DOI:
10.1039/C7QM00008A
(Research Article)
Mater. Chem. Front., 2017,
1, 1406-1412
Red-emitting AIEgen for luminescent solar concentrators†
Received
10th January 2017
, Accepted 22nd February 2017
First published on 24th February 2017
Abstract
This study reports for the first time the use of a red-emitting AIEgen, i.e. TPE-AC, for the realization of efficient luminescent solar concentrators (LSCs) based on poly(methyl methacrylate) (PMMA) and polycarbonate (PC) thin films (25 ± 5 μm). TPE-AC is an AIEgen with D–A features that absorbs visible light in the range between 400 and 550 nm and emits fluorescence peaked at 600–620 nm, with a maximum quantum yield (QY) of 50% when dispersed (0.1–1.5 wt%) in PMMA and PC matrices. QY and lifetime investigations demonstrated that fluorescence quenching occurred with varying the TPE-AC concentration, even if the optical features were still significant even at the highest fluorophore content. Study of the LSCs’ performances yielded worthy optical efficiencies of 6.7% for the TPE-AC/PC systems due to their superior light harvesting features and the compatibility of the AIEgen within the PC matrix.
Introduction
Sunlight concentration is a promising path to cost-effective photovoltaic (PV) systems.1 Compared to classical optical concentrators, luminescent solar concentrators (LSCs) show several advantages: low weight, high theoretical concentration factors, ability to work well with diffuse light and they do not require sun tracking or cooling apparatus.2,3 Today, LSC-PV systems have received great attention due to the demand from the building-integrated PV (BIPV) market.2,4,5 Furthermore, the BIPV market is set to steadily increase, and is also promoted by the European Energy Performance of Buildings Directive 2010/31/EU, which states that each new building should be made “nearly zero energy” from 2020 onwards.6 LSCs are slabs of transparent commodity plastics doped with high-quantum-yield fluorophores.3 Here, as the refractive index of the host is higher than the environment, it traps a fraction of the emitted photons by means of total internal reflection. Photons are then collected at the edges of the LSC to produce electric power by means of PV cells. Red-emitting fluorophores are generally preferred since their fluorescence matches well with the Si-based PV cells band gap.7–9 However, LSCs are plagued by unfavourable processes that hinder their ability to deliver light to PV cells, mainly fluorescence quenching due to dye aggregation.3,10 Indeed, red-emitting organic fluorophores strongly quench their own fluorescence in condensed phases, thus strongly limiting the generation of electric current by the PV cell.11 Surprisingly, aggregation-induced emission fluorophores (AIEgens) as dopants for LSCs have been recently reported as proof-of-principles by Ghiggino et al.12,13 AIEgens are non-emissive when dissolved in good solvents, but become highly luminescent in poor solvents or in the solid state.14–16 A prototypical AIE is tetraphenylethene (TPE), whose central olefin stator is surrounded by four aromatic rotors. TPE fluoresces intensely when aggregated via the mechanism of the restriction of intramolecular rotation (RIR); whereby molecular motions, which could dissipate excited state energy via non-radiative pathways, become blocked, driving the molecule to decay radiatively.17 Such a feature enables AIEgens to successfully enhance a wide array of high-tech applications, such as chemo-(bio)sensors and solid-state emitters with different emission ranges.
In this regards, we have selected a recently developed red-emitting AIEgen, abbreviated as TPE-AC (Fig. 1), based on the TPE stator and efficiently decorated with dimethylamine and malononitrile as the electron donor (D) and the electron acceptor (A) moieties, respectively. Notably, due to the stronger D–A interaction, TPE-AC emits intensively in the aggregated state close to the near infrared portion of the solar spectrum. TPE-AC is also photostable. All these findings have motivated its application in LSCs.18
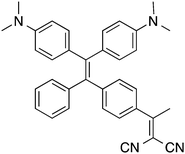 |
| Fig. 1 Chemical structure of TPE-AC. | |
The optical features of TPE-AC have been investigated when dispersed in transparent amorphous poly(methyl methacrylate) (PMMA) and polycarbonate (PC) films, i.e. the state-of-the-art in polymer matrices for LSCs.3 The thin film configuration was adopted since it allows an easy and fast set-up, which are the conditions required for the large-scale preparation of LSC samples. The light concentration and optical efficiencies of the derived LSCs were determined with a properly designed set-up.
