DOI:
10.1039/C6QI00428H
(Research Article)
Inorg. Chem. Front., 2017,
4, 183-190
Solid-state synthesis of Nd-doped glass: thermal collapse of Nd3+-incorporated NaY zeolites†
Received
12th October 2016
, Accepted 10th November 2016
First published on 11th November 2016
Abstract
A solid-state synthesis of Nd-doped glass (NDG) in the acquisition aspect from the thermal collapse of Nd3+ ion-exchanged NaY zeolites has been conducted through experiments. The idea of rational design of the “perfect” NDG has been proven to be conceptually and practically feasible. The solid-synthesis method in comparison with the traditional molten-salt method has potential advantages of achieving “perfect” distribution, i.e., Nd3+ can be well-dispersed in the zeolite precursor and the final solid-state glass. The phase transformation from the Nd3+-exchanged zeolite NaY to Nd-doped glass was characterized by X-ray diffraction (XRD), scanning electron microscopy (SEM) and differential scanning calorimetry (DSC), describing the migration and constraint of Nd3+ ions in the solid-state synthesis process. The fluorescence properties of the solid-state synthesized Nd-doped glass were also examined, and the results show that there is an optimized Nd3+ concentration associated with effective photoluminescence. The strategy of the solid-state process is possible to be applied to the synthesis of other functional glassy materials.
Introduction
In recent years, glasses have been widely used as phosphor materials because of their significant flexibility in size and shape, good optical properties, transparency and their capability of being doped with considerable amounts of metal ions with high uniformity.1 Nd-Doped glass (NDG), which combines the intrinsic properties of glass and the unique features of the neodymium element, has been considered as one of the most attractive optical materials for use in laser systems,2 fiber amplifiers,3 light emitting diodes,4 plasma display panels, electroluminescent devices,5etc.
Traditionally, NDG was synthesized based on the molten salt method.6,7 However, the conventional molten salt process consumes a lot of energy resources, sometimes involving tedious treatments. More importantly, it has an intrinsic difficulty in controlling the dispersive states of Nd3+ cations in the product.8 Therefore, more efficient methods for the preparation of NDG materials in which Nd3+ cations are well-dispersed are in high demand. Nowadays, a new strategy of fabricating silicate glass via a solid-state synthesis route is proposed and developed,9i.e., collapsing some zeolites into glasses by thermal treatment or high pressure.10,11 However, research in this field mainly focused on the computational aspect,12–16 such as the molecular dynamics study of the zeolite collapse.13,14 It has recently been discovered that the solid-state synthesis method has potential in producing glassy materials with short range order and low structural entropy, namely “perfect glass”.17,18 This concept provides a new opportunity of fabricating NDG in which Nd3+ cations are well “perfectly” distributed. It is expected theoretically that the optimized distribution could minimize the self-quenching of photoluminescence (PL).19
In order to investigate the feasibility of the solid-state route of making NDG, Nd3+-incorporated zeolites need to be prepared as precursor materials.20 As host materials, various metal ions and organic guest molecules could be implanted in the channels or the cages of zeolites.21–24 Rare earth cations, such as Nd3+ and La3+, have been ion-exchanged into a framework to functionalize zeolites.25–29 We followed the commonly used methods30 for implementing Nd3+ cations in the NaY zeolite to prepare our precursor material which was denoted as “Nd-NaY”.
For the sake of controlling rationally the solid-state synthesis process, key issues, such as the distribution and migration of Nd3+ cations in the zeolite, and the structural response of Nd-NaY to the thermal treatment, need to be addressed. Previous studies on the rare earth ion-exchanged zeolite Y using X-ray diffraction (XRD) and nuclear magnetic resonance (NMR) reveal that La3+ cations locate in the supercages of zeolite Y when the temperature is below 373 K, but migrate to sodalite cages after the samples are annealed at above 773 K, where Na+ cations transport oppositely from the sodalite cages to the supercages.31 Considering the similarity of Nd3+ and La3+ in their physical and chemical natures, it is expected that Nd3+ behaves in the same manner with La3+ in zeolite Y under heat treatment.32
Herein, we report on the solid-state synthesis of NDG via the collapse of Nd-NaY zeolites. Different from the method of computational simulation, the solid-state synthesis of an NDG product in the acquisition aspect is conducted through experiments. The idea of rational design of the “perfect” NDG has been proven to be conceptually and practically feasible. The following efforts towards the solid-state synthesis of NDG were made: first, Nd3+-incorporated NaY (Nd-NaY) zeolite was prepared via ion-exchange. Then, the NDG product was obtained through the collapse of the Nd-NaY zeolite. The collapse process of the Nd-NaY zeolite was recorded by powder X-ray diffraction (XRD), Scanning Electron Microscopy (SEM) and Differential Scanning Calorimetry (DSC). Based on the solid-state synthesis of NDG, we investigate the photoluminescence (PL) properties of the NDG product. This work proves that it is not only conceptually possible but also practically feasible to synthesize NDG using the solid-state method from an Nd-NaY precursor. It is a simple and feasible route to prepare the NDG product. The strategy of the solid-state process is also possible to be applied to the synthesis of other functional glassy materials.
