DOI:
10.1039/C6QM00141F
(Review Article)
Mater. Chem. Front., 2017,
1, 231-250
Revisiting one-dimensional TiO2 based hybrid heterostructures for heterogeneous photocatalysis: a critical review
Received
27th July 2016
, Accepted 5th September 2016
First published on 16th September 2016
Abstract
One-dimensional (1D) TiO2 nanostructures have been regarded as ideal candidates for solar energy conversion due to their unique structural merits including large surface-to-volume ratio, fast and long-distance charge transport, and good physi-co-chemical stability. Over the past few decades, tremendous interest has been devoted to fabricating a large number of 1D TiO2 based heterostructures for extensive photocatalytic applications. In this review article, we provide a comprehensive and systematic discussion on the latest developments of a large variety of 1D TiO2 based composite heterostructures along with their versatile photocatalytic applications in various research fields including nonselective photodegradation of organic pollutants for environmental remediation, photocatalytic selective organic transformation, as well as solar energy conversion for fuel production. Furthermore, future challenges and promising perspectives for this increasingly booming photocatalytic technology are also presented. It is anticipated that this review article will provide enriched and useful information on rational utilization of the outstanding structure and electronic properties of 1D TiO2 nanostructures for a wide range of photocatalytic applications.

Fang-Xing Xiao
| Fang-Xing Xiao obtained his BS and MS degrees in Huaqiao University in 2006 and 2009, respectively, and obtained his PhD degree from the Fuzhou University in 2013. Currently, he works as a research fellow in Prof. Dr Bin Liu's group at Nanyang Technological University. His research interest focuses on the assembly of hybrid semiconductors for photocatalytic and photoelectrochemical investigations. |

Bin Liu
| Bin Liu received his BEng and MEng in chemical engineering from the National University of Singapore with Professor Hua Chun Zeng and PhD in chemical engineering from University of Minnesota with Professor Eray S. Aydil. He also worked as a postdoctoral researcher in the Department of Chemistry at University of California, Berkeley with Professor Peidong Yang. He is currently an Assistant Professor in School of Chemical and Biomedical Engineering at Nanyang Technological University. His current research involves architected nanomaterials for solar-to-fuel and solar-to-electric conversion, photocatalysis for air and water treatment, nanocomposites for electrocatalysis. |
1. Introduction
Recent years have witnessed an exponential growth in the synthesis of one-dimensional (1D) nanostructures and their widespread applications in photocatalysis. In particular, titanium dioxide (TiO2), as a quintessential and mostly investigated wide bandgap semiconductor, has been widely explored for extensive photocatalytic applications due to its good environmental friendless, excellent physical-co-chemical stability, and low cost.1,2 Among various types of TiO2 nanostructures, 1D TiO2 nanomaterials (e.g., nanowires, nanofibers, nanorods, nanobelts and nanotubes) have received the most research attention,3,4 because they demonstrate many unprecedented structural advantages over conventional nanoparticles or bulk materials in terms of fast and long-distance electron transport, larger specific surface area and pore volume as well as enhanced light absorption and scattering stemming from the high length-to-diameter ratio.3 Despite the aforementioned merits, two predominant drawbacks including high recombination rate of photogenerated electron–hole pairs and poor light absorption in the visible solar spectrum still hinder the developments of 1D TiO2 nanostructures, which lead to low quantum efficiency of photocatalytic reactions.5
To surmount these two obstacles, various synthetic strategies and structure modifications have thus been explored to reinforce the photoactivities of 1D TiO2 nanomaterials, such as metal or non-metal doping, noble metal deposition, as well as hetero-coupling/sensitizing with many narrow bandgap semiconductors.6 In general, these strategies are mainly based on certain design principles, such as forming Schottky/p–n heterojunctions, increasing the surface active sites, extending the optical absorption range, or engineering band structures to match the specific energy levels. Noteworthily, among which, rational design of 1D TiO2-based hybrid heterostructures has been deemed as the most attractive and effective route to fabricate numerous efficient composite photocatalysts considering the wide selection scope of semiconductors. Moreover, constructing composite heterostructures is able to compensate the drawbacks of single component and concomitantly benefit the synergistic interaction of ingredients to boost the solar energy conversion efficiency.7,8 Consequently, it is highly desirable to systematically summarize the latest developments of 1D TiO2 based nanomaterials in heterogeneous photocatalysis.9–22
To date, various excellent reviews have appeared at either a multitude of materials not limited to TiO2 composites or TiO2 across a wide range of fields.9,10 However, up to date, there is still lack of an exhaustive review on the rational design, fabrication, and clarification of a large variety of 1D TiO2 based composite nanostructures in conjunction with their versatile photocatalytic applications, which is of paramount importance to the researchers who engage in heterogeneous photocatalysis.11–22 Furthermore, objective evaluation on the photoactivities of various 1D TiO2 based nanomaterials to explore the correlation between structures and properties from a perspective of rational structural design has seldom been comprehensively summarized.
In this review article, we provide a systematic summary with a focus on the latest developments of 1D TiO2 based composite heterostructures for versatile photocatalytic applications, including nonselective photocatalytic degradation of organic pollutants for environmental remediation, photocatalytic selective organic transformation, and solar energy conversion to fuel production. Additionally, future challenges and perspectives for boosting the photocatalytic performances of 1D TiO2 based composite photocatalysts are discussed. It is hoped that our review article could inspire ongoing research interest in utilizing fascinating structure and electronic properties of 1D TiO2 to create more efficient 1D TiO2 based composite heterostructures for versatile photocatalytic applications.
2. Fundamentals of 1D semiconductor-based photocatalysis
2.1 Principle of semiconductor-based photocatalysis
Semiconductors without continuous electronic states have an energy gap, which is termed as the ‘bandgap’ from the top of the filled valence band (VB) to the bottom of the vacant conduction band (CB). The semiconductor can absorb light with incident photon energy larger than the bandgap (Eg) of the semiconductor. Therefore, electrons can be excited from the VB of the semiconductor to the CB. The basic principle of semiconductor-based photocatalysis is depicted in Fig. 1, which primarily includes three main steps: (1) production of electron–hole charge carriers via photoexcitation; (2) separation and diffusion of charge carriers to the semiconductor surface; (3) triggering oxidation and reduction reactions on the surface. It has been well established that electrons (e−) in the CB show a strong tendency for reduction reactions; while the holes (h+) in the VB show a strong oxidative potential.23 In this way, electrons and holes can serve as reductant and oxidant to efficiently react with various electron acceptors (R) and electron donors (D) adsorbed on the surface of semiconductor, respectively. Notably, recombination processes can simultaneously occur in the photocatalytic process, for which the excited electrons and holes within the bulk phase or on the surface can recombine and dissipate the input energy in the form of heat or emitted light. Based on the above analysis, it is apparent that recombination of electrons and holes is detrimental for efficient photocatalysis. Blank 1D TiO2 cannot serve as visible-light-responsive photocatalyst due to its wide bandgap (ca. 3.2 eV), which can only be photoexcited by UV light.24 Therefore, it is of great interest to design 1D TiO2 based hybrid heterostructures with a view to boosting photoactivities and broadening the light harvesting range.
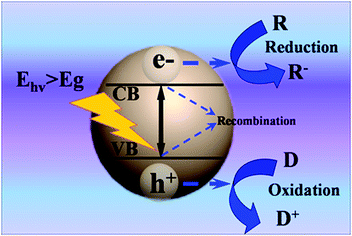 |
| Fig. 1 Basic principles of semiconductor-based photocatalysis; Ehv: irradiated photon energy, R: electron acceptor, D: electron donor. | |
2.2 Structural merits of 1D semiconductors
1D nanostructures such as nanowires, nanotubes, nanorods, and nanobelts have gained tremendous research attention in heterogeneous photocatalysis owing to their unique geometrical and electronic features, which can afford direct transport pathways for charge carriers and decouple the direction for light absorption and charge carrier transportation.25–27 Moreover, 1D semiconductor nanostructures are able to facilitate the light absorption and scattering, which is beneficial for photocatalytic reactions. In addition, it has been ascertained that subtle control over the physical parameters and components of materials can tailor the properties to attain the requirements for specific photocatalytic reactions.28–30 Consequently, 1D nanostructures provide a suitable platform to prepare efficient photocatalysts for solar energy conversion.
3. Synthetic strategies for 1D nanostructures
3.1 Top-down
Various top-down strategies have been developed to prepare 1D nanostructures including sculpting a nanostructure by etching and directly writing materials on a substrate.31 Among various top-down sculpting strategies, optical lithography (OL) has been applied as the most common technique, which is applicable to fabricate highly ordered 1D nanostructures with good control over configuration and size.32,33 Apart from OL technique, other lithography techniques including electron beam lithography (EBL),34 extreme ultra-violet (EUV),35 X-ray lithography (XRL),36 ion beam lithography (IBL),37 and electron beam induced deposition (EBID) lithography,38 are being developed to enrich lithography techniques for the fabrication of a large variety of 1D semiconductor nanostructures. The second category of top-down techniques mainly includes nano-imprint lithography (NIL)39 and scanning probe (SPL),40 by which directly “writing” materials onto a substrate is achieved. Although top-town methods can produce a large variety of 1D semiconductor nanostructures with high quality, many difficulties still need to be surmounted; for example, the methods need to be improved to be more time-effective and low-cost.
3.2 Bottom-up
The formation of 1D nanostructures through bottom-up approach is essentially a crystallization process, which includes nucleation and growth steps.41,42 Bottom-up growth possesses many advantages compared to the top-down methods in fabricating 1D nanostructures, for example, it benefits the preparation of self-assembled nanostructures which demonstrate unique integrative properties that are distinct from the properties shown by the individual constructing component. During the past few decades, various chemical routes have been explored as bottom-up methods, which achieve tunable control over dimensions, morphologies, and monodispersity of 1D nanostructures. Specifically, there are generally several synthetic strategies to fabricate 1D nanostructures based on bottom-up approach, including (1) preparation of 1D nanostructures using different 1D templates; (2) preparation of 1D nanostructures composed of 0D nanostructures via self-assembly buildup; (3) mediating the growth rate of different facets of a seed from a perspective of kinetic control using an appropriate capping reagent. Noteworthily, bottom-up approaches are not suitable for the formation of non-fluidic devices owing to the random positioning of the obtained nanostructures.43
3.3 Types and synthetic methods of 1D TiO2 nanostructures
Over the past decade, various 1D TiO2 nanostructures (e.g., nanorods, nanowires, nanofibers, nanotubes, and nanobelts) have been prepared for photocatalytic applications, which will be concisely summarized in the following part.
3.3.1 Nanotubes.
TiO2 nanotubes can be prepared by treating TiO2 powders with NaOH aqueous solution via hydrothermal process, which was first developed by Kasuga et al. in 1998.44,45 A 3D–2D–1D formation mechanism was proposed to elucidate the transformation process of TiO2 nanotubes.46 TiO2 nanotubes can also be attained using anodic alumina membrane (AAM) as the template via sol–gel method.47 Besides, a ZnO nanorod arrays on substrate can also be utilized as a template to prepare TiO2 nanotubes.48 Alternatively, anodic oxidation of Ti foil has been well-established as an efficient approach to prepare highly ordered self-aligned TiO2 nanotubes on conducting Ti substrate and this method can be extended to prepare many other transition metal oxide nanotube arrays at ambient conditions.49,50
3.3.2 Nanowires.
TiO2 nanowires have been extensively prepared by hydrothermal route. Typically, TiO2 nanowires can be prepared by treating TiO2 solid powders in a concentrated NaOH aqueous solution followed by hydrothermal reaction.51,52 Apart from it, TiO2 nanowires can also be obtained from layered titanate particles based on the hydrothermal method.53 In the formation of TiO2 nanowires from layered titanate particles, there are generally three processes: (i) exfoliation of layered Na2Ti3O7; (ii) formation of nanosheets; (iii) formation of nanowires. Highly ordered TiO2 nanowire arrays can be fabricated via electrophoretic deposition of TiO2 colloidal suspensions into an AAM template followed by removal of template.54 On the other hand, solvothermal method, physical vapor deposition (PVD) method,55 and electrodeposition method56,57 using the AAM template have also been developed to prepare TiO2 nanowires.58,59
3.3.3 Nanorods.
Analogous to nanotubes and nanowires, TiO2 nanorods can be prepared by sol–gel or solvothermal method using AAM or ZnO as template.60,61 Crystalline TiO2 nanorods have been prepared by oxidation of titanium plate with hydrogen peroxide,62–64 for which the oxygen source was revealed to play a crucial role. With the aid of various surfactants, TiO2 nanorods with different sizes can be synthesized.65,66 Besides, chemical vapor deposition (CVD)67 and microwave hydrothermal methods68 have also been developed to prepare TiO2 nanorods.