Experimental
Materials
All the solvents were purchased from Sigma-Aldrich and used as received. Poly(methyl methacrylate) (PMMA, Aldrich, Mw = 350
000 g mol−1, acid number <1 mg KOH g−1) and random copolymer polycarbonate–polysiloxane LEXAN® EXL 1414T (PC, SABIC, Mw = 220
000 g mol−1 with 1.5 wt% Si) were used as received. TPE-AC was prepared following the synthetic procedure reported in the literature.18 Optically clear glass slides were obtained by cleaning in 6 M HCl for at least 12 h, rinsing with water, acetone and isopropanol and then drying for 8 h at 120 °C.
Preparation of TPE-AC/polymer films
Different TPE-AC/PMMA and TPE-AC/PC thin films were prepared by pouring about 0.8 mL chloroform solution containing about 30 mg of the polymer and the required amount of TPE-AC to reach concentrations of 0.1–1.5 wt% on a 35 × 50 mm glass surface. Solvent evaporation was carried out on a warm plate (30 °C) and in a closed environment. The film thickness was measured by a Starrett micrometer to be 25 ± 5 μm. The polymer films were easily removed with a spatula after immersion in water so that they could be stored for successive measurements by attaching them on a 50 × 50 × 3 mm optically pure glass substrate (Edmund Optics Ltd BOROFLOAT window 50 × 50 TS) with a high-purity silicone oil (poly(methylphenyl siloxane), 710 fluid, Aldrich, refractive index n = 1.5365). The absorption and emission properties of such devices showed negligible differences with the freshly prepared ones.
Characterization
Absorption spectra were recorded at room temperature on a Perkin-Elmer Lambda 650 spectrometer. Fluorescence spectra were measured at room temperature on a Horiba Jobin-Yvon Fluorolog®-3 spectrofluorometer equipped with a 450 W xenon arc lamp and double-grating for both the excitation and emission monochromators. The emission quantum yields of the solid samples were obtained by means of a 152 mm diameter “Quanta-phi” integrating sphere coated with Spectralon® and mounted in the optical path of the spectrofluorometer, using as an excitation source a 450 W xenon lamp coupled with a double-grating monochromator for selecting the wavelengths. Lifetime measurements were collected by using a Leica TCS SP5 SMD inverted confocal microscope (Leica Microsystems AG, Wetzlar, Germany) equipped with an external pulsed diode laser (PicoQuant GmbH, Berlin, Germany) for excitation at 470 nm. The laser repetition rate was set to 40 MHz. The images had a size of 256 × 256 pixels and were acquired with a scan speed of 400 Hz (lines per second). The pinhole aperture was set from 0.6 to 1.00 Airy, depending on the sample luminescence. The TPE-AC/polymer films fixed on microscope glass slides were viewed with a 20× 0.5 NA dry objective (Leica Microsystems). The images were collected using low excitation power at the sample (<10–20 μW). Emissions were monitored in the 600–700 nm range by acousto-optical tuneable beam splitter (AOBS) based built-in detectors. Acquisition lasted until about 250 photons per pixel were collected. Emission lifetime images (FLIM) of the TPE-AC/polymers were elaborated using Picoquant Symphotime software for FLIM analysis.
