (τ1, τ2 and τ3) and the relative amplitudes of the decay species (A1, A2, A3) were calculated. The corresponding values are presented in Table 1.
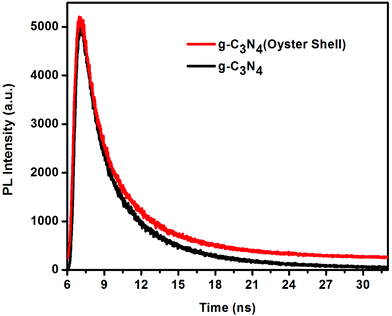 |
| Fig. 9 TRPL spectra of g-C3N4 and g-C3N4 (oyster shell). | |
Table 1 Summary of the average lifetime of charge carriers
Sample |
τ
1 (ns) (A1%) |
τ
2 (ns) (A2%) |
τ
3 (ns) (A3%) |
t
av (ns) |
g-C3N4 |
0.728 (64.5) |
2.9 (33) |
10.4 (2.5) |
3.45 |
g-C3N4 (oyster shell) |
0.53 (47) |
3.74 (41) |
9.55 (12) |
5.74 |
The average lifetime τ was calculated using the equation below:44
| 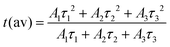 | (2) |
The average lifetime of the prepared g-C
3N
4 was calculated for comparison of the emission decay behavior of all the materials and to know the life time of the excited charge carriers.
It is clearly observed from the calculated data (Table 1) that the average life time of the excited charge carriers in the case of g-C3N4 (oyster shell) is 5.74 ns, whereas g-C3N4 prepared by the traditional route exhibited a lifetime of 3.45 ns. Therefore, the life time of the excited charge carriers is greater in g-C3N4 (oyster shell) than in g-C3N4. The longer life time of the charge carriers in the case of g-C3N4 (oyster shell) is mostly due to the exfoliated structure of g-C3N4. Due to the longer life time of the excited charge carriers in g-C3N4 (oyster shell), a greater number of free charge carriers is available for the initiation of photocatalytic reaction.38
Photocatalytic activity
To study the photocatalytic behavior of all the samples, the activities were tested for hydrogen evolution under visible light irradiation (λ ≥ 400 nm) and the degradation of RhB under solar light irradiation. For photocatalytic hydrogen evolution, all the experiments were carried out by taking 50 mL of 10 vol% TEA solution and 0.05 g of photocatalysts. From the reference experiments, it has been confirmed that no gas was produced when the reactor was illuminated in the absence of photocatalyst, or in the presence of the photocatalyst without light irradiation.
From the hydrogen evolution experiment (Fig. 10), it has been confirmed that both the prepared carbon nitride samples were able to produce hydrogen under visible light in the absence of any co-catalysts. The hydrogen evolution activities of both types of g-C3N4 as a function of time are shown in Fig. 10. In 3 h of photocatalytic experiment, g-C3N4 prepared from melamine was able to produce 18 μmol of hydrogen gas under visible light irradiation, whereas g-C3N4 (oyster shell) was able to generate 88 μmol of hydrogen gas in the same duration. In order to know the stability of the g-C3N4 (oyster shell) photocatalyst, repeated experiments were carried out with evacuation of the photocatalytic system in a time period of 3 h for four continuous cycles (Fig. 11). From the stability study, it was observed that the material was able to produce a steady rate of hydrogen, i.e., 87 μmol h−1.
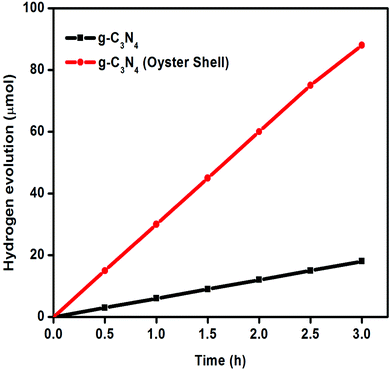 |
| Fig. 10 Photocatalytic hydrogen generation study over g-C3N4 and g-C3N4 (oyster shell) under visible light irradiation (λ ≥ 400 nm). | |
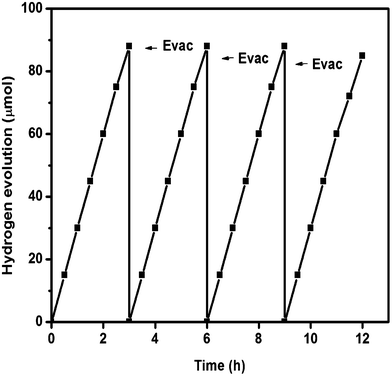 |
| Fig. 11 Stability study of g-C3N4 (oyster shell) for hydrogen generation under visible light irradiation (λ ≥ 400 nm). | |
The apparent conversion efficiency (ACE) was calculated as follows:
The intensity of incident light energy was found to be 120 mW; therefore, the ACE (%) was calculated to be 5.71%.