3.3.4 Nanobelts.
TiO2 nanobelts with a large percentage of exposed active facets in combination with exceptional physi-co-chemical properties have received enormous research interest for extensive applications.69 Various methods have been developed to synthesize TiO2 nanobelts including hydrothermal,70 electrochemical anodization,71 metallorganic CVD,72 and path-directed method.73 These methods show different levels of control over the synthetic parameters such as length and thickness.
3.3.5 Nanofibers.
Till now, the most frequently used method to prepare TiO2 nanofibers is electrospinning which is based on electrostatic force.74 It has been extensively used as a powerful tool to produce high-quality TiO2 nanofibers with nanometer scale diameter and large specific surface area.
4. 1D TiO2 based hybrid heterostructures for photocatalysis
4.1 Metal/1D TiO2 heterostructures
Metal nanoparticles (NPs) decorated 1D TiO2 nanostructures have been established as an important category of 1D TiO2 based nanocomposites owing to the formation of Schottky junction between metal and TiO2 as well as surface plasmon resonance (SPR) effect of metal components.75–78 When 1D TiO2 is closely integrated with a metal, a space-charge separation region can be created resulting in Schottky barrier. As shown in Fig. 2, Schottky barrier can serve as irreversible electron sink to trap photoelectrons from 1D TiO2 after bandgap excitation under light irradiation, which can remarkably improve the charge separation as well as create more active sites for photocatalytic reactions. For example, Xiao developed a facile layer-by-layer (LbL) assembly technique to synthesize well-defined M/TiO2 nanotube arrays (TNTs) heterostructures (M = Au, Ag, Pt), as shown in Fig. 3.79 It was found that these metal M/TNT hybrid nanostructures exhibited substantially enhanced photocatalytic performances toward organic dye degradation under UV light irradiation owing to the formation of Schottky barrier between metal NPs and TiO2 substrate. Besides, other synthetic approaches including photoreduction75,80 or chemical reduction method76 involving reducing agents have also been used to fabricate metal NPs functionalized 1D TiO2 composite photocatalysts.
 |
| Fig. 2 Schematic illustration of the Schottky barrier for metal/1D TiO2 nanostructures. | |
 |
| Fig. 3 (a) Top-view, (b) bottom, and (c) cross-sectional FESEM images of TNTs. FESEM images of (d) Au/TNTs, (e) Ag/TNTs, and (f) Pt/TNTs nanostructures prepared via LBL assembly method. (Reprinted with permission from the American Chemical Society.)79 | |
More Recently, SPR effect induced photocatalysis of metal/TiO2 nanostructures has attracted tremendous attention. Fig. 4 shows the schematic illustration for SPR-triggered charge transfer process for metal/TiO2 nanocomposites under visible light irradiation. It has been demonstrated that assembling metal NPs (e.g., Au, Ag, Pt) on 1D TiO2 framework is able to extend the optical absorption band edge of TiO2 from UV to visible spectrum because of the SPR effect afforded by the metal components.76,77,80,81 For example, as shown in Fig. 5, a well-defined CdS–Au–TiO2 nanorod array with sandwich structure has been constructed in which Au NPs are sandwiched between TiO2 nanorods and CdS quantum dots (QDs). The results showed that the unique ternary heterostructure was beneficial for charge separation and thus could significantly boost the solar energy conversion efficiency as compared to bi-junction counterpart.82 The role of Au NPs in this ternary heterostructure was revealed to act as a electron relay mediator which facilitates rapid charge transfer between CdS QDs and TiO2 when CdS QDs were photoexcited by light (λ < 525 nm). Moreover, it was found that Au NPs could simultaneously serve as a plasmonic photosensitizer, which contributes to the efficient solar-to-hydrogen conversion at longer wavelength (λ = 725 nm).
 |
| Fig. 4 Schematic illustration of charge transfer mechanism for metal/1D TiO2 nanostructures. | |
 |
| Fig. 5 Schematic illustration depicting the role of Au NPs in tuning the charge transfer process under light irradiation (λ < 525 nm), and hot electron transfer mechanism under light irradiation (λ = 725 nm). (Reprinted with permission from the American Chemical Society.)82 | |
4.2 Carbon/1D TiO2 composite heterostructures
Another attractive strategy to enhance photoactivities and solar energy conversion efficiency of 1D TiO2 is the coupling with carbon materials, such as graphene (GR), carbon nanotubes, carbon nanofibers, C60, and graphene quantum dots. Among which, GR has triggered a scientific “gold rush” by virtue of its excellent integrated properties, such as large specific surface area, excellent electrical conductivity, great electronic mobility and stability, thereby making it a promising candidate to form composite heterostructures with TiO2 with enhanced photoactivities.83–85
Several approaches have been reported to prepare GR/1D TiO2 composite heterostructures.86–88 For example, Lai's group developed an electro-deposition method combined with a carbonation technique to deposit GR film on TNT. The thus-obtained GR/TNT heterostructure exhibited significantly enhanced photoactivities toward degradation of MO under UV light irradiation as compared with pristine TNT and annealed TNT.86 Similarly, Xian et al. directly deposited a smooth GR film on the surface of TNTs using a simple electrochemical method.87 The as-prepared GR/TNTs heterostructure showed enhanced light absorption and photoactivity as compared to bare TNTs and P25 thin film.87 In other works, Sang et al. have constructed GR/TiO2 nanobelt heterostructures by wrapping TiO2 nanobelts with GR nanosheets via an in situ photoreduction method.88 As shown in Fig. 6, compared to blank TiO2 nanobelts, GR/TiO2 nanobelt heterostructures possess enhanced photoactivities for MO degradation and hydrogen production from water with excellent recyclability, which is mainly attributed to the formation of Ti–O–C bonds between GR and TiO2 nanobelts. Notably, intrinsic pronounced π–π interactions and high hydrophobicity of GR make it an excellent adsorbent toward MO molecules. Moreover, GR plays an important role as acceptor for photoelectrons excited from TiO2 nanobelts, thereby boosting the transfer of photogenerated electrons and prolonging the lifetime of electron–hole pairs over GR/TiO2 nanobelt heterostructures.
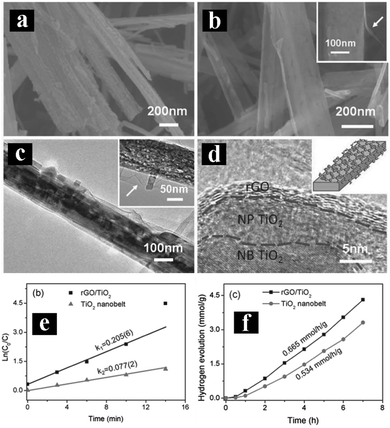 |
| Fig. 6 FESEM images of (a) TiO2 nanobelts, (b) GR/TiO2 nanobelts, (c) TEM image of GR/TiO2 nanobelts with magnified image in the inset; (d) HRTEM image of GR/TiO2 nanobelts with schematic model in the inset. (e) ln(C0/C) vs. irradiation time; (f) photocatalytic hydrogen generation over 1 wt% Pt-loaded GR/TiO2 and TiO2 nanobelt under UV irradiation. (Reprinted with permission from John Wiley & Sons, Inc.)88 | |
Carbon-based nanomaterials with sp2-hybridized atoms such as carbon nanotubes (CNTs) and carbon fibers (CFs) have also been well-established to demonstrate fascinating properties such as large specific surface area, high current density, good thermal and electrical conductivity, and fabulous chemical stability.89–92 For example, Sayed's group synthesized crystalline CNTs/TNTs membranes by chemical vapor deposition (CVD) of CNTs on TNTs and used the membranes for water splitting.90 Poddar's group reported the fabrication of CNTs/TiO2 nanorods heterostructure film on FTO through a simple low-temperature hydrothermal method.91 It was revealed that the heterostructure film exhibited a 60% increase in solar energy conversion efficiency compared with blank TiO2 nanorods.
Apart from CNTs, C60 has also attracted enormous attention for photocatalytic applications. For example, it was found that C60/TiO2 nanocomposites exhibited higher photoactivity compared with pure TiO2.93 In another work, C60/TiO2 nanorods (NRs) heterostructure was prepared by a hydrothermal method and its photoactivity was systematically probed.94 The results suggested that photocatalytic activity of C60/TiO2 NRs heterostructure was markedly improved under visible light irradiation as compared with pure TiO2 NRs and Degussa P25 counterparts, which was mainly ascribed to the intimate interfacial contact between C60 and TiO2 NRs. This interesting work indicated that C60 could be the potential candidate as an excellent electron acceptor to replace noble metals. Besides, GR quantum dots (GQDs), as a novel class of recently discovered carbon nanomaterials, have gained increasing interest because of its fascinating physi-co-chemical properties, up and down-converted photoluminescence, electron-accepting and -transporting properties.95 For example, GQDs modified TNT nanocomposite demonstrated significantly enhanced photoactivities toward degradation of MB under UV light irradiation, which is attributed to the efficient electron transfer from TiO2 to GQDs, thus prolonging the lifetime of charge carriers.96 As well, Pan et al. prepared GQDs sensitized anatase TNTs heterostructures by anodic oxidization. It was found that the GQDs/TNTs hybrid nanostructure demonstrated favorable photoactivity under visible light irradiation with negligible activity decrease relative to CdS and CdSe sensitized TNT nanocomposites, which generally suffer from serious photocorrosion.97
4.3 Semiconductor/1D TiO2 composite heterostructures
Semiconductor/1D TiO2 composite heterostructures can be divided into two categories: p–n and non-p–n heterostructures.98Fig. 7a shows the energy level configuration of p–n heterostructures, which is built at the interface between p and n-type semiconductors. 1D TiO2 nanostructures are typical n-type semiconductor and p-type semiconductors, such as NiO, Ag2O, Cu2O, can be coupled with 1D TiO2 to form the desired p–n heterojunction.99–101 The p–n junctions can absorb light with energy equal or larger than the bandgap (Eg) of the photocatalysts, based on which photogenerated electron–hole pairs can be rapidly separated by the electric field. Notably, the built-in electric field directs the flow of electrons to the CB of the n-type material and the holes can move to the VB of the p-type material, therefore leading to more efficient separation of photogenerated electron–hole pairs, longer life-span of charge carriers and increased reaction rate. For comparison, energy level of non-p–n heterojunction is also depicted in Fig. 7b which illustrates the optimal band position for efficient separation of charge carriers, in which the photogenerated electrons transfer from A to B on account of the more negative CB position of A. On the contrary, holes can travel in the opposite direction from the more positive VB of B to A. Thus, photoinduced electrons and holes are separated from each other, thereby prolonging the lifetime and remarkably reducing the recombination rate.