Optical efficiency measurement
Specially designed equipment was utilized, comprising a wooden box with darkened walls and a white back scattering floor (ERGA TAPES Srl Microcellular MCPET reflective sheet). The sample holder with the photovoltaic (PV) module (IXYS SLMD121H08L mono solar cell 86 × 14 mm: Voc = 5.04 V, ISC = 50.0 mA, FF > 70%, η = 22%, Fig. S3, ESI†) was suspended 2.5 cm above the floor. The PV module was masked so that the exposed surface matched the luminescent solar concentrator (LSC) edge (50 × 3 mm), with the aim to reduce the stray light to negligible levels. The remaining edges of the LSC were covered with aluminium tape. A led lamp (12 V DC 5 W colour temperature 5500 K) was housed in the top, 10 cm above the sample. The lamp's main body was outside the box to prevent the heating of the box. The PV module was connected to a digital potentiometer (AD5242) that was controlled via I2C by an Arduino Uno (http://https://www.arduino.cc) microcontroller using the I2C master library. A digital multimeter (KEITHLEY 2010) was connected in series with the circuit, between the PV module and the potentiometer, to obtain a characterization of the current as a function of the external load (Fig. S1, ESI†). The same multimeter was instead connected in parallel to the digital potentiometer while measuring the voltage (Fig. S2, ESI†). The Arduino Uno microcontroller unit was used to control the multimeter via the SCPI language over an RS-232 bus using a TTL to RS-232 converter chip (MAX232). The Arduino Uno unit was connected to a computer via the USB port and controlled by a Python script. A 12 V DC voltage light source was selected so that the luminous flux was continuous and there was no need to compensate the PV module capacitance. The measurement cycle began with a signal from the computer to the Arduino Uno unit, which set the multimeter parameter to measure current. Then, the Arduino Uno unit began the measurement loop, which comprised: (1) setting the potentiometer to a given value; (2) sending the trigger signal to the multimeter; (3) reading the measured data; and (4) sending the data back to the computer. The loop was repeated 256 times for potentiometer values ranging between 60 Ω and 1 MΩ. Then, the Arduino Uno unit set the multimeter to measure voltage and for each potentiometer value the system recorded eight data samples, which were subsequently processed by the Python script. The optical efficiency was reported as ηopt and obtained from the concentration factor, which was the ratio between the maximal current of the PV cell attached to the LSC edges under illumination of a light source and the maximal current of the bare cell put perpendicular to the light source. In our set-up, the QE variation of the PV module was under 2% within the range from 500 nm to 700 nm, i.e. matching the fluorescent emission spectra of TPE-AC.
Results and discussion
Spectroscopic characterization of TPE-AC/polymer films
TPE-AC has been recently designed and synthesized as a red-emitting AIE luminogen with excellent photostability. TPE-AC shows an absorption maximum at 455 nm and a faint emission in THF solution. At water fractions larger than 70% in THF, TPE-AC aggregates together, thus activating the AIE process and leading to the formation of highly emissive TPE-AC nanoparticles with fluorescence peaking at 705 nm.18
We therefore explored the use of TPE-AC as an accessible procedure to improve the performance of luminescent solar concentrators (LSCs). TPE-AC was dispersed at different contents (0.1–1.5 wt%) in transparent and amorphous polymers, such as poly(methyl methacrylate) (PMMA) and polycarbonate (PC). These concentrations were chosen to obtain homogeneous polymer films of a thickness of 25 ± 5 μm with optical characteristics that are not affected by spurious issues stemming from the high TPE-AC content, such as phase-separation at the film surface. PMMA and PC are amorphous matrices, transparent and commercially available, characteristics that make these polymers perfect candidates for large-scale LSCs applications.