Experimental
Reagents and materials
Neodymium nitrate hexahydrate (A. R.) was purchased from Sinopharm Chemical Reagent Co., Ltd. The gases Ar (>99.997%) and O2 (>99.999%) were supplied by Shanghai Chengkung Gas Co., Ltd. Zeolite NaY (Si/Al molar ratio = 2.46, the molar composition is NaAlSi2.46O6.92), a type of hydrate faujasite, was prepared hydrothermally by following a procedure described in the literature.33
Preparation of the Nd-NaY zeolites
Conventional Nd3+-doped NaY zeolites were prepared under magnetic stirring at room temperature in the Nd(NO3)3 solution with different Nd3+ concentrations. 1.0 g of NaY zeolite was dispersed into 60 ml of Nd(NO3)3 aqueous solution in which the concentrations of Nd3+ cations are 0, 0.00004, 0.004, 0.04, 0.012, 0.02, 0.04, 0.2, and 0.4 mol L−1. The obtained ion-exchanged NaY zeolites are denoted as samples II, III, IV, V, VI, VII, VIII, IX, and X, respectively. The pure NaY zeolite raw material without ion exchange is denoted as sample I. After being stirred for 24 hours, ion-exchanged NaY zeolites were filtered, washed with deionized water and dried in an oven at 80 °C for 6 hours. All the samples are then calcined at 773 K for 6 hours, to make the Nd3+ cations migrate from the supercages into the sodalite cages.
Preparation of Nd-doped glasses
Further calcinations were carried out for the Nd-NaY zeolite samples at higher temperatures between 1000 K and 1300 K to obtain the Nd-doped glassy state materials via solid-state phase transformation. The as-made Nd-NaY zeolites were heated in a hot-pressing furnace at the corresponding temperature for 6 hours. The heating speed was kept at 5 °C min−1. By this thermal-treatment process, the Nd-doped glassy products were obtained.
Characterization
The powder X-ray diffraction (XRD) patterns were recorded on a Rigaku D/Max 2550 X-ray diffractometer with Cu Kα radiation (λ = 1.5418 Å). Scanning electron microscopy (SEM) images were recorded on a Hitachi S-4800 field-emission scanning electron microscope at an acceleration voltage of 5.0 kV. Differential scanning calorimetry (DSC) measurements were performed on a Perkin-Elmer DSC8000 (PerkinElmer, Inc., USA) at a scan rate of 5 °C min−1. Nitrogen adsorption/desorption measurements were performed on an ASAP 2020 Accelerated Surface Area and Porosimetry system (Micromeritics Inc., USA). The emission spectra were recorded on a TRIAX 550 fluorescence spectrometer. The Nd3+ fluorescence decay time was measured by exciting the samples with a laser diode. The 29Si MAS NMR measurements were performed on a Varian Infinity plus 400 NMR spectrometer. The chemical composition of the samples was determined by inductively coupled plasma atomic emission spectroscopy (ICP-AES, Perkin Elmer 3300DV, Perkin Elmer, USA).
Results and discussion
Previous studies had reported the feasibility of the solid-state route for making “perfect glass” based on the thermal collapse of zeolites,9,34 which provides a new opportunity of fabricating NDG. A proposed preparation procedure for the solid-state synthesis of NDG is illustrated in Scheme 1. First, the Nd-NaY zeolite was prepared via ion-exchange. The NDG product was then obtained through the thermal collapse of the Nd-NaY zeolite. Towards this solid-state synthesis of NDG, the priority of research is in investigating the feasibility of the structure and thermal stability of the Nd-NaY zeolite precursor.