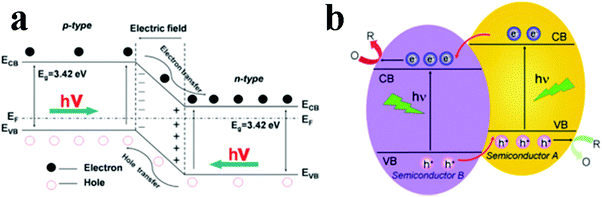 |
| Fig. 7 Two types of charge carriers transfer pathways for semiconductor composite heterostructures. (Reprinted with permission from Royal Society of Chemistry.)98 | |
4.3.1 Metal oxide/1D TiO2 heterostructures.
Metal oxides that have been used to modify 1D TiO2 are primarily centered on Ag2O, NiO, CuO, Cu2O, ZnO, Fe2O3, Sn3O4, Bi2O3 and ZnO, which are classified into three different types according to their light absorption properties, i.e., UV light, visible light and UV-vis light active metal oxide/1D TiO2 nanostructures.102–107 As typical UV light active metal oxide/1D TiO2 heterostructures, ZnO/TiO2 nanofibers core–shell nanocomposites were synthesized by Jiang's group via a combination of electrospinning and hydrothermal techniques.103 The morphologies of ZnO component could be tuned from nanorods to nanoplates by simply adjusting the experimental parameters. Pan et al. synthesized hierarchical ZnO/TiO2 nanobelt heterostructures via a facile hydrothermal method and used it as an efficient photoanode for photoelectrochemical water splitting.102 As visible-light-active metal oxide/1D TiO2 heterostructure, Bi2O3/TiO2 nanotube composite as a typical p–n heterojunction, was fabricated by an ultrasonication-assisted successive ionic layer adsorption and reaction (SILAR) strategy.106 The formation of p–n heterojunction facilitated the charge separation/transfer. In addition, Cu2O/TiO2 p–n heterojunction in which varied amounts of p-type Cu2O nanoparticles were deposited on n-type TNT by an ultrasonication-assisted sequential chemical bath deposition (CBD) approach.108 The results indicated that photoelectrochemical and photoelectrocatalytic activities of TNTs were significantly enhanced when optimal amount of Cu2O was deposited.108 Alternatively, it was reported that integration of WO3 with 1D TiO2 was in favor of improving the photocatalytic or photoelectrochemical activities of blank 1D TiO2.109,110 For example, Reyes-Gil's group prepared a well-defined WO3/TNTs heterostructure by anodization and electrodeposition for solar water splitting and wastewater treatment.109 The results suggested that the unique tubular structure and composition of composite heterostructures improved the solar spectrum absorption as well as photodegradation and photocurrent efficiencies, as compared to blank TNTs and WO3 counterparts.109 Besides, as shown in Fig. 8, WO3@TiO2@WO3 ternary core–shell heterostructures composed of WO3 nanoparticles uniformly decorated on the whole framework of highly porous TiO2 nanotubes were synthesized using an emulsion electrospinning technique followed by thermal evaporation and annealing.110 In comparison with binary WO3/TiO2 nanotubes and WO3/TiO2 nanofibers, WO3@TiO2@WO3 ternary heterostructures possessed substantially improved optical absorption and showed the optimal photocatalytic performances under both UV and visible light irradiation, which was ascribed to the high specific surface area and synergetic effects of 1D TiO2 and WO3 in WO3@TiO2@WO3 ternary heterostructures.
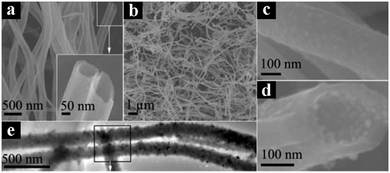 |
| Fig. 8 FESEM images of (a and b) porous WO3 nanotubes with an enlarged cross-section image in the inset; (c and d) are enlarged images of the wall and cross-section of the WO3@TiO2@WO3 ternary heterostructures with corresponding (e) TEM image. (Reprinted with permission from Royal Society of Chemistry.)110 | |
4.3.2 Chalcogenide/1D TiO2 composite heterostructures.
Recently, a large number of chalcogenide nanomaterials (e.g., In2S3, Ag2S, CdSe, PbS, BiS3, MoS2 nanoparticles, QDs or 2D nanosheet)/1D TiO2 heterostructures have been prepared via diverse synthetic strategies including cation exchange, electrochemical deposition, CBD, sonication-assisted ionic layer adsorption or hydrothermal approach.111–118 For example, high-performance MoS2/TNT heterostructures consisting of layered MoS2 nanoparticles on highly ordered anodized TNT have been prepared by a photocatalytic route.118 In another case, construction of few-layer MoS2 nanosheet coated TiO2 nanobelt heterostructures (TiO2@MoS2) with 3D hierarchical configuration was reported by a simple hydrothermal method.114 As shown in Fig. 9, core–shell TiO2@MoS2 heterostructures showed excellent photocatalytic hydrogen production activity and high photoactivities toward organic dye degradation, owing to the matched energy level of MoS2 and TiO2, thus suppressing the recombination of photogenerated electron–hole pairs. The chalcogenides typically act as narrow bandgap semiconductors, which demonstrate substantial absorption in the visible spectrum. Therefore, coupling chalcogenides with 1D TiO2 to form composite heterostructures could significantly increase light harvesting and thus boost photocatalytic performances.111,112,114 In terms of the photocatalytic mechanism, photoelectrons in the VB of chalcogenides are generally excited under light irradiation and then transferred to the CB of TiO2 due to their favorable band alignment.
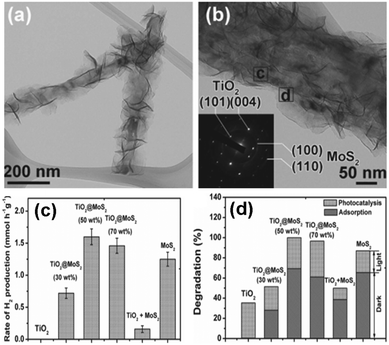 |
| Fig. 9 (a and b) TEM images of TiO2@MoS2 heterostructures, (c) photocatalytic activities of different samples, (d) adsorption of Rhodamine B in dark and photoactivities toward degradation of RhB over different samples. (Reprinted with permission John Wiley & Sons, Inc.)114 | |
4.3.3 Complex compound/1D TiO2 heterostructures.
Good chemical stability and desirable geometry of 1D TiO2 makes it a suitable candidate for the preparation of complex compound heterostructures, such as Bi2WO6/TiO2, Ag3PO4/TiO2, ZnFe2O4/TiO2, and etc.119–121
Recently, Fu's group prepared Bi2Ti2O7/TiO2 nanowire heterostructures via a two-step sequential solvothermal growth followed by calcination treatment.122 The 1D Bi2Ti2O7/TiO2 heterostructures effectively increases the separation of photogenerated charge carriers and thus enhances the visible-light-driven self-cleaning properties. This method can also be extended to the synthesis of other complex compound heterostructures, e.g., SrTiO3 or Bi4Ti3O12/1D TiO2 heterostructures.123,124 In another work, BiOI/TNT has also been successfully prepared by the sequential chemical bath deposition (S-CBD) method,125 for which the p–n heterojunctions with unique flake-tube structure along with larger surface area and strong visible light absorption concurrently give rise to the remarkably enhanced visible-light-driven photoelectrocatalytic performances and stability. Moreover, some other complex chalcogenide compounds, such as ZnIn2S4 and CuInS2, have also been considered to be the active component for the preparation of hybrid heterostructures.126,127
4.4 Polymer/1D TiO2 heterostructures
When conducting polymers such as polyaniline, polypyrrole (PPy), poly(3-hexylthiophene) or their derivatives are combined with 1D TiO2, the thus-formed composite heterostructures possess some unique physical and electrical properties including increased electrical conductivity, flexibility, and tunable band structure.128–130 Among various conducting polymers, PPy has attracted the most research attention owing to its high conductivity and good stability. For example, Sutter's group investigated the preparation of pyrrole/TNT composite heterostructures via an electro-polymerization method by tuning pulses of light and potential. They found that the highly ordered TNT as the substrate could provide a high surface area for uniform deposition of PPy and vertical pathways for transport of photogenerated electrons.129 Wan et al. reported that TiO2 nanotubes linked with H2TCPP (meso-tetra(4-carboxyphenyl)porphyrin) could serve as efficient visible-light photocatalysts for the degradation of organic dye pollutants. Moreover, the authors found that the H2TCPP molecule acted as an effective sensitizer to broaden the light absorption, narrow the bandgap, and enhance the photocatalytic activity of TNT under visible light irradiation.
4.5 1D TiO2 based multiphase heterostructures
Many researchers have devoted to understanding the role of TiO2-based multiphase heterostructures in enhancing the separation of photogenerated charge carriers in photocatalytic systems for solar energy conversion.131–136 The multiphase heterostructures composed of mixed crystalline phases of TiO2 (e.g., rutile, anatase, brookite and TiO2(B)) show enhanced photoactivity by virtue of the efficient separation of photogenerated charge carriers in different phases. The differences in lattice structures can cause different electronic densities and band structures, thus leading to slight difference in their band alignments. For example, Zhang's group developed 1D anatase/TiO2(B) nanotube heterostructures through a novel two-step alkaline hydrothermal route followed by ion exchange and calcination.135 As shown in Fig. 10, anatase/TiO2(B) heterostructure shows improved photoactivities compared with the single phase (anatase or TiO2(B)) and P25 for degradation of acetaldehyde and photocatalytic water splitting under UV light irradiation, which is mainly due to the synergistic effect of high specific surface area and improved separation efficiency of electron–hole pairs induced by the unique heterostructure of anatase/TiO2(B) nanocomposites. The type II band alignment with photogenerated electrons transferred from anatase to TiO2(B) was confirmed by the systematic analysis of DRS, XPS, Mott–Schottky measurements and EPR. In a similar work, Aydil's group has prepared TiO2(B) core and anatase shell (i.e., TiO2(B)@anatase) core–shell nanowires heterostructures via a facile solution synthesis method.137 As clearly shown in Fig. 11, TiO2(B)@anatase core–shell nanowires heterostructures show enhanced photoactivity toward MO degradation compared with the single-component counterparts due to the favorable band alignment between TiO2(B) and anatase TiO2 and the formations of well-defined 1D core–shell heterojunctions, thus resulting in prolonged lifetime of photogenerated charge carriers.