The optical features of TPE-AC dispersed in PMMA and PC films follow a similar trend as a function of the dye content. Fig. 2a and b display the absorption characteristics of TPE-AC/PMMA and TPE-AC/PC films, respectively. TPE-AC shows a broad absorption band from 400–550 nm with the molecular absorption maxima at about 450 nm in PMMA and 470 nm in PC. Such difference may be ascribed to the higher dielectric constant of PC (2.8) compared to that of PMMA (2.3). It is worth noting that the broad absorption results were very favourable for a luminescent collector configuration, especially in harvesting photons in the UV-visible range. All the absorptions increased steadily with the TPE-AC concentration without levelling off at the highest content. Moreover, no evident absorption bands attributed to the formation of TPE-AC aggregates were present. This feature was also confirmed by the almost linear trend of the absorption maximum plotted as a function of TPE-AC content expressed as wt% (see Fig. 2, insets). Moreover, the slope of their linear fits, associated to the extinction coefficient of TPE-AC in polymer, was only slightly higher in the case of PC films than those based on PMMA, possibly due to the different dielectric constants.19
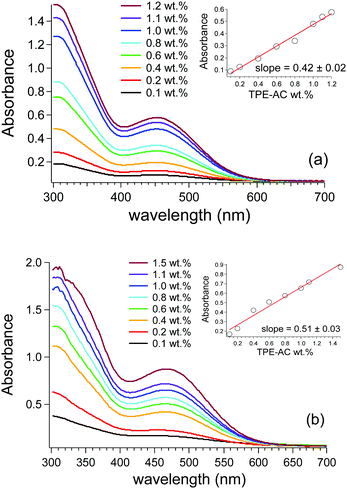 |
| Fig. 2 UV-vis absorption spectra of TPE-AC/PMMA (a) and TPE-AC/PC (b) films as a function of fluorophore concentration (wt%). In the insets, the absorbance maximum was plotted as a function of the TPE-AC content. | |
In contrast, TPE-AC/polymer films showed emission features that depended on the fluorophore concentration (Fig. 3a and b). When dispersed into polymers the TPE-based molecules exhibited typical AIE activity, stemming from the reduction of non-radiative decay pathways arising from intramolecular rotation. In other words, the rigid polymer matrix blocked the quenching rotations and activated the emission. When dispersed at low concentration (i.e. 0.1 wt%), TPE-AC showed a bright emission peaked at 600 nm and 620 nm in PMMA and PC, respectively, with a significant Stokes shift of 150 nm that limits the reabsorption phenomena. Notably, this emission range appeared to be optimal for LSCs, providing maximum spectral overlap with the external quantum efficiency of the Si-based PV cell (Fig. S3, ESI†). The emission intensity increased with the TPE-AC content up to 0.6–0.7 wt%, above which fluorescence quenching occurred, even if only partially. Indeed, the fluorescence of the TPE-AC/PC film containing the highest fluorophore content (i.e. 1.5 wt%) still appeared very brilliant (Fig. 3b, inset). Fluorescence quenching has been reported for highly conjugated D–A AIEgens that experience the formation of less emissive supramolecular (amorphous) aggregates due to intermolecular π–π interactions.20–23 Moreover, as evidenced by the progressive red-shift of the emission maxima with the TPE-AC content (Fig. 3a and b),24 auto-absorption phenomena occurred, which adversely affected the overall fluorescence of the polymer films.25
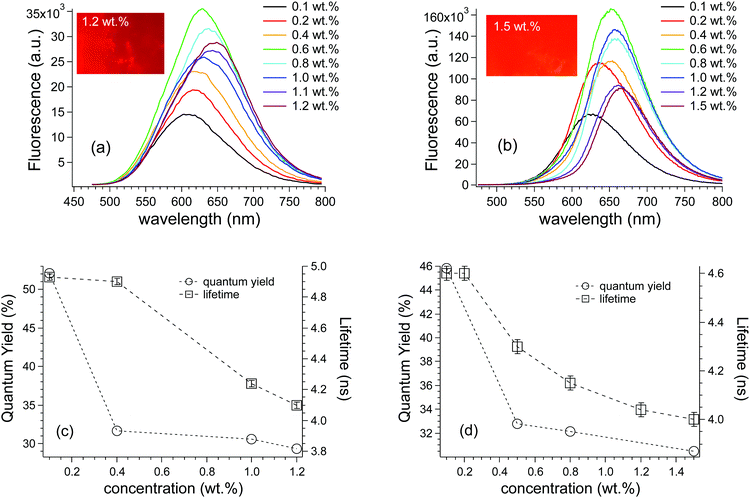 |
| Fig. 3 Fluorescence spectra, quantum yields (QY) and longer lifetime of TPE-AC/PMMA ((a and c), respectively) and TPE-AC/PC ((b and d), respectively) films as a function of fluorophore concentration (wt%). Pictures of the same films with the highest TPE-AC content were taken under illumination with a near-UV lamp at 366 nm and reported as insets. | |