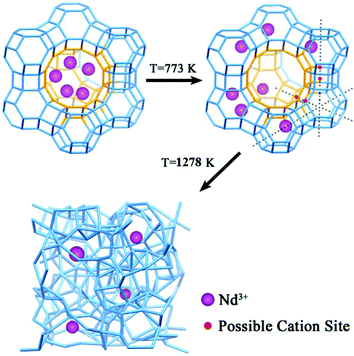 |
| Scheme 1 Schematic representation of the preparation process for the NDG product. | |
The Nd-NaY samples were prepared by introducing Nd3+ cations into the cages of zeolite Y via ion exchange.35 The analysis results of Nd-NaY samples with different Nd3+ concentrations measured by inductively coupled plasma (ICP) are listed in Table 1. As shown in Table 1, there is a saturation of ion exchange between Nd3+ and Na+ cations. For the samples from III to X, the atom ratios of Nd3+ cations in ion-exchanged Nd-NaY zeolites are 0.002, 0.023, 0.244, 0.768, 1.277, 2.450, 2.480 and 2.450 mol%, corresponding to 0.009, 0.089, 0.842, 2.770, 4.637, 8.628, 9.097 and 8.628 Nd3+ cations per sodalite cage, respectively. Obviously, the ion exchange has already reached saturation within the sample VIII. This means one sodalite cage of NaY zeolites can accommodate up to approximately 9 Nd3+ cations. The composition of sample VIII is approximately Nd0.26Na0.22AlSi2.46O6.92 based on the ICP elemental analysis (Table S1, ESI†), revealing that the maximum amount of Nd3+ cations in the Nd-NaY zeolite via ion-exchange can reach as high as 15 wt%.
Table 1 The Nd3+cation concentrations of Nd-NaY zeolites and their corresponding molar ratios
Sample |
Nd3+ solution (mol L−1) |
Nd molar ratio by ICP (mol%) |
Nd3+ counts per sodalite cage |
Nd3+ counts per unit cell |
I |
N/A |
0.000 |
0.000 |
0.000 |
II |
0 |
0.000 |
0.000 |
0.000 |
III |
0.00004 |
0.002 |
0.009 |
0.014 |
IV |
0.0004 |
0.023 |
0.089 |
0.149 |
V |
0.004 |
0.244 |
0.842 |
1.403 |
VI |
0.012 |
0.768 |
2.770 |
4.617 |
VII |
0.02 |
1.277 |
4.637 |
7.728 |
VIII |
0.04 |
2.450 |
8.628 |
14.380 |
IX |
0.2 |
2.480 |
9.097 |
14.556 |
The ion-exchange procedure mainly involves the exchange between the Nd3+ cations and Na+ cations of the NaY zeolite. Fig. 1a shows that with the increase of Nd3+ concentration in the solution, the molar percentage of Na+ in the ion-exchanged products decreases in a chemically equivalent manner. There is no significant change in the Si4+ and Al3+ concentration observed in our experiments as reported in the literature.36,37Fig. 1b shows the relationship between the change of the concentration of the Nd element (ΔNd) in the Nd-NaY samples and that of the Na element (ΔNa). Its gradient is approximately −1/3 as shown in Fig. 1b, which indicates that the cations are exchanged in a stoichiometric ratio. According to the literature,38–40 rare earth cations prefer to occupy the space of supercages after being ion-exchanged into the framework at 373 K.
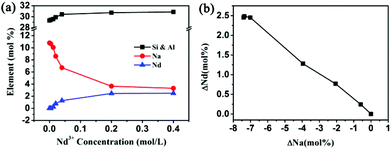 |
| Fig. 1 (a) The molar percentage change of each element versus the concentration of Nd3+ in the Nd(NO3)3 solution. (b) The change of Nd elemental concentration versus the change of Na elemental concentration in the Nd-NaY zeolite. The elemental composition of ion-exchanged samples was measured by ICP. | |
Structural description of Nd-NaY zeolites
The depiction about the lattice planes of zeolites corresponds to their main XRD peaks. As shown in Fig. 2a, the main peaks located at 6.2°, 15.6°, 23.6°, 26.9°, and 31.4° can be readily indexed to the (111), (331), (533), (642), and (555) diffractions, respectively, of the crystalline NaY zeolite (JCPDS no. 39-1380). In order to investigate the structural change of zeolite NaY, the samples I, II, V and VIII, and their corresponding samples after being heated up to 1073 K (denoted as “sample ID-T”) were collected and characterized by XRD patterns. As shown in Fig. 2b, none of the corresponding aggregates of neodymium oxide or metal salt impurities was detected in the XRD patterns of these Nd3+-exchanged NaY zeolites. For sample VIII (there are about 9 Nd3+ cations per sodalite cage), the main XRD peaks apart from (111) are much weaker than other three samples with lower Nd3+ concentrations, indicating significant distortion of lattice planes associated with the sodalite cages. It has been reported that rare earth cations in NaY zeolites undergo an irreversible migration from supercages to sodalite cages under thermal treatment at 773 K.32 Based on this migration, it is presumable that most of the Nd3+ cations occupy the preferred sites associated with the sodalite cages after calcination at 773 K. As shown in Fig. 2c, after being heated at 1073 K for 6 hours, the phase of the pure NaY zeolite became amorphous while the Nd-doped NaY samples containing Nd3+ cations were still in the crystalline state. When Nd3+ cations occupy the sodalite cages of the NaY zeolite, these Nd3+ cations play a role of a space-filling agent within the zeolites,32 resulting in a structural integrity of the NaY zeolites. Thus we can draw a conclusion that Nd-incorporation could enhance the thermal stability of the NaY zeolite.