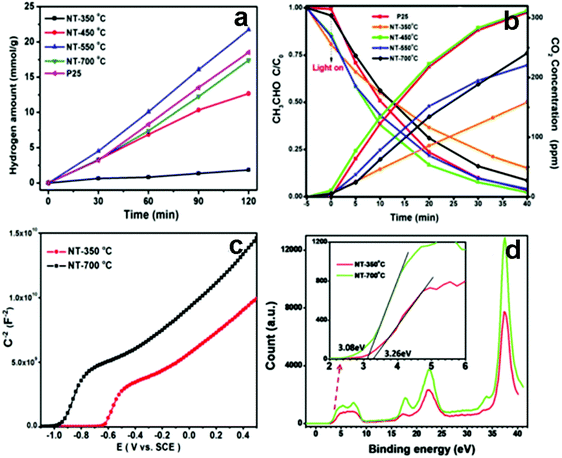 |
| Fig. 10 (a) Photocatalytic hydrogen production over different TiO2 samples, (b) photocatalytic acetaldehyde degradation under UV light irradiation. (c) Mott–Schottky and (d) valence band XPS spectra of different TiO2 samples, inset: local magnified valence band XPS spectra. (Reprinted with permission from Royal Society of Chemistry.)135 | |
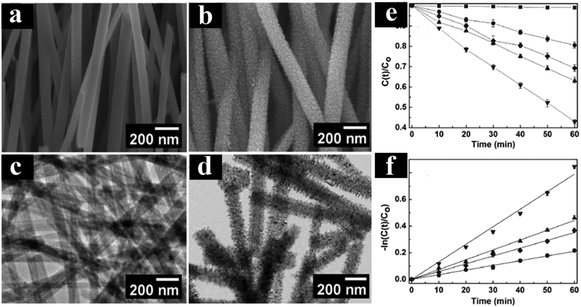 |
| Fig. 11 (a and c) FESEM and TEM images of H2Ti2O5·H2O nanowires. (b and d) FESEM and TEM images of TiO2(B)@anatase core–shell nanowires. (e and f) Photoactivities of different samples under UV light irradiation. (Reprinted with permission from American Chemical Society.)137 | |
Apart from TiO2(B)@anatase multiphase heterostructures, 1D rutile@anatase TiO2 core–shell heterostructure has also been explored by many groups.133,134,138,139 For example, as shown in Fig. 12, Li's group demonstrated a systematic study on the synthesis of 1D rutile@anatase TiO2 nanowire heterostructures by treating rutile TiO2 nanowires with titanium precursor solutions (e.g., TiCl4, Ti(OBu)4, or Ti(OiP)4) followed by a post-annealing process, which resulted in the in situ formation of anatase TiO2 layer on the outer surface of rutile nanowires.139 Compared to pristine TiO2 nanowires, all rutile@anatase TiO2 nanowire heterostructures exhibited significantly improved photocurrent responses under the illumination of white light, which was mainly ascribed to the effective passivation of surface states on the rutile surface which retarded the charge recombination arising from trap states, thus facilitating the interfacial charge transfer and improving the overall carrier utilization efficiency.
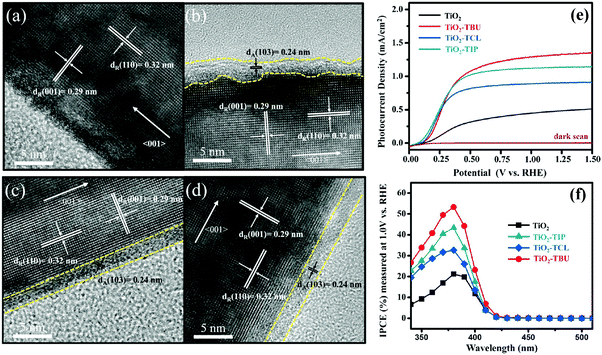 |
| Fig. 12 HRTEM images of (a) pristine TiO2, (b) TiO2-TIP, (c) TiO2-TCL, and (d) TiO2-TBU nanowire arrays prepared using different precursors. (e) Linear sweep voltammograms of pristine TiO2, TiO2-TCL, TiO2-TIP, and TiO2-TBU nanowire electrodes in the dark and under white light illumination (AM 1.5G, 100 mW cm−2). (f) IPCE results of pristine TiO2, TiO2-TCL, TiO2-TIP, and TiO2-TBU nanowire at 1 V vs. RHE. (Reprinted with permission from American Chemical Society.)139 | |
5. Photocatalytic applications of 1D TiO2 based composite heterostructures
5.1 Nonselective removal of organic pollutants
In recent years, photocatalytic nonselective degradation of organic pollutants over 1D TiO2 based heterostructures has become a promising route for environmental remediation.140–144 For example, Xiao et al. fabricated hierarchically ordered ZnO NRs or ZnO nanoparticles decorated TNT heterostructures towards degradation of RhB under UV light irradiation.140,141 As shown in Fig. 13, ZnO NRs decorated nanoporous-layer-covered TiO2 nanotube arrays (ZnO NRs/NP-TNTAs) heterostructures exhibit significantly enhanced photocatalytic performances as compared to the single component counterparts, which is attributed to the intimate interfacial contact between ZnO NRs and NP-TNTAs afforded by the hierarchical 1D heterostructures, thereby leading to prolonged life-span of photogenerated electron–hole pairs. According to a series of control experiments in N2 and O2, as well as with adding different scavengers for quenching hydroxyl radicals (˙OH), holes (h+), electrons (e−), and superoxide radicals (O2˙−), photocatalytic mechanism was proposed.
 |
| Fig. 13 (a) Photoactivities of NP-TNTAs, ZnO NRs film, and ZnO NRs/NP-TNTAs heterostructure toward degradation of RhB under UV light irradiation (365 ± 15 nm), (b) control experiments of ZnO NRs/NP-TNTAs heterostructure, top-view FESEM images of (c) NP-TNTAs and (d) ZnO NRs/NP-TNTAs heterostructure, and low-magnified (e and f) and high-magnified (g and h) cross-sectional FESEM images of NP-TNTAs and ZnO NRs/NP-TNTAs heterostructure, respectively. (Reprinted with permission from Royal Society of Chemistry.)141 | |
More recently, Kang's group employed gold nanoparticles (Au NPs) coating on the surface of TiO2 nanowires which were subjected to nitridation treatment (TiO2-NWs) for photocatalytic degradation of MO under visible light irradiation.142 Both nitridation treatment and Au NPs decoration afforded superior photocatalytic activities of Au/TiO2-NWs heterostructures for organic dye pollutant removal in comparison with pristine TiO2-NWs. The synergistic effect of nitrogen-doping and localized plasmon excitation of Au NPs on the photocatalytic dye degradation was elucidated.142 In another work, Zhou et al. reported the synthesis of PdO/TiO2 and Pd/TiO2 nanobelts heterostructures (with mean diameters of 1.3 and 1.6 nm for PdO and Pd, respectively) via a facile co-precipitation approach combined with a reduction process.143 It was found that PdO/TiO2 nanobelts heterostructures exhibited the highest photoactivity toward degradation of MO, methylene blue (MB), rhodamine B (RhB) and p-chlorophenol under UV-visible light irradiation, which was ascribed to the energy-level matching and p–n heterojunction effects for PdO/TiO2 nanobelts and Schottky barrier effect for Pd/TiO2 nanobelts, respectively. Notably, plasmonic excitation and quantum-size effects of Pd/TiO2 nanobelts were simultaneously observed under visible light irradiation.
In addition to the above 1D TiO2 based binary heterostructures, ternary heterostructures have also been explored for photocatalytic removal of organic dye pollutants.144 Taking TiO2 nanobelts as a substrate, Liu's group fabricated the surface-coarsened TiO2 nanobelts (SC-TNBs) which were closely enwrapped with Ag NPs and GR nanosheets (i.e., Ag/GR/SC-TNBs) using a facile and green self-assembly strategy combined with a photoreduction method. As shown in Fig. 14, compared with blank SC-TNBs and binary heterostructures, Ag/GR/SC-TNBs ternary heterostructures show substantially improved photoactivities toward RhB degradation under UV light irradiation on account of the construction of two heterojunctions among the assembly units, which promotes the cascade electrons transfer and thus prolongs the lifetime of photogenerated electron–hole pairs. Meanwhile, Ag NPs act as efficient “electron reservoirs” and GR nanosheets serve as electron transporters and collectors over ternary heterostructures in the photocatalytic process.
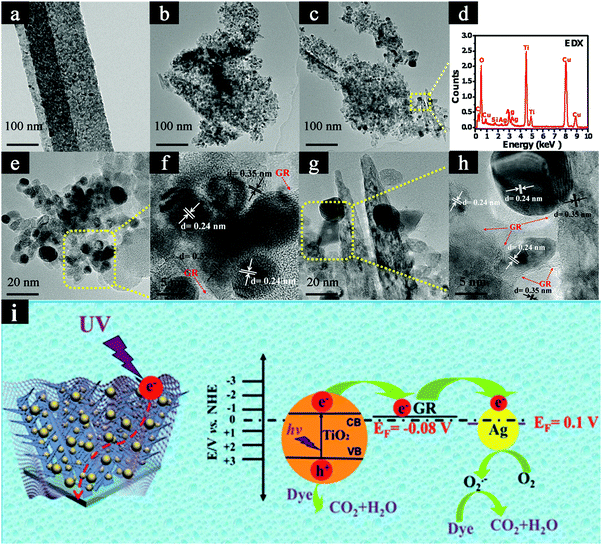 |
| Fig. 14 TEM images of (a) TNBs, (b) SC-TNBs, (c, e and g) Ag/GR/SC-TNBs ternary heterostructure, (f and h) HRTEM images, and (d) EDX result of Ag/GR/SC-TNBs ternary heterostructure. (i) Proposed photocatalytic mechanism of Ag/GR/SC-TNBs heterostructure. (Reprinted with permission from Royal Society of Chemistry.)144 | |
5.2 Selective organic transformation
Photocatalytic selective organic transformations including selective oxidation of alcohols and reduction of nitro compounds to amino compounds, have been deemed as one of the most vital reactions in both industrial and laboratory syntheses owing to their environmental friendliness which avoids using detrimental heavy metal catalysts, strong chemical oxidants, reducing agents, and/or harsh reaction conditions, thus possessing great potential for green chemicals production.145–147
To date, many examples regarding using 1D TiO2 based composite heterostructures for photocatalytic selective oxidation of alcohols have been reported. For example, Liu's group have systematically studied the photocatalytic performances of various metal/1D TiO2 nanobelts (TiO2 NBs) heterostructures, for example, Pd/TiO2, Au/TiO2, Cu/TiO2, Au–Cu/TiO2 and Au–Pd/TiO2 for aerobic selective oxidation of alcohols to their corresponding aldehydes.148,149 More specifically, Au or bimetallic Au–Pd alloy NPs decorated 1D TiO2 NBs were synthesized by a deposition–precipitation method.148 The results indicated that Au–Pd/TNBs heterostructures demonstrated a remarkably improved visible-light-driven photoactivity compared to the monometallic Au/TNBs or Pd/TNBs due to the plasmon-mediated charge distribution from Au sites to Pd sites within alloy NPs. The authors also prepared Au–Cu bimetallic NPs supported TiO2 NBs by a one-pot photodeposition-galvanic replacement method,149 which enabled the tunable catalytic properties via controlling the ratio of Au and Cu. Furthermore, it was found that Au–Cu/TiO2 NBs ternary heterostructure exhibited significantly enhanced photocatalytic performances for aerobic oxidation of benzyl alcohol to benzaldehyde compared with their monometallic counterparts. In another work, Wu's group reported a facile electrospinning method to synthesize CdS/TiO2 nanofibers (NFs) heterostructures for selective oxidation of alcohols under visible light irradiation.150 As shown in Fig. 15, CdS/TiO2 NFs heterostructures exhibited the highest benzyl alcohol conversion (ca. 22%), and benzaldehyde selectivity (ca. 99%) in the presence of visible light irradiation compared with blank TiO2 NFs, CdS and TiO2/CdS composite which was prepared by simply mixing TiO2 NFs and commercial CdS. The mechanism for visible-light-driven photocatalytic selective oxidation of alcohols to aldehydes over 1D CdS/TiO2 NFs heterostructures was further probed. The aforementioned works offer enriched information on the rational design and construction of advanced 1D TiO2 based composite photocatalysts for selective oxidation reactions.