The extent of fluorescence quenching was investigated by quantum yield (QY) measurements and lifetime experiments (Fig. 3c and d) since luminescence decreasing with fluorophore concentration adversely affects the LSC optical performances. QY was at a maximum for the lowest TPE-AC content in both polymer matrices, i.e. around 50% for the 0.1–0.3 wt% TPE-AC/polymer films. By increasing the fluorophore concentration, a sharp decline of QY occurred to reach values around 30% for the highest TPE-AC content investigated. Notably, QY decreasing appeared more gradual in the PC films, possibly indicating a higher compatibility of the TPE-AC molecules with this polymer matrix. Notwithstanding the evident decrease in QYs below those of the state-of-the-art LSCs systems,3,26 values as high as 30% are still considered good for fluorophores/polymer mixtures at such a long wavelength emission.11,27 A similar trend was also observed by looking at the lifetime parameters. At the lowest fluorophore content, TPE-AC/PMMA films were characterized by average lifetime parameters of 2.5 ns (26% population) and 4.9 ns (74% population), whereas TPE-AC/PC films showed a shorter-lived emission i.e. 2.1 ns (32% population) and 4.6 ns (68% population). This difference may be ascribed to the higher TPE-AC compatibility in PC, which limits the effects of the restricted intramolecular rotation, thus enabling more effective non-radiative decay channels.17 Moreover, by increasing the TPE-AC content, the average lifetime decreases for both polymer films, thus reaching values around 4 ns for the longer-lived population. This result is ascribed to the formation of amorphous supramolecular TPE-AC aggregates that besides showing lower QYs are also characterized by shorter lifetimes.28 However, about 70% of the population contributes to the longer-lived lifetime, which is still characteristic of TPE AIEgens with brilliant emissions.16
Optically efficiency determination of TPE-AC-based LSC
In order to assess the performances of LSCs, an optically pure 50 × 50 × 3 mm glass was coated with TPE-AC/polymer films with a thickness of 25 ± 5 μm. The photocurrent measurements were accomplished with a home-built apparatus by using a Si-based PV cell attached to one edge of the concentrator, as described in the experimental section. The optical efficiency ηopt (Table 1) was evaluated from the concentration factor C, which is the ratio between the short-circuit current measured in the case of the cell over the LSC edge (ILSC) and the short-circuit current of the bare cell when perpendicular to the light source (ISC) (eqn (1)): |  | (1) |
where G is the geometrical factor (G = 16.6), which is the ratio between the area exposed to the light source and the collecting area.
Table 1 Concentration factors (C) and optical efficiencies (ηopt) calculated for TPE-AC/polymer LSCs with different TPE-AC contents (wt%)
Entry |
C
|
η
opt (%) |
TPE-AC/PMMA (0.1 wt%) |
0.79 ± 0.02 |
4.8 |
TPE-AC/PMMA (0.2 wt%) |
0.84 ± 0.01 |
5.1 |
TPE-AC/PMMA (0.4 wt%) |
0.89 ± 0.03 |
5.4 |
TPE-AC/PMMA (0.6 wt%) |
0.87 ± 0.01 |
5.2 |
TPE-AC/PMMA (0.8 wt%) |
0.85 ± 0.02 |
5.1 |
TPE-AC/PMMA (1.0 wt%) |
0.86 ± 0.03 |
5.2 |
TPE-AC/PMMA (1.1 wt%) |
0.90 ± 0.04 |
5.4 |
TPE-AC/PMMA (1.2 wt%) |
0.89 ± 0.06 |
5.4 |
TPE-AC/PMMA (1.5 wt%) |
0.85 ± 0.04 |
5.1 |
|
TPE-AC/PC (0.1 wt%) |
0.93 ± 0.02 |
5.6 |
TPE-AC/PC (0.2 wt%) |
0.98 ± 0.04 |
5.9 |
TPE-AC/PC (0.4 wt%) |
1.03 ± 0.06 |
6.2 |
TPE-AC/PC (0.6 wt%) |
1.07 ± 0.01 |
6.5 |
TPE-AC/PC (0.8 wt%) |
1.11 ± 0.01 |
6.7 |
TPE-AC/PC (1.0 wt%) |
1.09 ± 0.02 |
6.6 |
TPE-AC/PC (1.2 wt%) |
1.03 ± 0.05 |
6.2 |
TPE-AC/PC (1.5 wt%) |
1.02 ± 0.05 |
6.1 |
Notably, C and ηopt increase for both TPE-AC LSCs as a function of the fluorophore concentration, according to the progressive amount of dispersed dye able to harvest UV-vis light irradiation. However, this trend was affected by dissipative phenomena, which is in agreement with the fluorescence quantum yield decreasing with concentration (Fig. 3) possibly due to auto-absorption phenomena.25 Notably, the progressive formation of less emissive TPE-AC aggregates counterbalances the effect provided by the increased number of available TPE-AC chromophores, thus adversely affecting the amount of light concentrated to the Si-based