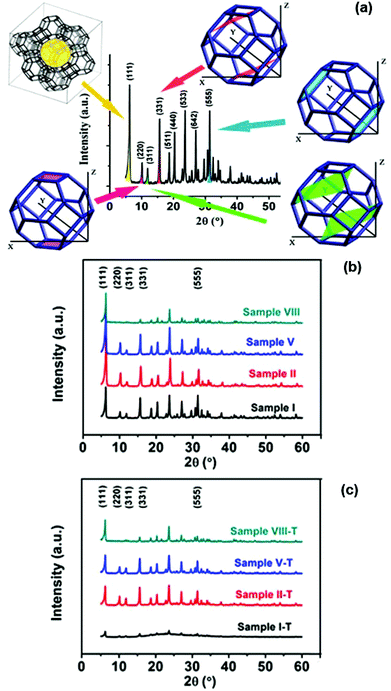 |
| Fig. 2 XRD patterns of NaY zeolites and their corresponding lattice planes (a). XRD patterns of the Nd-NaY zeolites with different Nd3+ concentrations (b), and their corresponding samples after calcinations at 1073 K (T) for 6 hours (c). | |
Thermal stability of Nd-NaY zeolites
Based on the discussion above, the Nd3+ ion-exchanged NaY samples exhibit better thermal stability than the pure NaY zeolite. In this section, the thermal stability of the Nd-NaY samples is further investigated using SEM and DSC techniques.
The feasibility of the solid-state route for making glassy materials based on the thermal collapse of zeolites is firstly tested and verified. There is a typical glass transition observed in the thermal collapse of the NaY zeolite (Fig. S1, ESI†), which proves that the final product obtained by the solid-state synthesis is in the glassy state. This phase transformation from a crystalline material into amorphous glass could also be confirmed by XRD patterns (Fig. S2, ESI†).
To further investigate the thermal stability, the samples for the pure NaY zeolite and Nd-NaY zeolite were compared with the SEM images (Fig. S3, ESI†). The morphologies of the NaY zeolite and the Nd-NaY zeolite are similar before the heating treatment. However, after the samples are heated at 1073 K, the grain shape of the pure NaY zeolite changed more significantly than that of the Nd-NaY sample. The addition of Nd3+ cations obviously leads to an increase of the thermal stability of the zeolite. The SEM images of thermal collapse processes for the Nd-doped NaY sample are shown in Fig. 3. Tiny particles are still observed after calcinations at 773 K (Fig. 3a). When heated at 1073 K, these small particles can agglomerate together to form larger clusters (Fig. 3b). Thermal collapse of the Nd-NaY sample is present with the temperature elevated. When the temperature was over 1200 K, the rate of thermal collapse quickened. As shown in the SEM images of Fig. 3c–f, the thermal collapse of the Nd-NaY zeolites is based on the evolution of the microstructure, the edges of the neighbouring grains merged at 1203 K, and then joined together to form a coral-like structure at 1243 K, and eventually grew into bulk over 1283 K. This phase transformation can also be observed from the photographs (Fig. S4, ESI†). With the elevation of collapse temperature, the existence of the Nd-NaY sample gradually changed from tiny powder to a transparent bulk. Thus we can speculate that the solid-state synthesis of NDG is a cumulative process, and the suitable temperature range should be controlled from 1100 K to 1300 K. The nitrogen adsorption/desorption measurements of the Nd-NaY zeolite and its collapse NDG product were carried out on a Micromeritics ASAP 2020 instrument at 77 K (Fig. S5, ESI†). The Brunauer–Emmett–Teller (BET) specific surface area of the Nd-NaY zeolite is 571 m2 g−1, but the specific surface area of the NDG product sharply decreases to 0.2 m2 g−1, indicating that the zeolite precursor has collapsed and shrinked into an amorphous glass product.