As reported earlier, g-C3N4 has suitable thermodynamic potential for the water splitting reaction, i.e., the conduction and valence band edge potentials of g-C3N4 are located at −1.12 and 1.57 eV.5 The characterisation results for both prepared materials showed that g-C3N4 prepared over oyster shells has some interesting properties for its best photocatalytic activity. In order to prove the exceptional activity of g-C3N4, we tested the dye degradation activity of both the photocatalysts by taking Rhodamine B as a model dye. The concentration vs. percentage of degradation of Rhodamine B solution is shown in Fig. 12(a) and (b). It was observed that both were able to degrade Rhodamine B solution. In comparison to g-C3N4 prepared through the traditional route, the sample prepared over oyster shell is more efficient towards the degradation of organic dye. g-C3N4 (oyster shell) was able to degrade 100% up to 150 ppm RhB solution, whereas g-C3N4 degraded 100% up to 100 ppm solution. With 200 ppm Rhodamine B solution, g-C3N4 (oyster shell) was able to degrade 90.2%, whereas g-C3N4 was able to degrade only 58%. This proves that g-C3N4 (oyster shell) not only shows good activity towards hydrogen production, but also towards the dye degradation reaction.
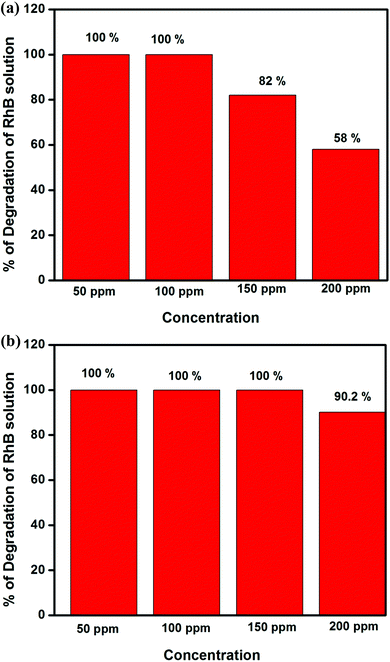 |
| Fig. 12 (a) Degradation of Rhodamine B as a function of concentration over g-C3N4 (time = 1 h). (b) Degradation of Rhodamine B as a function of concentration over g-C3N4 (oyster shell) (time = 1 h). | |
First, from the XRD results, it was observed that the most intense (002) plane in the case of g-C3N4 prepared by the biomediated route was significantly broadened and also shifted toward lower angle, which clearly signifies the increase in the interlayer distance between the conjugated aromatic system in the case of g-C3N4 prepared using oyster shell, compared to g-C3N4 prepared by the traditional method.37 The increase in the interlayer distance resulted in the formation of exfoliated g-C3N4 sheets with greater interlayer distance. The increased interlayer distance between the C3N4 layers can accommodate sufficient water molecules and drive the water reduction reaction by the photocatalytic process. This mechanism is well explained by our previous study and also by other groups. Second, the most interesting observation found in the case of g-C3N4 prepared through the bio-mediated route is that the intensity of light absorption is higher than that of the g-C3N4 prepared by the traditional thermal condensation method. The absorption edge of the g-C3N4 (oyster shell) is slightly shifted towards higher angle, which is the most important criterion for an active photocatalyst, clearly described in the UV-vis DRS section. The photocatalytic activity of a photocatalyst is directly proportional to the amount of light absorbed during the photocatalysis process. As shown in UV-vis DRS, g-C3N4 prepared over the oyster shells was able to absorb an extra amount of light compared to g-C3N4 prepared from melamine by the tradition thermal condensation method. The higher intensity of light absorption was mostly due to the efficient separation of the interlayer spacing of the conjugated aromatic system. The efficient separation of the interlayer leads to the penetration of more light in the bulk of the semiconductor photocatalyst, which plays a significant role in the formation of greater numbers of charge carriers in the semiconductor photocatalysts and enhanced the photocatalytic activity.
Third, TEM results confirmed that the two dimensional layer in the case of g-C3N4 prepared over oyster shells is clearly separated and also exfoliated. These exfoliated sheets can provide enough surfaces for photocatalytic reaction. From the earlier report, it was proven that the photocatalysts with layered morphology are very effective for hydrogen evolution reaction.50,51 In the case of g-C3N4 (oyster shell), the layers of the C3N4 sheets are well separated and elongated and can easily accommodate enough TEA solutions, which precede the reduction reaction.