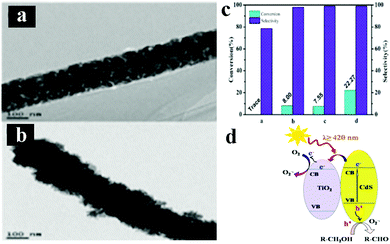 |
| Fig. 15 TEM images of (a) TiO2 NFs, (b) CdS/TiO2 NFs heterostructure, (c) photocatalytic selective oxidation of benzyl alcohol over TiO2 NFs, CdS, mixed TiO2/CdS, and CdS/TiO2 heterostructure under visible light irradiation (λ ≥ 420 nm). (d) Possible photocatalytic mechanism. (Reprinted with permission from American Chemical Society.)150 | |
Besides selective oxidation reactions, recent work has highlighted the photoreduction of different nitro compounds to amino compounds under ambient conditions.151–153 For instance, our group prepared CdS QDs decorated NP-TNTAs (CdS–NP-TNTAs) hybrid heterostructures via a spontaneous self-assembly route, by which tailor-made CdS QDs with negatively charged surface were uniformly deposited on a hierarchically ordered NP-TNTAs scaffold by substantial electrostatic interactions.151 The thus-assembled CdS–NP-TNTAs heterostructure exhibited the optimal visible-light-responsive photocatalytic performances toward reduction of nitrophenol derivatives under ambient conditions among CdS-TNTAs, NP-TNTAs and TNTAs counterparts. In another work, our group reported a facile electrostatic self-assembly strategy to achieve the construction of well-defined Aux/NP-TNTAs heterostructures for significantly enhanced photocatalytic reduction of nitro compounds under simulated solar light irradiation as compared to blank NP-TNTAs.152 The glutathione (GSH)-capped Aux clusters used with distinct Highest Occupied Molecular Orbital (HOMO)–Lowest Unoccupied Molecular Orbital (LUMO) gap are able to act like a semiconductor with small bandgap and thereby can be utilized as a novel photosensitizer to extend the optical absorption of 1D TiO2.152 In a following work, our group further reported the preparation of Au nanocrystals and GSH-capped Aux clusters co-modified NP-TNTAs ternary heterostructures via a facile layer-by-layer (LbL) self-assembly strategy based on electrostatic attractive interaction.153 As displayed in Fig. 16B, the hierarchically ordered Aux/Au/NP-TNTAs ternary heterostructures show the optimal photoactivities toward reduction of aromatic nitro compounds under visible light irradiation compared with NP-TNTAs, Au/NP-TNTAs and Aux/NP-TNTAs counterparts, by which the imperative roles of Au NPs as electron relay mediator and plasmonic sensitizer for Aux clusters were ascertained. Fig. 16A shows the schematic illustration of possible electron transfer pathways in Aux/Au/NP-TNTAs ternary system under visible light irradiation, which reveals two main transfer pathways for electrons photoexcited over Aux clusters: (1) direct transfer of photoexcited electrons from Aux clusters to the CB of TiO2; (2) electrons transfer from Aux clusters to the Fermi level of Au NPs and subsequently to the CB of TiO2 in which Au NPs act as electron relay mediator. This work promotes our understanding of photocatalytic mechanism on selective organic transformation over metal cluster/metal nanocrystal/1D TiO2 heterostructured photocatalysts.
 |
| Fig. 16 (A) Schematic illustration of electron transfer pathways in Aux/Au/NP-TNTAs ternary heterostructure. (B) Photocatalytic reduction of (a) 4-NA, (b) 2-NA, (c) 3-NA, (d) 4-nirtrophenol (4-NP), (e) 2-NP, and (f) 3-NP over NP-TNTAs, Au/NP-TNTAs, Aux/NP-TNTAs, and Aux/Au/NP-TNTAs heterostructures under visible light irradiation (λ > 420 nm). (Reprinted with permission from American Chemical Society.)153 | |
5.3 Hydrogen production
With increasing concern over the crisis of global energy, solar-driven photocatalysis using 1D semiconductors to generate clean chemical fuels, such as photocatalytic hydrogen production has been widely investigated as a promising route to meet the increasing worldwide demands for clean energy.146,147,154 Over the past decades, significant progresses have been made in exploring the photocatalytic hydrogen production using 1D TiO2 based composite heterostructures.155–160
Some typical works on photocatalytic water splitting based on 1D TiO2 based composite heterostructures have been extensively reported.155–157 For instance, hierarchical reduced graphene oxide (RGO)–TiO2 nanowire arrays were prepared by Fu's group via an in situ growth process followed by calcination.155 Compared to pure anatase TiO2 and RGO/TiO2 composite prepared by mechanical mixing, the as-prepared hierarchical RGO–TiO2 nanowire arrays showed much more enhanced H2 production rate without using any noble metals as the co-catalysts, which resulted from the synergetic effect of improved separation of electron–hole pairs, increased catalytic active sites and enhanced light absorption afforded by RGO as well as unique hierarchical structure. In another work, Basahel et al., reported in situ self-decoration of Pt/TiO2 nanotubes heterostructures with a small amount of Pt (0.2 at%) using Ti–Pt alloys, which resulted in a remarkable improvement of the photocatalytic hydrogen evolution performance under UV and visible light irradiation.156 It was revealed that Pt nanoparticles (4–5 nm) could affect the electronic structure of TiO2 by constructing a semiconductor/metal heterojunction leading to an efficient photocatalyst for H2 production. Impressively, the Pt/TiO2 nanotubes heterostructures showed a significantly enhanced hydrogen production rate (100.4 mL cm−2 h−1) under UV light illumination in comparison with blank TiO2 nanotubes (18.5 mL cm−2 h−1). More significantly, the hydrogen production amount over Pt/TiO2 nanotubes heterostructures under solar simulated light irradiation reached around 60 times higher than that obtained over blank TiO2 nanotubes. Besides, C3N4/1D TiO2 heterostructures have also been explored for photocatalytic water splitting.157,158 For instance, as shown in Fig. 17, GR-like g-C3N4 nanosheets/N-doped TiO2 nanofibers heterostructures (GCN/NT NFs) with an intimate interface and large specific surface area were fabricated by an electrospinning technique combined with a modified heat-etching method.157 The results showed that GCN/NT NFs possessed much more improved photocatalytic H2 production performance (8931.3 μmol h−1 g−1) as compared with blank TiO2 NFs, N-doped TiO2 NFs and g-C3N4 nanosheets under simulated solar light irradiation, owing predominantly to the synergistic effect between g-C3N4 nanosheets and N-doped TiO2 NFs, which played a vital role in enhancing the light absorption and charge transport.
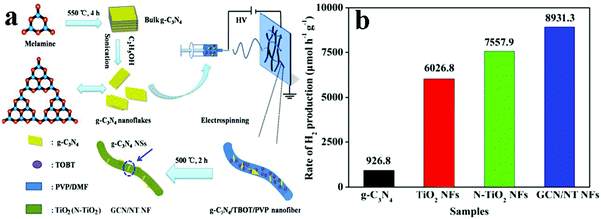 |
| Fig. 17 (a) Schematic illustration of the fabrication of GCN/NT NFs and (b) photocatalytic H2 production rate of TiO2 NFs, g-C3N4 and GCN/NT NFs under simulated solar light irradiation. (Reprinted with permission from Springer.)157 | |
5.4 CO2 reduction
Photocatalytic conversion of CO2 into chemical fuels has been ascertained as a promising way to achieve solar energy conversion. Particularly, photoreduction of CO2 using 1D TiO2 based composite nanostructures has aroused increasing interests in recent years for solar fuel production.159,160 For example, Pratim et al., synthesized highly efficient Pt–TiO2 films composed of columnar TiO2 decorated with ultrafine Pt NPs (0.5–2 nm) via gas-phase deposition method for CO2 photoreduction to methane.159 As shown in Fig. 18, methane (CH4) yield reached a peak value of 1361 μmol per g-cat per h with a quantum yield of ca. 2.41%. Synergistic effects of high specific surface area, minimized charge barriers by oriented single-phase crystallinity of the film, and efficient separation of electron–hole pairs afforded by Pt NPs are considered to be the integrated reason for high photocatalytic performance. In addition, as displayed in Fig. 19, Shankar's group prepared the ternary core–shell heterostructures with coaxial Cu–Pt NPs supported periodically on modulated double-walled TiO2 nanotube arrays (Cu–Pt/PMTiNTs) for efficient conversion of CO2 to light hydrocarbons under sunlight irradiation, such as CH4, C2H4, and C2H6.160 To probe the role of Cu–Pt bimetallic coatings in the ternary heterostructures, a collection of Cu–Pt/PMTiNTs catalysts with different ratios of Cu/Pt were prepared. It was found that Cu0.33Pt0.67/PMTiNTs heterostructure was the most efficient catalyst to produce light hydrocarbons (610 nmol cm−2 h−1) at ambient temperature using low-concentration CO2.
 |
| Fig. 18 (a) Yields of CH4 and CO on a Pt–TiO2 NHs film vs. irradiation time, (b) yields of CO and CH4 over P25, pristine TiO2 film, and Pt–TiO2 NHs films with different Pt deposition times and (c) schematic diagram of CO2 photoreduction mechanism over Pt–TiO2 NHs films. (Reprinted with permission from American Chemical Society.)159 | |
 |
| Fig. 19 (A) FESEM image, (B) high-resolution Cu 2p and (C) high-resolution Pt 4f spectra of Cu–Pt/PMTiNT. (D) Cross-sectional FESEM and EDX mapping of Cu–Pt/PMTiNTs. (E) Schematic illustration of solar-driven CO2 conversion mechanism. (Reprinted with permission from John Wiley & Sons, Inc.)160 | |
5.5 Photocatalysis across the full solar spectrum
With continuous efforts over the past few decades, various strategies as mentioned above have been developed to expand the light absorption range of 1D TiO2 nanostructures to maximally utilize solar energy. However, it is still challenging to extend the light absorption to the near-infrared (NIR) region.
Recently, 1D TiO2 based composite photocatalysts have been reported by Liu's group.161,162 Utilizing TiO2 nanobelts as the substrate, Bi2WO6/TiO2 nanobelts hybrid heterostructures have been prepared and utilized as photocatalysts.161 The results indicated that the as-prepared catalysts can harness UV, visible, and near-infrared light to degrade organic contaminants (e.g., MO) in aqueous solution, suggesting the great application potential as a versatile photoresponsive material for waste-water cleanup.161 As clearly shown in Fig. 20, Bi2WO6 nanosheets demonstrate good visible-light-driven photoactivities and even good photocatalytic performances toward degradation of MO under near-infrared light irradiation owing to the formation of oxygen vacancies on Bi2WO6 which raises the Fermi level and reduces the band edge, thus allowing for inter-band transitions and carrier creation under near-infrared excitation. Moreover, Bi2WO6/TiO2 nanobelt heterostructures exhibited substantially enhanced UV-vis-NIR photocatalytic activities compared with P25, TiO2 nanobelts, and Bi2WO6 nanosheets due to the synergistic interaction of Bi2WO6 and TiO2. Based on the above results, combining with the photocatalytic property of TiO2 nanobelts under UV light irradiation, the authors concluded that the UV-vis-NIR multi-band responsive hybrid heterostructures could be synthesized by assembling Bi2WO6 nanosheets on TiO2 nanobelts, as shown in Fig. 20b and c. In a following work, the same group reported the preparation of carbon quantum dots (CQDs) sensitized hydrogenated TiO2 nanobelt (CQDs/H-TiO2) heterostructures for full solar spectrum photocatalysis under UV, visible and near-infrared irradiation.162 Notably, the hydrogenated TiO2 nanobelts (H-TiO2) created oxygen vacancies and Ti3+ ions, thereby extending the light absorption spectrum of TiO2 to the visible region. More importantly, CQDs possess both up- and down-converted photoluminescence, electron-accepting and -transporting properties, which renders CQDs-based hybrid photocatalysts promising agents for harvesting NIR light. Compared with blank H-TiO2 and TiO2 nanobelts, composite heterostructures showed significantly improved photoactivities toward MO degradation and water splitting under UV, visible and NIR light irradiation, as shown in Fig. 21.