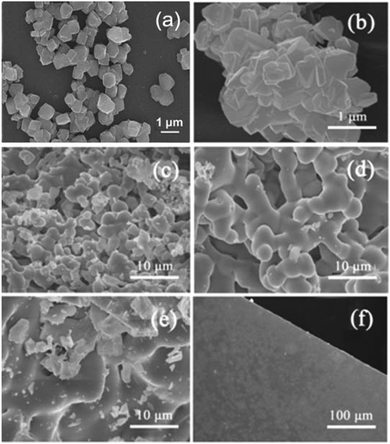 |
| Fig. 3 SEM images of Nd-NaY zeolites (sample VIII) after being calcined at 773, 1073, 1203, 1223, 1243, and 1283 K for 6 hours, respectively. | |
As for the amorphization, i.e., collapse of the Nd-doped zeolites happens in the temperature range of 1100 K to 1300 K, further investigations in this paper mainly focus on this temperature range. Systematic DSC measurements were carried out on the Nd-NaY zeolites containing different concentrations of Nd3+ cations, which are utilized to investigate their thermal response. Fig. 4a summarizes the DSC curves corresponding to all of the samples as indicated in the figure legends. As shown in Fig. 4a, a distinct broadened peak located at 1203 K is observed for the pure NaY zeolite. With the introduction of Nd3+ cations, the collapse peak shifts slowly to higher temperature positions. When the Nd3+ counts are approximately 0.01, 0.8, 2.7, 4.6, and 9 Nd3+ cations per sodalite cage, the exothermic peak of phase transformation shifts to high temperature zones at 1221 K, 1226 K, 1232 K, 1250 K and 1278 K, respectively, indicating that their collapse temperature has increased with the increase of Nd3+ concentration.41 From this point of view, Nd3+-incorporation can enhance the thermal stability of NaY zeolites, which is consistent with the above XRD results. Moreover, the full width at half maximum (FWHM) of the exothermic peaks become sharper with the increase of Nd3+ concentration, and consequently, the collapse temperature of the samples also increased, also revealing that the impact of the Nd3+ cations on the structure stability is cumulative. Thus FWHM can be regarded as an indicator to investigate the structure stability of the samples under thermal treatment. The collapse enthalpy indicators measured by integrating each collapse peak increase with respect to the addition of Nd3+ cations as shown in Fig. 4b. Obviously, the introduction of Nd3+ cations into the zeolites would increase the collapse temperature. The Nd3+ cations occupy the space of the framework cages and make the zeolites more thermally stable.
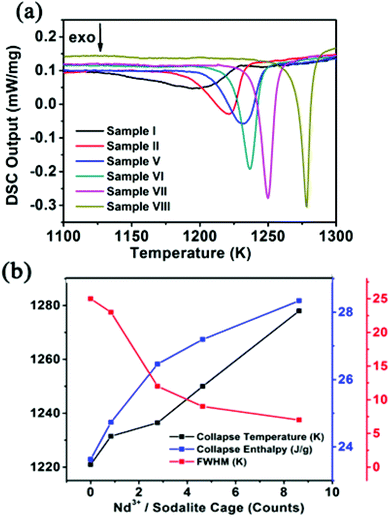 |
| Fig. 4 (a) DSC upscans of various samples carried out under an Ar atmosphere from 1100 K to 1300 K, (b) collapse temperature, enthalpy and FWHM extracted from DSC curves with respect to Nd3+ concentration. | |
Thermal stability of the pre-pressed Nd-NaY zeolites
It is debated that the locating sites of Nd3+ in the zeolite Y framework should have influences on the thermal stability. However, in the above DSC experiments, all the samples were calcined at 773 K before the measurements were carried out, in which Nd3+ cations had migrated into the sodalite cages. It is expected that the pre-treatment with pressure could break the channels in which Nd3+ cations transfer from the supercages to the sodalite cages. Once the channels of the Nd-NaY zeolite were broken, the Nd3+ cations would be constrained in the supercages. Thus NDG in which Nd3+ cations are well distributed is expected to be fabricated. Herein DSC investigations were carried out to compare the thermal stability between the Nd-NaY samples with and without pre-treatment with pressure, i.e., Nd3+ cations in the sodalite cages or in the supercages.