The formation of a homojunction in the case of g-C3N4 (oyster shell), as confirmed by the Mott–Schottky analysis, is the most important criterion for enhanced photocatalytic activity. The formation of the p–n homojunction creates an electric field at the interface. After the irradiation of light, the electrons and holes are generated at both p and n type g-C3N4. The electrons tend to migrate from the conduction band of the p-type g-C3N4 to the n-type g-C3N4, whereas the holes migrate from the valance band of n-type g-C3N4 to p-type g-C3N4; this enables the effective inhibition of the recombination of charge carriers. The retardation of the recombination of charge carriers was also confirmed by EIS and TRPL spectra. This process results in the significant use of charge carriers for driving the photocatalytic reaction. Therefore, the formation of the homojunction played an important role in the enhancement of photocatalytic activity. The mechanism of hydrogen evolution is schematically presented in Scheme 2.
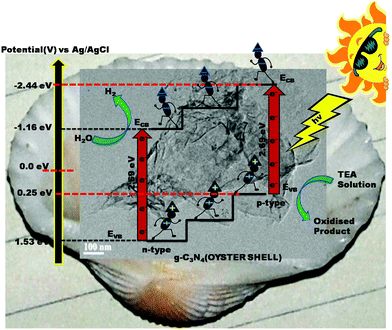 |
| Scheme 2 Schematic of the mechanism of hydrogen production over g-C3N4 (oyster shell). | |
Conclusion
In summary, we prepared exfoliated 2D sheets and p–n homojunction g-C3N4 with excellent optical properties, electronic properties and photocatalytic activity by a simple biomediated route, which presents high efficiency towards visible light driven photocatalytic activity. The excellent performance benefits from the unique structural advantages, i.e., exfoliated sheets, electron transfer, light harvesting and accessible reaction sites are further confirmed by various characterization techniques like XRD, DRUV-vis spectra, TEM, FESEM, AFM, PL spectra, TRPL spectra, XPS and electrochemical studies, respectively. The unique photocatalytic activity of g-C3N4 (oyster shell) is in good agreement with EIS analysis, PL study, in situ homojunction formation and ordered morphology with good separation of two dimensional layers of C3N4 sheets. The slow and uniform evolution of gaseous carbon species from the decomposition of oyster shells not only helps in the formation of exfoliated sheets, but also introduces some electron acceptor cyano (–CN) groups, which drives the formation of the p–n homojunction. Further, we believe that this novel preparation method of g-C3N4 may provide a new pathway for the development and modification of g-C3N4 based materials. In addition to the use as photocatalyst, this new type of synthesis method can be envisaged for application in the fields of electrocatalysis, optoelectronic devices, battery, fuel cells applications, etc.
Acknowledgements
The authors are very much thankful to the SOA University, management for their support and encouragement. Dr P. C. Sahoo, R&D officer, IOCL, India is greatly acknowledged for the valuable discussion regarding the study. We are obliged to Prof. P. V. Satyam, IOP, Bhubaneswar for the support of TEM and FESEM analysis. SERB, Government of India is greatly acknowledged for the support of first track young scientist project (sanction No. YSS/2015/001949).
References
- X.-L. Zheng, C.-T. Dinh, F. P. Garcia de Arquer, B. Zhang, M. Liu, O. Voznyy, Y.-Y. Li, G. Knight, S. Hoogland, Z.-H. Lu, X.-W. Du and E. H. Sargent, Small, 2016, 12, 3181–3188 CrossRef CAS PubMed.
- S. Martha, D. K. Padhi and K. M. Parida, ChemSusChem, 2014, 7, 585–597 CrossRef CAS PubMed.
- A. Molla, M. Sahu and S. Hussain, Sci. Rep., 2016, 6, 26034 CrossRef CAS PubMed.
- S. Patnaik, S. Martha and K. M. Parida, RSC Adv., 2016, 6, 46929–46951 RSC.
- X. C. Wang, K. Maeda, A. Thomas, K. Takanabe, G. Xin, J. M. Carlsson, K. Domen and M. Antonietti, Nat. Mater., 2008, 8, 76–80 CrossRef PubMed.
- Y. Zheng, J. Liu, J. Liang, M. Jaroniec and S. Z. Qiao, Energy Environ. Sci., 2012, 5, 6717–6731 CAS.