PV cell. Nevertheless, TPE-AC/PC LSCs showed optical performances higher than those calculated for TPE-AC/PMMA LSCs, with the maximum ηopt of 6.7 occurring for PC films containing about 1 wt% of TPE-AC. This value is comparable, and in some cases far greater, than that of most of the organic fluorophores (rhodamine B and perylene bisimides) investigated with the same LSC geometry and apparatus by our group.27,29,30 The superior performance evidenced by the PC-based LSC can be possibly attributed to a combination of effects: the first resides on the higher energy absorbed by the wider and more intense absorption band of TPE-AC dispersed within the PC matrix (see Fig. 2a and b); the second relies on the higher TPE-AC compatibility with PC, which allowed a less abrupt declining of the QY with the fluorophore content. Nevertheless, we are aware of and believe we can overcome the compatibility and auto-absorption issues associated with the use of TPE-AC in PMMA and PC matrices. A properly designed TPE-based AIEgen decorated with push–pull and compatibilizing moieties will be proposed in our next study in order to reach state-of-the-art concentration efficiencies.3,26
The ηopt variations as a function of TPE-AC concentration (Fig. 4) were also fitted with eqn (2):
| ηopt = ε′·c·e−μopt·c + D | (2) |
where
ηopt is a term proportional to the current generated by the Si-based PV,
c is the concentration of the dye in wt% and
ε′ and
μopt are two empirical constants defined as:
| ε′ ∝ h·e−![[l with combining macron]](https://www.rsc.org/images/entities/i_char_006c_0304.gif) | (3) |
| μopt ∝ μ′′(QY,p)·![[l with combining macron]](https://www.rsc.org/images/entities/i_char_006c_0304.gif) | (4) |
where
h is the thickness of the thin film,
![[l with combining macron]](https://www.rsc.org/images/entities/i_char_006c_0304.gif)
is the mean path length of the radiation in the optical system and
μ′′ is a term depending on both QY and the probability of fluorescence reabsorption (
p).
D is an empirical constant added since even a transparent and clear material is capable of trapping light due to scattering phenomena from surface and bulk defects.
Eqn (2) is based on the work of Goezberger.
10 Both
ε′ and
μopt are empirical parameters and their determination is considered straightforward for analysing the performances of different LSCs with the same geometry. Notably,
ε′ is a coefficient associated with the absorption features of the fluorophore/polymer system, whereas
μopt integrates all the fluorescence quenching phenomena. An optimal fluorophore/polymer LSC system should therefore exhibit a high
ε′ and a small
μopt so that the maximum efficiency is shifted to higher concentrations and the curve steadily ascends due to the direction of the linear part (
eqn (2)). A detailed determination of
eqn (2) was reported in the literature by our group.
30 The fitting parameters of the photocurrents are reported in
Table 2.
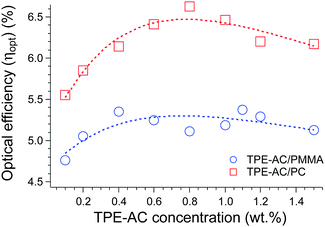 |
| Fig. 4 Optical efficiency (ηopt) % variation of TPE-AC/PMMA and TPE-AC/PC LSCs as a function of fluorophore content. The curves were fitted with eqn (1) with the parameters listed in Table 2. | |
Table 2 Fitting parameters of the photocurrent data measured for TPE-AC/PMMA and TPE-AC/PC LSCs
Entry |
ε′ |
μ
opt
|
D
|
TPE-AC/PMMA |
2.39 ± 0.98 |
1.36 ± 0.22 |
4.64 ± 0.17 |
TPE-AC/PC |
4.77 ± 0.81 |
1.28 ± 0.09 |
5.10 ± 0.14 |
The fitting parameters of TPE-AC/PMMA and TPE-AC/PC LSCs appeared to be similar. Notably, D values were also equivalent for both systems, showing the contribution of non-fluorescent trapping was almost the same for samples with the same geometry. Nevertheless, it seemed that the former system was characterized by a lower ε′ value. The lower ε′ is possibly the result of the less intense absorption band of TPE-AC in PMMA compared to that in PC (Fig. 2a and b), as ε′ is related to the light harvesting features of the system.