Fig. 5a shows the DSC results of the Na-NaY zeolite (sample VIII) at a scan rate (5 °C min−1) after being preheated at 773 K for 20 minutes, which gives more time for Nd3+ migration if possible. As shown by the black (solid and dashed) lines in Fig. 5a, the collapse temperatures of the Nd-NaY zeolite with and without calcination (respectively corresponding to the solid and dashed black lines) under a pressure of 0 GPa are identical, which implies that the scan rate of 5 °C min−1 is slow enough for Nd3+ cations transporting to their favourite locations. However, as shown by the red (solid and dashed) lines in Fig. 5a, for the same pair of samples, both pre-treated under a pressure of 0.5 GPa, the collapse temperature of the calcined sample (Nd3+ cations are in the sodalite cages) is 1230 K, while the collapse temperature of the uncalcined sample (Nd3+ cations are in supercages) is only 1218 K. Obviously, the collapse temperature of the sample with Nd3+ cations located in the sodalite cages is 12 K higher than that of the sample with Nd3+ cations located in supercages. The same trend is found for the samples pre-treated under a pressure of 1.0 GPa and 1.5 GPa. Under the pressure of 1.0 GPa and 1.5 GPa, the collapse temperature of the sample with Nd3+ cations located in the supercages is also lower than that of the sample with Nd3+ cations located in the sodalite cages, which is consistent with the results under the pressure of 0.5 GPa. From the above analysis, it suggests that the sodalite cage has more thermal stability than the supercage. The collapse enthalpy of the samples which was measured by integrating each collapse peak is shown in Fig. 5b. From Fig. 5b, it is found that the pre-treatment under different pressures would reduce the collapse temperature. With the increase of the pressure, the collapse temperature would shift to the low temperature zone. Moreover, it is found that the FWHM of the DSC curves becomes much broader with the increase of the treatment pressure. In this way under the different treatment pressures, NDG with Nd3+ cations constrained in sodalite cages or supercages would expect to be fabricated.
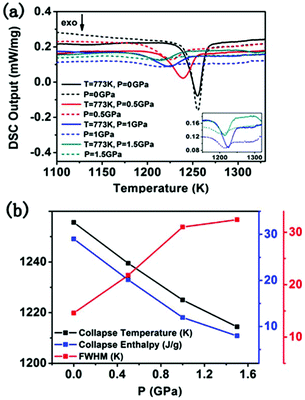 |
| Fig. 5 (a) DSC upscans for sample VIII pre-treated under different conditions. (b) The collapse temperature, enthalpy and FWHM extracted from DSC curves. The inset is the magnified part of DSC. | |
Luminescence effect of solid-state synthesized NDG
Photoluminescence (PL) property is one of the key features of NDG materials. It is known that strong PL can be limited by the following three factors: (i) the low transparency of the materials, (ii) deactivation attributed to H2O and –OH in the vicinity of Nd3+ cations,42,43 and (iii) clustering of Nd3+ cations in the materials.19 The strongest PL excitation of NDG is at a wavelength of 582 nm, which is in the visible light region, the factor (i) can be overcome by controlling the transparency of the glass. Water molecules absorbed by the zeolite can be removed by preheating the raw materials before the solid-state synthesis, and then the influence of the factor (ii) could also be eliminated. Furthermore, rational control over the temperature of calcinations of the glass forming process could result in the migration of most Nd3+ cations from supercages to sodalite cages where their clustering is not feasible.
Both the emission and excitation spectra of the NDG with different Nd3+ concentrations were recorded, as shown in Fig. 6a and b. It can be seen that all of the NDG samples give rise to emission and excitation spectra at the same wavelength but with variation in the peak intensities. This uniformity of luminescence spectra suggests that both emission and excitation exhibit structural similarities for the NDG product with different Nd3+ concentrations. The 4F3/2–4I11/2 transition intensity was plotted as a function of the Nd3+ cation doping concentration (Fig. 6c). As shown in Fig. 6c, a parabolic curve of the photoluminescence intensity was observed with the increase of Nd3+ concentration. It can be seen that the emission intensities increase sharply with respect to the Nd3+ concentration from 0 to 0.84 Nd3+ cations per sodalite cage. However, when Nd3+ cations per sodalite cage are beyond 0.84, the photoluminescence would decrease rapidly. The decay time of the PL emission also has a similar trend. Among all the NDG samples, a lifetime longer than 400 μs was observed in the sample V in which the Nd3+ concentration is 0.84 Nd3+ cations per sodalite cage (Fig. 6c). Below or beyond this concentration, the lifetime of DNG would decrease rapidly. As per the above discussion, it is already known that the clustering of Nd3+ cations is another important factor which influences the PL intensity. Strong PL emission (the luminescent 4F3/2–4I11/2 transition at 1066 nm), which is excited by light of wavelength 582 nm, needs Nd3+ cations to be dispersed homogeneously without the formation of clusters. Once the Nd3+ cations form the cluster, adjacent Nd3+ cations would lead to a self-quenching process.19 Thus we believe that the solid-state synthesis is a new route for the formation of NDG with the advantages of rationally controlling the positions of Nd3+ in the precursor as well as the final product. The well dispersed and isolated Nd3+ cations can avoid self-quenching and give rise to strong and stable PL emission. Based on the highest PL intensity, the ideal rational design of the “perfect” NDG is about one Nd3+ cation per sodalite cage, which is equivalent to the molar percentage of Nd3+ cations which is 0.24 within the Nd-NaY zeolites. According to the ICP elemental analysis, the composition of the zeolite sample V is Nd0.03Na0.91AlSi2.46O6.92, indicating that the amount of Nd3+ cations in the zeolite is 1.86 wt% (Table S1, ESI†). This means that 1.86 wt% of Nd ions doped in the zeolite NaY is found to be the optimized amount of raw material required for fabricating NDG with the best performance. According to Fig. 6c, it can be concluded that the rational design of NDG has been successful. The solid-state synthesized NDG from the Nd-NaY zeolite with about one Nd3+ cation per sodalite cage does give the optimized performance in PL among the samples. It is a simple and feasible route to prepare an NDG product in which Nd3+ cations are well “perfectly” distributed.