 |
| Fig. 20 (a) Photocatalytic degradation of MO over Bi2WO6 nanosheets under UV light, visible light, and NIR light irradiation (b and c) TEM images of Bi2WO6/TiO2 nanobelt heterostructures. Photocatalytic degradation of MO over P25, TiO2 nanobelts, Bi2WO6 nanosheets and Bi2WO6/TiO2 nanobelt heterostructures under (d) UV light, (e) visible light, (f) NIR light and (g) simulated solar light irradiation. (h) DRS results of TiO2 nanobelts, Bi2WO6 nanosheets and Bi2WO6/TiO2 nanobelts heterostructures. (i) Visible and NIR absorption spectra of TiO2 nanobelts, Bi2WO6 nanosheets, and Bi2WO6/TiO2 nanobelts heterostructures. (Reprinted with permission from John Wiley & Sons, Inc.)161 | |
 |
| Fig. 21 Photocatalytic degradation of MO over P25, TiO2 nanobelts, H-TiO2 nanobelts and CQDs/H-TiO2 heterojunctions under (a) UV, (b) visible, and (c) NIR light irradiation; (d) photocatalytic hydrogen production of loaded TiO2 nanobelts, H-TiO2 nanobelts, and CQDs/H-TiO2 heterojunctions with 1 wt% Pt loading amount under the irradiation of Xe arc lamp (300 W); and (e) schematic illustration for photocatalytic mechanism of CQDs/H-TiO2 heterojunctions under UV, visible and NIR light irradiation. (Reprinted with permission from Elsevier.)162 | |
Besides CQDs, NIR light absorption has also been observed in several other materials, such as up-conversion materials, gold cluster, PbS, and Cu2(OH)PO4.163–166 For a typical example, Tatsuma's group found that Au25 clusters sensitized TiO2 electrodes exhibited anodic photocurrent and negative shifts of photo-potential in response to visible and NIR light for the conversion of light to electricity.164 In another work, Qin et al., found that YF3:Yb3+@Tm3+/TiO2 core–shell heterostructure could effectively decompose MB under the irradiation of NIR light due to the up-conversion mechanism.165 Alternatively, Chalita and other researchers synthesized PbS QDs/TNTs heterostructures for photoreleasing nitric oxide and producing singlet oxygen under NIR light irradiation.166 All of the above works provide new insights for the rational construction of 1D TiO2 based composite heterostructures as efficient photocatalysts under wide spectrum of solar light for solar energy conversion.
6. Summary and perspectives
In summary, this review article systematically summarized the recent developments of 1D TiO2 based composite heterostructures, including metal/1D TiO2, semiconductor/1D TiO2, complex compound/1D TiO2, polymer/1D TiO2, and 1D TiO2 based multiphase heterostructures. Moreover, versatile photocatalytic applications of these 1D TiO2 based composite heterostructures including nonselective photocatalytic degradation of organic dye pollutants, photocatalytic selective organic transformation, hydrogen production, and CO2 reduction have been elucidated.
Notably, 1D TiO2 based composite heterostructures not only retain the excellent properties of 1D TiO2 nanostructures, but also inherit the properties of the secondary phase materials, which can significantly enhance the photocatalytic performances and broaden the light harvesting capability. The factors of aspect ratio, doping level, and surface states have shown pronounced effects on the photoactivities of 1D TiO2 based composite heterostructures. All of these factors should be integrally considered to design more efficient 1D TiO2 composite heterostructures. Supported by these fundamental understandings, the promising opportunities and developments of 1D TiO2 composite heterostructures should be particularly highlighted.
Firstly, rational fabrication of 1D TiO2 based spatially ordered core–shell nanosystems for photocatalytic or photoelectrochemical (PEC) applications may represent a promising future direction. The architectures can be mainly classified into two categories: (1) 1D nanostructures grown on the trunk of 1D TiO2 nanostructures forming hierarchically ordered 1D/1D branched hybrid nanostructures;141 (2) 2D or 3D units grown on 1D TiO2 nanostructures forming spatially ordered nanoarchitectures.126,127
Secondly, constructing well-organized 1D TiO2 based multi-component heterostructures which demonstrate “Z-scheme” mechanism in photocatalytic or PEC systems might be another promising direction.167,168 Z-scheme mechanism dominated photocatalytic or PEC systems have been established to build up unique pathways for tunable charge transfer via rational design of well-defined 1D TiO2 based hybrid architectures.
Despite the progresses made in previous works, great potential of 1D TiO2 based composite heterostructures in heterogeneous photocatalysis and photoelectrocatalysis has not been fully exploited. With rational exploration of the electronic structures of 1D TiO2 nanostructures, forming 1D TiO2 based hybrid heterostructures is an emerging and viable route to increase photogenerated charge separation and transfer. It is anticipated that our review article could provide useful information on precisely controllable synthesis of a large variety of 1D TiO2 based composite heterostructures for extensive photocatalytic and PEC applications.
Acknowledgements
This work was supported by the Nanyang Technological University startup grant: M4080977.120, Singapore Ministry of Education Academic Research Fund (AcRF) Tier 1: M4011021.120 and Public Sector Funding from Agency for Science, Technology and Research of Singapore (A*Star): M4070232.120.
References
- J. Schneider, M. Matsuoka, M. Takeuchi, J. Zhang, Y. Horiuchi, M. Anpo and D. W. Bahnemann, Chem. Rev., 2014, 114, 9919–9986 CrossRef CAS PubMed.
- L. Sang, Y. Zhao and C. Burda, Chem. Rev., 2014, 114, 9283–9318 CrossRef CAS PubMed.
- K. Lee, A. Mazare and P. Schmuki, Chem. Rev., 2014, 114, 9385–9454 CrossRef CAS PubMed.
- X. Wang, Z. Li, J. Shi and Y. Yu, Chem. Rev., 2014, 114, 9346–9384 CrossRef CAS PubMed.
- M. Dahl, Y. Liu and Y. Yin, Chem. Rev., 2014, 114, 9853–9889 CrossRef CAS PubMed.
- J. Zhang, F.-X. Xiao, G. Xiao and B. Liu, Appl. Catal., A, 2016, 521, 50–56 CrossRef CAS.
- F.-X. Xiao, J. Miao and B. Liu, J. Am. Chem. Soc., 2014, 136, 1559–1569 CrossRef CAS PubMed.
- F.-X. Xiao, J. Miao and B. Liu, Mater. Horiz., 2014, 1, 259–263 RSC.
- L. Liu and X. Chen, Chem. Rev., 2014, 114, 9890–9918 CrossRef CAS PubMed.
- G. Liu, H. G. Yang, J. Pan, Y. Q. Yang, G. Q. Lu (Max) and H.-M. Cheng, Chem. Rev., 2014, 114, 9559–9612 CrossRef CAS PubMed.
- B. Liu and E. S. Aydil, J. Am. Chem. Soc., 2009, 131, 3985–3990 CrossRef CAS PubMed.
- F.-X. Xiao, Z. Zeng, S.-H. Hsu, S.-F. Hung, H.-M. Chen and B. Liu, ACS Appl. Mater. Interfaces, 2015, 7, 28105–28109 Search PubMed.
- F.-X. Xiao, M. Paqliaro, Y.-J. Xu and B. Liu, Chem. Soc. Rev., 2016, 45, 3088–3121 RSC.
- G. Wang, H. Wang, Y. Ling, Y. Tang, X. Yang, R. C. Fitzmorris, C. Wang, J. Z. Zhang and Y. Li, Nano Lett., 2011, 11, 3026–3033 CrossRef CAS PubMed.
- L. Li, X. Qin, G. Wang, L. Qi, G. Du and Z. Hu, Appl. Surf. Sci., 2011, 257, 8006–8012 CrossRef CAS.
- J. Lee, Y. Lee, H. Song, D. Jang and Y. Choa, Curr. Appl. Phys., 2011, 11, S210–S214 CrossRef.
- P. Hoyer, Langmuir, 1996, 12, 1411–1413 CrossRef CAS.
- N. Liu, X. Chen, J. Zhang and J. W. Schwank, Catal. Today, 2014, 225, 34–51 CrossRef CAS.
- D. V. Bavykin, J. M. Friedrich and F. C. Walsh, Adv. Mater., 2006, 18, 2807–2824 CrossRef CAS.
- Z. L. He, W. X. Que, J. Chen, X. T. Yin, Y. C. He and J. B. Ren, ACS Appl. Mater. Interfaces, 2012, 4, 6815–6825 Search PubMed.
- A. Hu, X. Zhang, K. D. Oakes, P. Peng, Y. N. Zhou and M. R. Servos, J. Hazard. Mater., 2011, 189, 278–285 CrossRef CAS PubMed.
- N. T. Q. Hoa, Z. Lee, S.-H. Kang, V. Radmilovic and E.-T. Kim, Appl. Phys. Lett., 2008, 92, 122508 CrossRef.
- R. Marschall, Adv. Funct. Mater., 2014, 24, 2421–2440 CrossRef CAS.
- M. Wang, J. Ioccozia, L. Sun, C. Lin and Z. Lin, Energy Environ. Sci., 2014, 7, 2182–2202 CAS.
- J. L. Xie, C. X. Guo and C. M. Li, Energy Environ. Sci., 2014, 7, 2559–2579 CAS.
- B. Weng, S. Liu, Z.-R. Tang and Y.-J. Xu, RSC Adv., 2014, 4, 12685–12700 RSC.
- S. Liu, Z.-R. Tang, Y. Sun, J. C. Colmenares and Y.-J. Xu, Chem. Soc. Rev., 2015, 44, 5053–5075 RSC.
- R. K. Joshi and J. J. Schneider, Chem. Soc. Rev., 2012, 41, 5285–5312 RSC.
- M. Law, L. E. Greene, J. C. Johnson, R. Saykally and P. Yang, Nat. Mater., 2005, 4, 455–459 CrossRef CAS PubMed.
- J. Tang, Z. Huo, S. Brittman, H. Gao and P. Yang, Nat. Nanotechnol., 2011, 6, 568–572 CrossRef CAS PubMed.
- S. Sotiropoulou, Y. Sierra-Sastre, S. S. Mark and C. A. Batt, Chem. Mater., 2008, 20, 821–834 CrossRef CAS.
- R. G. Hobbs, N. Petkov and J. D. Holmes, Chem. Mater., 2012, 24, 1975–1991 CrossRef CAS.
- C. Pan, Z. Luo, C. Xu, J. Luo, R. Liang, G. Zhu, W. Wu, W. Guo, X. Yan, J. Xu, Z. L. Wang and J. Zhu, ACS Nano, 2011, 5, 6629–6636 CrossRef CAS PubMed.
- J.-P. Colinge, C.-W. Lee, A. Afzalian, N. D. Akhavan, R. Yan, I. Ferain, P. Razavi, B. O'Neill, A. Blake, M. White, A.-M. Kelleher, B. McCarthy and R. Murphy, Nat. Nanotechnol., 2010, 5, 225–229 CrossRef CAS PubMed.
- P. P. Naulleau, C. N. Anderson, L.-M. Baclea-an, P. Denham, S. George, K. A. Goldberg, M. Goldstein, B. Hoef, G. Jones, C. Koh, B. La Fontaine, W. Montgomery and T. Wallow, J. Vac. Sci. Technol., B, 2009, 27, 2911–2915 CAS.
- J. B. Antony, B. B. Chris, P. W. Gwyn and V. Yuli, J. Phys. D: Appl. Phys., 2003, 36, 2471 CrossRef.
- D. Winston, V. R. Manfrinato, S. M. Nicaise, L. L. Cheong, H. Duan, D. Ferranti, J. Marshman, S. McVey, L. Stern, J. Notte and K. K. Berggren, Nano Lett., 2011, 11, 4343–4347 CrossRef CAS PubMed.
- I. Sychugov, Y. Nakayama and K. Mitsuishi, Nanotechnology, 2010, 21, 285307 CrossRef CAS PubMed.
- E. A. Costner, M. W. Lin, W.-L. Jen and C. G. Willson, Annu. Rev. Mater. Res., 2009, 39, 155–180 CrossRef CAS.