- J. Zhang, G. Zhang, X. Chen, S. Lin, L. Mçhlmann, G. Dołęga, G. Lipner, M. Antonietti, S. Blechert and X. Wang, Angew. Chem., Int. Ed., 2012, 51, 3183–3187 CrossRef CAS PubMed.
- J. Zhang, M. Zhang, R. Q. Sun and X. Wang, Angew. Chem., Int. Ed., 2012, 51, 10145–10149 CrossRef CAS PubMed.
- Y. Wang, X. Wang and M. Antonietti, Angew. Chem., Int. Ed., 2012, 51, 68–89 CrossRef CAS PubMed.
- S. W. Cao, J. X. Low, J. G. Yu and M. Jaroniec, Adv. Mater., 2015, 27, 2150–2176 CrossRef CAS PubMed.
- Q. Han, B. Wang, J. Gao, Z. Cheng, Y. Zhao, Z. Zhang and L. Qu, ACS Nano, 2016, 10, 2745–2751 CrossRef CAS PubMed.
- C. Liu, Y. Zhang, F. Dong, A. H. Reshak, L. Ye, N. Pinna and H. Huang, Appl. Catal., B, 2017, 203, 465–474 CrossRef CAS.
- N. Tian, H. Huang, C. Liu, F. Dong, T. Zhang, X. Du and Y. Zhang, J. Mater. Chem. A, 2015, 3, 17120–17129 CAS.
- C. Liu, H. Huang, X. Du, T. Zhang, N. Tian, Y. Guo and Y. Zhang, J. Phys. Chem. C, 2015, 119, 17156–17165 CAS.
- S. Samanta, S. Martha and K. M. Parida, ChemCatChem, 2014, 6, 1453–1462 CAS.
- Y. J. Zhang, T. Mori, J. H. Ye and M. Antonietti, J. Am. Chem. Soc., 2010, 132, 6294–6295 CrossRef CAS PubMed.
- J. N. Coleman, M. Lotya, A. O’Neill, S. D. Bergin, P. J. King, U. Khan, K. Young, A. Gaucher, S. De, R. J. Smith, I. V. Shvets, S. K. Arora, G. Stanton, H. Y. Kim, K. Lee, G. T. Kim, G. S. Duesberg, T. Hallam, J. J. Boland, J. J. Wang, J. F. Donegan, J. C. Grunlan, G. Moriarty, A. Shmeliov, R. J. Nicholls, J. M. Perkins, E. M. Grieveson, K. Theuwissen, D. W. McComb, P. D. Nellist and V. Nicolosi, Science, 2011, 331, 568–571 CrossRef CAS PubMed.
- S. B. Yang, X. L. Feng, L. Wang, K. Tang, J. Maier and K. Müllen, Angew. Chem., Int. Ed., 2010, 49, 4795–4799 CrossRef CAS PubMed.
- F. Schedin, A. K. Geim, S. V. Morozov, E. W. Hill, P. Blake, M. I. Katsnelson and K. S. Novoselov, Nat. Mater., 2007, 6, 652–655 CrossRef CAS PubMed.
- K. S. Novoselov, Z. Jiang, Y. Zhang, S. V. Morozov, H. L. Stormer, U. Zeitler, J. C. Maan, G. S. Boebinger, P. Kim and A. K. Geim, Science, 2007, 315, 1379 CrossRef CAS PubMed.
- M. Choi, K. Na, J. Kim, Y. Sakamoto, O. Terasaki and R. Ryoo, Nature, 2009, 461, 246–250 CrossRef CAS PubMed.
- Y. W. Zhu, S. Murali, M. D. Stoller, K. J. Ganesh, W. W. Cai, P. J. Ferreira, A. Pirkle, R. M. Wallace, K. A. Cychosz, M. Thommes, D. Su, E. A. Stach and R. S. Ruoff, Science, 2011, 332, 1537–1541 CrossRef CAS PubMed.
- K. S. Novoselov, A. K. Geim, S. V. Morozov, D. Jiang, Y. Zhang, S. V. Dubonos, I. V. Grigorieva and A. A. Firsov, Science, 2004, 306, 666–669 CrossRef CAS PubMed.
- J. Zhang, Y. Chen and X. Wang, Energy Environ. Sci., 2015, 8, 3092–3108 CAS.
- Y. Zheng, L. Lin, B. Wang and X. Wang, Angew. Chem., Int. Ed., 2015, 54, 12868–12884 CrossRef CAS PubMed.
- X. Du, I. Skachko, A. Barker and E. Y. Andrei, Nat. Nanotechnol., 2008, 3, 491–495 CrossRef CAS PubMed.
- X. L. Li, X. R. Wang, L. Zhang, S. W. Lee and H. J. Dai, Science, 2008, 319, 1229–1232 CrossRef CAS PubMed.