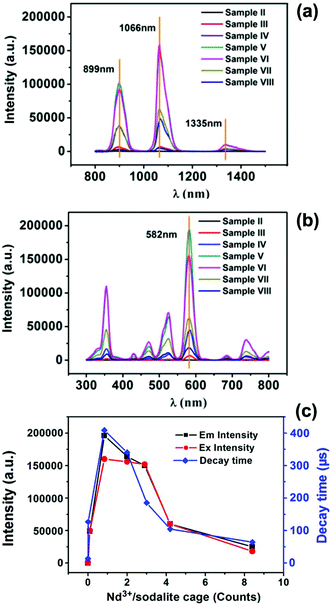 |
| Fig. 6 (a) PL emission spectra excited at 582 nm wavelength, (b) PL excitation spectra for the 1066 nm emission, (c) emission spectral intensity (at 1066 nm), excitation spectral intensity (at 582 nm) and decay time with respect to the counts of Nd3+ per sodalite cage. | |
Conclusions
The solid-state synthesis of the NDG product in the acquisition aspect from the thermal collapse of Nd3+ ion-exchanged NaY zeolites has been conducted through experiments. In order to prepare structurally optimized NDG, sufficient calcination is necessary for the purpose of promoting the migration of Nd3+ cations from supercages to sodalite cages. The addition of Nd3+ cations could enhance the thermal stability of NaY zeolites. When the Nd3+ concentration is about 0.24 in molar percentage, equivalently one cation per sodalite cage, the Nd-NaY zeolite is found to be the optimized raw material for fabricating NDG with the best performance. DSC studies reveal that the amorphization temperature of the Nd-NaY zeolites depends on the Nd3+ concentration and its locations in the zeolite framework. With the increase of the Nd3+ concentration from zero to a saturated molar percentage of about 2.5 (about 9 Nd3+ cations per sodalite cage), pre-calcined (at 773 K for 6 hours) Nd-NaY samples exhibit a rising collapse temperature from 1203 K to 1278 K. For the zeolite structure, sodalite cages are more suitable than supercages for constraining the guest Nd3+ cations in terms of dispersion.
The idea of rational design of the “perfect” NDG has been proven to be conceptually and practically feasible for the first time. This work proves that it is not only conceptually possible but also practically feasible to synthesize NDG using the solid-state method from an Nd-NaY precursor. It is a simple and feasible route to prepare the NDG product. The strategy of the solid-state process is also possible to be applied to the synthesis of other functional glassy materials.
Acknowledgements
This work was supported by the Shanghai Committee of Science and Technology (13ZR1429300), the Shanghai Rising-star Program (B-type) (no.15QB1402300), the Shanghai International Science and Technology Cooperation Project (no. 14520720500), and the Minhang District Project (2016MH195).
Notes and references
- P. Chimalawong, J. Kaewkhao, C. Kedkaew and P. Limsuwan, Optical and electronic polarizability investigation of Nd3+-doped sodalime silicate glasses, J. Phys. Chem., 2010, 71, 965–970 CAS.
- J. H. Campbell and T. I. Suratwala, Nd-doped phosphate glasses for high-energy/high-peak-power lasers, J. Non-Cryst. Solids, 2000, 263–264, 318–341 CrossRef.
- R. K. Brow, The structure of simple phosphate galsses, J. Non-Cryst. Solids, 2000, 263–264, 1–28 CrossRef.
- T. Som and B. Karmakar, Green and red fluorescence upconversion in neodymium-doped phonon antimony glasses, J. Alloys Compd., 2009, 476, 383–389 CrossRef CAS.