- G. Scappucci, G. Capellini, B. Johnston, W. M. Klesse, J. A. Miwa and M. Y. Simmons, Nano Lett., 2011, 11, 2272–2279 CrossRef CAS PubMed.
- Y. Xia, P. Yang, Y. Sun, Y. Wu, B. Mayers, B. Gates, Y. Yin, F. Kim and H. Yan, Adv. Mater., 2003, 15, 353–389 CrossRef CAS.
- H. Zhang, Y. Liu, D. Yao and B. Yang, Chem. Soc. Rev., 2012, 41, 6066–6088 RSC.
- D. Mijatovic, J. C. T. Eijkel and A. van den Berg, Lab Chip, 2005, 5, 492–500 RSC.
- T. Kasuga, M. Hiramatsu, A. Hoson, T. Sekino and K. Niihara, Langmuir, 1998, 14, 3160–3163 CrossRef CAS.
- D. V. Bavykin, J. M. Friedrich and F. C. Walsh, Adv. Mater., 2006, 18, 2807–2824 CrossRef CAS.
- Y. Q. Wang, G. Q. Hu, X. F. Duan, H. L. Sun and Q. K. Xue, Chem. Phys. Lett., 2002, 365, 427–431 CrossRef CAS.
- S. Lee, C. Jeon and Y. Park, Chem. Mater., 2004, 16, 4292–4295 CrossRef CAS.
- J. J. Qiu, W. D. Yu, X. D. Gao and X. M. Li, Nanotechnology, 2006, 17, 4695–4698 CrossRef CAS PubMed.
- D. Gong, C. A. Grimes, O. K. Varghese, W. Hu, R. S. Singh, Z. Chen and E. C. Dickey, J. Mater. Res., 2001, 16, 3331–3334 CrossRef CAS.
- G. K. Mor, K. Shankar, M. Paulose, O. K. Varghese and C. A. Grimes, Nano Lett., 2005, 5, 191–195 CrossRef CAS PubMed.
- A. R. Armstrong, G. Armstrong, J. Canales, R. Garcia and P. G Bruce, Adv. Mater., 2005, 7, 862–865 CrossRef.
- J. N. Nian and H. S. Teng, J. Phys. Chem. B, 2006, 110, 4193–4198 CrossRef CAS PubMed.
- M. Wei, Y. Konishi, H. Zhou, H. Sugihara and H. Arakawa, Chem. Phys. Lett., 2004, 400, 231–234 CrossRef CAS.
- Y. Lin, G. S. Wu, X. Y. Yuan, T. Xie and L. D. Zhang, J. Phys.: Condens. Matter, 2003, 15, 2917–2922 CrossRef CAS.
- B. Xiang, Y. Zhang, Z. Wang, X. H. Luo, Y. W. Zhu, H. Z. Zhang and D. P. Yu, J. Phys. D: Appl. Phys., 2005, 38, 1152–1155 CrossRef CAS.
- Y. Lei, L. D. Zhang and J. C. Fan, Chem. Phys. Lett., 2001, 338, 231–236 CrossRef CAS.
- S. Liu and K. Huang, Sol. Energy Mater. Sol. Cells, 2004, 85, 125–131 Search PubMed.
- B. Wen, C. Liu and Y. Liu, Inorg. Chem., 2005, 44, 6503–6505 CrossRef CAS PubMed.
- B. Wen, C. Liu and Y. Liu, J. Phys. Chem. B, 2005, 109, 12372–12375 CrossRef CAS PubMed.
- L. Miao, S. Tanemura, S. Toh, K. Kaneko and M. J. Tanemura, J. Cryst. Growth, 2004, 264, 246–252 CrossRef CAS.
- L. Miao, S. Tanemura, S. Toh, K. Kaneko and M. Tanemura, Appl. Surf. Sci., 2004, 238, 175–179 CrossRef CAS.
- J. M. Wu, J. Cryst. Growth, 2004, 269, 347–355 CrossRef CAS.
- J. M. Wu, T. W. Zhang, Y. W. Zeng, S. Hayakawa, K. Tsuru and A. Osaka, Langmuir, 2005, 21, 6995–7002 CrossRef CAS PubMed.
- J. M. Wu and T. W. Zhang, J. Photochem. Photobiol., A, 2004, 162, 171–177 CrossRef CAS.
- P. D. Cozzoli, E. Fanizza, M. L. Curri, D. Laub and A. Agostiano, Chem. Commun., 2005, 942–944 RSC.
- R. Buonsanti, V. Grillo, E. Carlino, C. Giannini, M. L. Curri, C. Innocenti, C. Sangregorio, K. Achterhold, F. G. Parak, A. Agostiano and P. D. Cozzoli, J. Am. Chem. Soc., 2006, 128, 16953–16970 CrossRef CAS PubMed.
- S. K. Pradhan, P. J. Reucroft, F. Yang and A. Dozier, J. Cryst. Growth, 2003, 256, 83–88 CrossRef CAS.
- G. Ma, X. Zhao and J. Zhu, Int. J. Mod. Phys. B, 2005, 19, 2763–2768 CrossRef CAS.
- Z. Zhao, J. Tian, Y. Sang, A. Cabot and H. Liu, Adv. Mater., 2015, 27, 2557–2582 CrossRef CAS PubMed.
- T. D. Nguyen-Phan, M. B. Song, E. J. Kim and E. W. Shin, Res. Chem. Intermed., 2010, 36, 613 CrossRef CAS.
- J. Wang, L. Zhao, V. S.-Y. Lin and Z. Lin, J. Mater. Chem., 2009, 19, 3682–3687 RSC.
- N. T. Q. Hoa and E.-T. Kim, Electrochem. Solid-State Lett., 2008, 11, K1–K3 CrossRef CAS.
- Y. Wang, R. Wang, C. Guo, J. Miao, Y. Tian, T. Ren and Q. Liu, Nanoscale, 2012, 4, 1545 RSC.
- J. W. Jung, C. L. Lee, S. Yu and I. D. Kim, J. Mater. Chem. A, 2016, 4, 703–750 CAS.
- M. Tian, G. Wu and A. Chen, ACS Catal., 2012, 2, 425–432 CrossRef CAS.
- K. Liu, Y. Bi, S. Qu, F. Tan, D. Chi, S. Lu, Y. Li, Y. Kou and Z. Wang, Nanoscale, 2014, 6, 6180–6186 RSC.
- X. Zhang, Y. Liu, S.-T. Lee, S. Yang and Z. Kang, Energy Environ. Sci., 2014, 7, 1409–1419 CAS.
- F. Xiao, Chem. Commun., 2012, 48, 6538–6540 RSC.
- F. Xiao, J. Phys. Chem. C, 2012, 116, 16487–16498 CAS.
- Q. Lu, Z. Lu, Y. Lu, L. Lv, Y. Ning, H. Yu, Y. Hou and Y. Yin, Nano Lett., 2013, 13, 5698–5702 CrossRef CAS PubMed.
- S.-F. Hung, F.-X. Xiao, Y.-Y. Hsu, N.-T. Suen, H.-B. Yang, H.-M. Chen and B. Liu, Adv. Energy Mater., 2016, 6, 1501339 CrossRef.
- J. Li, S. K. Cushing, P. Zheng, T. Senty, F. Meng, A. D. Bristow, A. Manivannan and N. Wu, J. Am. Chem. Soc., 2014, 136, 8438–8449 CrossRef CAS PubMed.
- N. Li, G. Liu, C. Zhen, F. Li, L. Zhang and H. M. Cheng, Adv. Funct. Mater., 2011, 21, 1717–1722 CrossRef CAS.
- G. Williams, B. Seger and P. V. Kamat, ACS Nano, 2008, 2, 1487–1491 CrossRef CAS PubMed.
- H. Zhang, X. Lv, Y. Li, Y. Wang and J. Li, ACS Nano, 2010, 4, 380–386 CrossRef CAS PubMed.
- M.-Z. Ge, S.-H. Li, J.-Y. Huang, K.-Q. Zhang, S. S. Al-Deyab and Y.-K. Lai, J. Mater. Chem. A, 2015, 3, 3491–3499 CAS.
- J. Xian, D. Li, J. Chen, X. Li, M. He, Y. Shao, L. Yu and J. Fang, J. Mater. Chem. A, 2014, 2, 5187–5192 CAS.
- Y. Sang, Z. Zhao, J. Tian, P. Hao, H. Jiang, H. Liu and J. P. Claverie, Small, 2014, 10, 3775–3782 CrossRef CAS PubMed.
- M.-M. Titirici, R. J. White, N. Brun, V. L. Budarin, D. S. Su, F. Monte, J. H. Clark and M. J. MacLachlan, Chem. Soc. Rev., 2015, 44, 250–290 RSC.
- Z. R. Hesabi, N. K. Allam, K. Dahmen, H. Garmestani and M. A. El-Sayed, ACS Appl. Mater. Interfaces, 2011, 3, 952–955 CAS.
- S. Sadhu and P. Poddar, J. Phys. Chem. C, 2014, 118, 19363–19373 CAS.
- W. Guo, C. Xu, X. Wang, W. S. Wang, C. Pan, C. Lin and Z. L. Wang, J. Am. Chem. Soc., 2012, 134, 4437–4441 CrossRef CAS PubMed.
- L. Jaesang, D. F. John, B. H. Joseph and K. Jae-Hong, Environ. Sci. Technol., 2007, 41, 2529–2535 CrossRef.
- Y. Long, Y. Lu, Y. Huang, Y. Peng, Y. Lu, S.-Z. Kang and J. Mu, J. Phys. Chem. C, 2009, 113, 13899–13905 CAS.
- X. T. Zheng, A. Ananthanarayanan, K. Q. Luo and P. Chen, Small, 2015, 11, 1620–1636 CrossRef CAS PubMed.
- B. K. Gupta, G. Kedawat, Y. Agrawal, P. Kumar, J. Dwivedi and S. K. Dhawan, RSC Adv., 2015, 5, 10623–10631 RSC.
- D. Pan, C. Xi, Z. Li, L. Wang, Z. Chen, B. Lu and M. Wu, J. Mater. Chem. A, 2013, 1, 3551–3555 CAS.
- H. Wang, L. Zhang, Z. Chen, J. Hu, S. Li, Z. Wang, J. Liu and X. Wang, Chem. Soc. Rev., 2014, 43, 5234–5244 RSC.
- W. Zhou, H. Liu, J. Wang, D. Liu, G. Du, S. Han, J. Lin and R. Wang, Phys. Chem. Chem. Phys., 2010, 12, 15119–15123 RSC.
- Z. Wu, Y. Wang, L. Sun, Y. Mao, M. Wang and C. Lin, J. Mater. Chem. A, 2014, 2, 8223–8229 CAS.
- Q. Huang, F. Kang, H. Liu, Q. Li and X. Xiao, J. Mater. Chem. A, 2013, 1, 2418–2425 CAS.
- K. Pan, Y. Dong, W. Zhou, Q. Pan, Y. Xie, T. Xie, G. Tian and G. Wang, ACS Appl. Mater. Interfaces, 2013, 5, 8314–8320 CAS.
- N. Wang, C. Sun, Y. Zhao, S. Zhou, P. Chen and L. Jiang, J. Mater. Chem., 2008, 18, 3909–3911 RSC.
- G. Chen, S. Ji, Y. Sang, S. Chang, Y. Wang, P. Hao, J. Claverie, H. Liu and G. Yu, Nanoscale, 2015, 7, 3117–3125 RSC.
- L. Jia, J. Xie, C. Guo and C. M. Li, RSC Adv., 2015, 5, 62611–62618 RSC.
- M. Ge, C. Cao, S. Li, S. Zhang, S. Deng, J. Huang, Q. Li, K. Zhang, S. S. Al-Deyab and Y. Lai, Nanoscale, 2015, 7, 11552–11560 RSC.