Conclusions
We demonstrated that TPE-AC, a red-emitting AIEgen with D–A features, once dispersed into PMMA and PC transparent amorphous matrices, confers to the resulting thin films optical efficiencies, which make them suitable for the preparation of LSCs. The TPE-AC/polymer films showed fluorescence peaking in the range of 600–620 nm with a high QY (about 50%) and were adversely affected by auto-absorption phenomena only in part. Notably, the QY experiments and lifetime measurements demonstrated that the optical characteristics of the films at the highest TPE-AC content are considered still significant for fluorophores/polymer mixtures at such a long wavelength emission. Spectroscopic investigations also revealed higher absorption and emission efficiencies of TPE-AC when dispersed in PC, possibly attributable to the better compatibility of the fluorophore within this polymer matrix. In light of these peculiar features, TPE-AC/PC LSCs yielded optical efficiencies of maximum 6.7%, which are comparable and even far greater than that gathered from LSCs based on red-emitting fluorophores in the same range of concentrations and geometrical factors. Future approaches for ηopt enhancement should adopt new synthetic strategies aimed at improving the fluorophore compatibility within polymer matrices as well as limiting the auto-absorption phenomena to definitely limit the efficiency losses due to fluorescence quenching. Moreover, the combination of compatible AIEgens would also offer the possibility to enhance the spectral window of absorption of the LSC and a cascade of emission via fluorescence resonance energy transfer (FRET).31–33 Nevertheless, all our findings consistently support the effective use of red-emitting AIEgens in the realization of efficient LSCs.
Acknowledgements
The research leading to these results has received funding from MIUR-FIRB (RBFR122HFZ) and in part from the Università di Pisa under PRA 2015 (project No. 2015_0038), and the Innovation and Technology Commission (ITC-CNERC14SC01). Prof. Gennaro Pescitelli is kindly acknowledged for helpful discussions.
Notes and references
- N. Armaroli and V. Balzani, Chem. – Eur. J., 2016, 22, 32–57 CrossRef CAS PubMed
.
- M. Debije, Nature, 2015, 519, 298–299 CrossRef CAS PubMed
.
- M. G. Debije and P. P. C. Verbunt, Adv. Energy Mater., 2012, 2, 12–35 CrossRef CAS
.
-
J. L. Sawin, Renewables Global Status Report, Renewable Energy Policy Network for the 21th Century, Paris, 2014.
- W. G. J. H. M. van Sark, Renewable Energy, 2013, 49, 207–210 CrossRef CAS
.
-
BIPV Technologies and Markets 2015-2022, Dublin, Ireland, 2015.
- Y. Zhou, D. Benetti, Z. Fan, H. Zhao, D. Ma, A. O. Govorov, A. Vomiero and F. Rosei, Adv. Energy Mater., 2016, 6, 1501913 CrossRef
.
- M. Carlotti, G. Ruggeri, F. Bellina and A. Pucci, J. Lumin., 2016, 171, 215–220 CrossRef CAS
.
- R. Turrisi, A. Sanguineti, M. Sassi, B. Savoie, A. Takai, G. E. Patriarca, M. M. Salamone, R. Ruffo, G. Vaccaro, F. Meinardi, T. J. Marks, A. Facchetti and L. Beverina, J. Mater. Chem. A, 2015, 3, 8045–8054 CAS
.
- A. Goetzberger and W. Greube, Appl. Phys., 1977, 14, 123–139 CAS
.
-
B. Valeur and M. N. Berberan-Santos, Molecular Fluorescence: Principles and Applications, Wiley-VCH, Weinheim, Germany, 2nd edn, 2013 Search PubMed
.
- J. L. Banal, J. M. White, K. P. Ghiggino and W. W. H. Wong, Sci. Rep., 2014, 4, 4635 Search PubMed
.
- J. L. Banal, B. Zhang, D. J. Jones, K. P. Ghiggino and W. W. H. Wong, Acc. Chem. Res., 2017, 50, 49–57 CrossRef CAS PubMed
.
- Y. Hong, W. Y. Lam Jacky and Z. Tang Ben, Chem. Soc. Rev., 2011, 40, 5361–5388 RSC
.
- R. Hu, N. L. C. Leung and B. Z. Tang, Chem. Soc. Rev., 2014, 43, 4494–4562 RSC
.