- P. Niu, L. Zhang, G. Liu and H.-M. Cheng, Adv. Funct. Mater., 2012, 22, 4763–4770 CrossRef CAS.
- A. Thomas, A. Fischer, F. Goettmann, M. Antonietti, J. O. Muller, R. Schlogl and J. M. Carlsson, J. Mater. Chem., 2008, 18, 4893–4908 RSC.
- S. Yang, Y. Gong, J. Zhang, L. Zhan, L. Ma, Z. Fang, R. Vajtai, X. Wang and P. M. Ajayan, Adv. Mater., 2013, 25, 2452–2456 CrossRef CAS PubMed.
- X. Zhang, X. Xie, H. Wang, J. Zhang, B. Pan and Y. Xie, J. Am. Chem. Soc., 2013, 135, 18–21 CrossRef CAS PubMed.
- G. Liu, G. Zhao, W. Zhou, Y. Liu, H. Pang, H. Zhang, D. Hao, X. Meng, P. Li, T. Kako and J. Ye, Adv. Funct. Mater., 2016, 26, 6822–6829 CrossRef CAS.
- Y. Qu and X. Duan, Chem. Soc. Rev., 2013, 42, 2568 RSC.
- H. Li, Y. Zhou, W. Tu, J. Ye and Z. Zou, Adv. Funct. Mater., 2015, 25, 998 CrossRef CAS.
- J. Zhang, X. Chen, K. Takanabe, K. Maeda, D. K. Omen, J. D. Epping and X. Wang, Angew. Chem., Int. Ed., 2010, 49, 441–444 CrossRef CAS PubMed.
- T. F. Yeh, C.-Y. Teng, S.-J. Chen and H. Teng, Adv. Mater., 2014, 26, 3297 CrossRef CAS PubMed.
- S. Martha, A. Nashim and K. M. Parida, J. Mater. Chem. A, 2013, 1, 7816–7824 CAS.
- S. Nayak, L. Mohapatra and K. M. Parida, J. Mater. Chem. A, 2015, 3, 18622–18635 CAS.
- S. Pany and K. M. Parida, Phys. Chem. Chem. Phys., 2015, 17, 8070–8077 RSC.
- L. Ge, C. Han and J. Liu, J. Mater. Chem., 2012, 22, 11843–11850 RSC.
- J. R. Ran, T. Y. Ma, G. P. Gao, X. W. Du and S. Z. Qiao, Energy Environ. Sci., 2015, 8, 3708–3717 CAS.
- J. Zhang, J. Jiang and X. S. Zhao, J. Phys. Chem. C, 2011, 115, 6448–6454 CAS.
- Z. Gao, J. Wang, Z. Li, W. Yang, B. Wang, M. Hou, Y. He, Q. Liu, T. Mann, P. Yang, M. Zhang and L. Liu, Chem. Mater., 2011, 23, 3509–3516 CrossRef CAS.
- A. S. Dezfuli, M. R. Ganjali, H. R. Naderi and P. Norouzi, RSC Adv., 2012, 5, 46050–46058 RSC.
- M. Pasta, F. La Mantia, L. Hu, H. D. Deshazer and Y. Cui, Nano Res., 2010, 3, 452–458 CrossRef CAS.
- T. F. Yeh, C.-Y. Teng, S.-J. Chen and H. Teng, Adv. Mater., 2014, 26, 3297 CrossRef CAS PubMed.
- H. Li, Y. Zhou, W. Tu, J. Yea and Z. Zou, Adv. Funct. Mater., 2015, 25, 998 CrossRef CAS.
- G. L. Yoon, B.-T. Kim, B.-O. Kim and S.-H. Han, Waste Manage., 2003, 23, 825–834 CrossRef CAS PubMed.
- S. Martha, K. H. Reddy and K. M. Parida, J. Mater. Chem. A, 2014, 2, 362–3631 Search PubMed.
- T. Takata, Y. Furumi, K. Shinohara, A. Tanaka, M. Hara, J. N. Kondo and K. Domen, Chem. Mater., 1997, 9, 1063–1064 CrossRef CAS.
- S. Ikeda, M. Hara, J. N. Kondo, K. Domen, H. Takahashi, T. Okubo and M. Kakihana, Chem. Mater., 1998, 10, 72–77 CrossRef CAS.
Footnotes |
† Electronic supplementary information (ESI) available. See DOI: 10.1039/c7qm00055c |
‡ S. Martha and S. Mansingh contributed equally to this work. |
|
This journal is © the Partner Organisations 2017 |