- M. Guo, L. Li, H. Lin, Y. Zuo, X. Huang and G. Li, Chem. Commun., 2013, 49, 11752–11754 RSC.
- M. Wang, L. Sun, Z. Lin, J. Cai, K. Xie and C. Lin, Energy Environ. Sci., 2013, 6, 1211–1220 CAS.
- K. R. Reyes-Gil and D. B. Robinson, ACS Appl. Mater. Interfaces, 2013, 5, 12400–12410 CAS.
- B. Lu, X. Li, T. Wang, E. Xie and Z. Xu, J. Mater. Chem. A, 2013, 1, 3900–3906 CAS.
- F.-X. Xiao, J. Miao, H. Y. Wang, H. Yang, J. Chen and B. Liu, Nanoscale, 2014, 6, 6727–6737 RSC.
- P. Lv, W. Fu, H. Yang, H. Sun, Y. Chen, J. Ma, X. Zhou, L. Tian, W. Zhang, M. Li, H. Yao and D. Wu, CrystEngComm, 2013, 15, 7548–7555 RSC.
- B. A. Gonfa, M. R. Kim, N. Delegan, A. C. Tavares, R. Izquierdo, N. Wu, M. A. E. Khakani and D. Ma, Nanoscale, 2015, 7, 10039–10049 RSC.
- W. Zhou, Z. Yin, Y. Du, X. Huang, Z. Zeng, Z. Fan, H. Liu, J. Wang and H. Zhang, Small, 2013, 9, 140–147 CrossRef CAS PubMed.
- W. Zhou, Z. Yin, Y. Du, X. Huang, Z. Zeng, Z. Fan, H. Liu, J. Wang and H. Zhang, RSC Adv., 2014, 4, 7838–7844 RSC.
- F. Cai, F. Yang, Y. Zhang, C. Ke, C. Cheng, Y. Zhao and G. Yan, Phys. Chem. Chem. Phys., 2014, 16, 23967–23974 RSC.
- Y. Li, S. Luo, Z. Wei, D. Meng, M. Ding and C. Liu, Phys. Chem. Chem. Phys., 2014, 16, 4361–4368 RSC.
- C. Meng, Z. Liu, T. Zhang and J. Zhai, Green Chem., 2015, 17, 2764–2768 RSC.
- M. Shang, W. Wang, L. Zhang, S. Sun, L. Wang and L. Zhou, J. Phys. Chem. C, 2009, 113, 14727–14731 CAS.
- R. Liu, P. Hu and S. Chen, Appl. Surf. Sci., 2012, 258, 9805–9809 CrossRef CAS.
- L. Chen, S. Li, Z. Liu, Y. Lu, D. Wang, Y. Lin and T. Xie, Phys. Chem. Chem. Phys., 2013, 15, 14262–14269 RSC.
- H. Liu, Y. Chen, G. Tian, Z. Ren, C. Tian and H. Fu, Langmuir, 2015, 31, 5962–5969 CrossRef CAS PubMed.
- Z. Zhao, J. Tian, D. Wang, Y. Sang, H. Liu, J. Wang, S. Chen, R. I. Boughton and H. Jiang, J. Mater. Chem., 2012, 22, 23395–23403 RSC.
- T. Cao, Y. Li, C. Wang, C. Shao and Y. Liu, Langmuir, 2011, 27, 2946–2952 CrossRef CAS PubMed.
- W.-Q. Fan, X.-Q. Yu, S.-Y. Song, H.-Ye. Bai, C. Zhang, D. Yan, C.-B. Liu, Q. Wang and W.-D. Shi, CrystEngComm, 2014, 16, 820–825 RSC.
- Q. Liu, H. Lu, Z. Shi, F. Wu, J. Guo, K. Deng and L. Li, ACS Appl. Mater. Interfaces, 2014, 6, 17200–17207 CAS.
- Z. Liu, K. Guo, J. Han, Y. Li, T. Cui, B. Wang, J. Ya and C. Zhou, Small, 2014, 10, 3153–3161 CrossRef CAS PubMed.
- S. Yang, X. Cui, J. Gong and Y. Deng, Chem. Commun., 2013, 49, 4676–4678 RSC.
- E. Ngaboyamahina, C. Debiemme-Chouvy, A. Pailleret and E. M. M. Sutter, J. Phys. Chem. C, 2014, 118, 26341–26350 CAS.
- M. Wei, J. Wan, Z. Hu, B. Wang and Z. Peng, RSC Adv., 2015, 5, 58184–58190 RSC.
- Z. Liu, X. Zhang, S. Nishimoto, M. Jin, D. A. Tryk, M. Taketoshi and A. Fujishima, Langmuir, 2007, 23, 10916–10919 CrossRef CAS PubMed.
- S. Shen, X. Wang, T. Chen, Z. Feng and C. Li, J. Phys. Chem. C, 2014, 118, 12661–12668 CAS.
- H. Zhuang, J. Miao, H. Huang, J. Long, Y. Zhang, H. Yang, S. He, Y. Yang, X. Wang and B. Liu, ChemPhysChem, 2015, 16, 1352–1355 CrossRef CAS PubMed.
- P. Yan, X. Wang, X. Zheng, R. Li, J. Han, J. Shi, A. Li, Y. Gan and C. Li, Nano Energy, 2015, 15, 406–412 CrossRef CAS.
- C. Wang, X. Zhang, Y. Wei, L. Kong, F. Chang, H. Zheng, L. Wu, J. Zhi and Y. Liu, Dalton Trans., 2015, 44, 13331–13339 RSC.
- W. Zhou, L. Gai, P. Hu, J. Cui, X. Liu, D. Wang, G. Li, H. Jiang, D. Liu, H. Liu and J. Wang, CrystEngComm, 2011, 13, 6643–6649 RSC.
- B. Liu, A. Khare and E. S. Aydil, ACS Appl. Mater. Interfaces, 2011, 3, 4444–4450 CAS.
- L. Pan, H. Huang, C. K. Lim, Q. Y. Hong, M. S. Tse and O. K. Tan, RSC Adv., 2013, 3, 3566–3571 RSC.
- Y.-C. Pu, Y. Ling, K.-D. Chang, C.-M. Liu, J. Z. Zhang, Y.-J. Hsu and Y. Li, J. Phys. Chem. C, 2014, 118, 15086–15094 CAS.
- F. Xiao, ACS Appl. Mater. Interfaces, 2012, 4, 7055–7063 CAS.
- F.-X. Xiao, S. F. Hung, H. B. Tao, J. Miao, H. B. Yang and B. Liu, Nanoscale, 2014, 6, 14950–14961 RSC.
- P. Sudhagar, A. Devadoss, T. Song, P. Lakshmipathiraj, H. Han, V. V. Lysak, C. Terashima, K. Nakata, A. Fujishima, U. Paik and Y. S. Kang, Phys. Chem. Chem. Phys., 2014, 16, 17748–17755 RSC.
- W. Zhou, Y. Guan, D. Wang, X. Zhang, D. Liu, H. Jiang, J. Wang, X. Liu, H. Liu and S. Chen, Chem. – Asian J., 2014, 9, 1648–1654 CrossRef CAS PubMed.
- J. Zhang, F.-X. Xiao, G. Xiao and B. Liu, Nanoscale, 2014, 6, 11293–11302 RSC.
- Y. Zhang, N. Zhang, Z.-R. Tang and Y.-J. Xu, ACS Nano, 2012, 6, 9777–9789 CrossRef CAS PubMed.
- N. Zhang, S. Liu and Y.-J. Xu, Nanoscale, 2012, 4, 2227–2238 RSC.
- N. Zhang, Y. Zhang and Y.-J. Xu, Nanoscale, 2012, 4, 5792–5813 RSC.
- T. Jiang, C. Jia, L. Zhang, S. He, Y. Sang, H. Li, Y. Li, X. Xu and H. Liu, Nanoscale, 2015, 7, 209–217 RSC.
- J. T. Jiang, C. Jia, L. Zhang, S. He, Y. Sang, H. Li, Y. Li, X. Xu and H. Liu, J. Mater. Chem. A, 2014, 2, 16292–16298 Search PubMed.
- N. Qin, Y. Liu, W. Wu, L. Shen, X. Chen, Z. Li and L. Wu, Langmuir, 2015, 31, 1203–1209 CrossRef CAS PubMed.
- F.-X. Xiao, J. Miao, H.-Y. Wang and B. Liu, J. Mater. Chem. A, 2013, 1, 12229–12238 CAS.
- F.-X. Xiao, J. Miao, H. B. Tao, S. F. Hung, H.-Y. Wang, H. B. Yang, J. Chen, R. Chen and B. Liu, Small, 2015, 11, 2115–2131 CrossRef CAS PubMed.
- F.-X. Xiao, Z. Zeng and B. Liu, J. Am. Chem. Soc., 2015, 137, 10735–10744 CrossRef CAS PubMed.
- F.-X. Xiao, S.-F. Hung, J. Miao, H.-Y. Hsin, H. B. Yang and B. Liu, Small, 2015, 11, 554–567 CrossRef CAS PubMed.
- X. Cao, G. Tian, Y. Chen, J. Zhou, W. Zhou, C. Tian and H. Fu, J. Mater. Chem. A, 2014, 2, 4366–4374 CAS.
- S. N. Basahel, K. Lee, R. Hahn, P. Schmuki, S. M. Bawaked and S. A. Al-Thabaiti, Chem. Commun., 2014, 50, 6123–6125 RSC.
- C. Han, Y. Wang, Y. Lei, B. Wang, N. Wu, Q. Shi and Q. Li, Nano Res., 2015, 8, 1199–1209 CrossRef CAS.
- M. Yang, J. Liu, X. Zhang, S. Qiao, H. Huang, Y. Liu and Z. Kang, Phys. Chem. Chem. Phys., 2015, 17, 17887–17893 RSC.
- W. N. Wang, W. J. An, B. Ramalingam, S. Mukherjee, D. M. Niedzwiedzki, S. Gangopadhyay and P. Biswas, J. Am. Chem. Soc., 2012, 134, 11276–11281 CrossRef CAS PubMed.
- X. Zhang, F. Han, B. Shi, S. Farsinezhad, G. P. Dechaine and K. Shankar, Angew. Chem., Int. Ed., 2012, 51, 12732–12735 CrossRef CAS PubMed.
- J. Tian, Y. Sang, G. Yu, H. Jiang, X. Mu and H. Liu, Adv. Mater., 2013, 25, 5075–5080 CrossRef CAS PubMed.
- J. Tian, Y. Leng, Z. Zhao, Y. Xia, Y. Song, P. Hao, J. Zhan, M. Li and H. Liu, Nano Energy, 2015, 11, 419–427 CrossRef CAS.
- G. Wang, B. Huang, X. Ma, Z. Wang, X. Qin, X. Zhang, Y. Dai and M.-H. Whangbo, Angew. Chem., Int. Ed., 2013, 52, 1–5 CrossRef.
- N. Sakai and T. Tatsuma, Adv. Mater., 2010, 22, 3185–3188 CrossRef CAS PubMed.
- C. Ratanatawanate, A. Chyao and K. Balkus, J. Am. Chem. Soc., 2011, 133, 3492–3497 CrossRef CAS PubMed.
- W. Qin, D. Zhang, D. Zhao, L. Wang and K. Zheng, Chem. Commun., 2010, 46, 2304–2306 RSC.
- W. Liao, M. Murugananthan and Y. Zhang, Phys. Chem. Chem. Phys., 2015, 17, 8877–8884 RSC.
- C. Liu, J. Tang, H. M. Chen, B. Liu and P. Yang, Nano Lett., 2013, 13, 2989–2992 CrossRef CAS PubMed.
|
This journal is © the Partner Organisations 2017 |