- J. Mei, N. L. C. Leung, R. T. K. Kwok, J. W. Y. Lam and B. Z. Tang, Chem. Rev., 2015, 115, 11718–11940 CrossRef CAS PubMed
.
- G. Iasilli, A. Battisti, F. Tantussi, F. Fuso, M. Allegrini, G. Ruggeri and A. Pucci, Macromol. Chem. Phys., 2014, 215, 499–506 CrossRef CAS
.
- M. Kang, X. Gu, R. T. K. Kwok, C. W. T. Leung, J. W. Y. Lam, F. Li and B. Z. Tang, Chem. Commun., 2016, 52, 5957–5960 RSC
.
-
T. Welton and C. Reichardt, Solvents and Solvent Effects in Organic Chemistry − Fourth, Updated and Enlarged Edition, Wiley-VCH, Weinheim, Germany, 4th edn, 2011 Search PubMed
.
- J. Zhou, Z. Chang, Y. Jiang, B. He, M. Du, P. Lu, Y. Hong, H. S. Kwok, A. Qin, H. Qiu, Z. Zhao and B. Z. Tang, Chem. Commun., 2013, 49, 2491–2493 RSC
.
- F. Ciardelli, G. Ruggeri and A. Pucci, Chem. Soc. Rev., 2013, 42, 857–870 RSC
.
- H. Li, Z. Chi, X. Zhang, B. Xu, S. Liu, Y. Zhang and J. Xu, Chem. Commun., 2011, 47, 11273–11275 RSC
.
- G. Singh, V. Bhalla and M. Kumar, Phys. Chem. Chem. Phys., 2015, 17, 22079–22089 RSC
.
- F. Donati, A. Pucci, C. Cappelli, B. Mennucci and G. Ruggeri, J. Phys. Chem. B, 2008, 112, 3668–3679 CrossRef CAS PubMed
.
- R. W. Olson, R. F. Loring and M. D. Fayer, Appl. Opt., 1981, 20, 2934–2940 CrossRef CAS PubMed
.
- W. G. J. H. M. v. Sark, K. W. J. Barnham, L. H. Slooff, A. J. Chatten, A. Bçhtemann, A. Meyer, S. J. McCormack, R. Koole, D. J. Farrell, R. Bose, E. E. Bende, A. R. Burgers, T. Budel, J. Quilitz, M. Kennedy, T. Meyer, C. D. M. Doneg, A. Meijerink and D. Vanmaekelbergh, Opt. Express, 2008, 16, 21773–21792 CrossRef PubMed
.
- A. Pucci, M. Pavone, P. Minei, A. B. Munoz-Garcia, E. Fanizza, P. Cimino and A. Rodriguez, RSC Adv., 2016, 6, 17474–17482 RSC
.
- Y. Yuan, L. Zhang, L. Hu, W. Wang and G. Min, J. Solid State Chem., 2011, 184, 3364–3367 CrossRef CAS
.
- J. Lucarelli, M. Lessi, C. Manzini, P. Minei, F. Bellina and A. Pucci, Dyes Pigm., 2016, 135, 154–162 CrossRef CAS
.
- M. Carlotti, A. Panniello, E. Fanizza and A. Pucci, Sol. Energy, 2015, 119, 452–460 CrossRef CAS
.
- B. Balaban, S. Doshay, M. Osborn, Y. Rodriguez and S. A. Carter, J. Lumin., 2014, 146, 256–262 CrossRef CAS
.
- S. T. Bailey, G. E. Lokey, M. S. Hanes, J. D. M. Shearer, J. B. McLafferty, G. T. Beaumont, T. T. Baseler, J. M. Layhue, D. R. Broussard, Y.-Z. Zhang and B. P. Wittmershaus, Sol. Energy Mater. Sol. Cells, 2007, 91, 67–75 CrossRef CAS
.
- M. J. Currie, J. K. Mapel, T. D. Heidel, S. Goffri and M. A. Baldo, Science, 2008, 321, 226–228 CrossRef CAS PubMed
.
Footnote |
† Electronic supplementary information (ESI) available: Scheme of the apparatus utilized for the LSCs performances; EQE of the Si-PV cell. See DOI: 10.1039/c7qm00008a |
|
This journal is © the Partner Organisations 2017 |
Click here to see how this site uses Cookies. View our privacy policy here.