- M. H. V. Werts, R. H. Woudenberg, P. G. Emmerink, R. V. Gassel, J. W. Hofstaat and J. W. Verhoeven, A near-infrared luminescent label based on YbIII ions and its application in a fluoroimmunoassay, Angew. Chem., Int. Ed., 2009, 39, 4542–4544 CrossRef.
- L. A. B. Pilkington, The float glass process, Proc. R. Soc. London, Ser. A, 1969, 314, 1–25 CrossRef CAS.
- P. F. Becher, S. B. Waters, C. G. Westmoreland and L. Riester, Compositional effects on the properties of Si-Al-Re-based oxynitride glasses (RE=La, Nd, Gd, Y, or Lu), J. Am. Ceram. Soc., 2002, 85, 897–902 CrossRef CAS.
- G. N. Greaves and S. Sen, Inorganic glasses, glass-forming liquids and amorphizing solids, Adv. Phys., 2007, 56, 1–166 CrossRef CAS.
- G. N. Greaves, F. Meneau, O. Majerus, D. G. Jones and J. Taylor, Identifying vibrations that destabilize crystals and characterize the glassy state, Science, 2005, 308, 1299–1302 CrossRef CAS PubMed.
- H. J. Lee, Y. M. Kim, O. S. Kweon and I. J. Kim, Structural and morphological transformation of NaX zeolite crystals at high temperature, J. Eur. Ceram. Soc., 2007, 27, 561–564 CrossRef CAS.
- S. Belkhiri, M. Guerza, S. Chouikh, Y. Boucheffa, Z. Mekhalif, J. Delhalle and C. Colella, Textural and structural effects of heat treatment and γ-irradiation on Cs-exchanged NaX zeolite, bentonite and their mixtures, Microporous Mesoporous Mater., 2012, 161, 115–122 CrossRef CAS.
- K. T. Thomson, R. M. Wentzcovitch, A. McCormick and H. T. Davis, A density functional study of sodalite: a new view on an old system, Chem. Phys. Lett., 1998, 283, 39–43 CrossRef CAS.
- A. B. Mukhopadhyay, C. Oligschleger and M. Dolg, Molecular dynamics investigation of structural properties of a zeolite ZSM-5 based amorphous material, Phys. Rev. B: Condens. Matter, 2003, 67 Search PubMed.
- K. L. Joshi and A. C. T. van Duin, Molecular dynamics study on the Influence of additives on the high-temperature structural and acidic properties of ZSM-5 zeolite, Energy Fuels, 2013, 27, 4481–4488 CrossRef CAS.
- M. O. Coppens, A. T. Bell and A. K. Chakraborty, Effect of topology and molecular occupancy on self-diffusion in lattice models of zeolites - Monte-Carlo simulations, Chem. Eng. Sci., 1998, 53, 2053–2061 CrossRef CAS.
- L. Benco, T. Demuth, J. Hafner, E. Hutschka and H. Toulhoat, Linear hydrocarbons adsorbed in the acid zeolite gmelinite at 700 K ab initio molecular dynamics simulation of hexane and hexane, J. Catal., 2002, 205, 147–156 CrossRef CAS.
- G. N. Greaves and F. Meneau, Probing the dynamics of instability in zeolitic materials, J. Phys.: Condens. Matter, 2004, 16, 345972 CrossRef.
- G. N. Greaves, F. Meneau, F. Kargl, D. Ward, P. Holliman and F. Albergamo, Zeolite collapse and polyamorphism, J. Phys.: Condens. Matter, 2007, 19, 415102 CrossRef.
- A. I. Burshtein, Concentration self-quenching, Sov. Phys. JETP, 1983, 57, 1165–1171 Search PubMed.
- C. S. Cundy and P. A. Cox, The hydrothermal synthesis of zeolites: Precursors, intermediates and reaction mechanism, Microporous Mesoporous Mater., 2005, 82, 1–78 CrossRef CAS.
- J. C. Scaiano and H. Garcia, Intrazeolite photochemistry: Toward supramolecular control of molecular photochemistry, Acc. Chem. Res., 1999, 32, 783–793 CrossRef CAS.
- A. K. Cheetham, G. Ferey and T. Loiseau, Open-framework inorganic materials, Angew. Chem., Int. Ed., 1999, 38, 3268–3292 CrossRef CAS.
- J. Wahlen, D. De Vos, S. De Hertogh, V. Nardello, J. M. Aubry, P. Alsters and P. Jacobs, Lanthanum-exchanged zeolites as active and selective catalysts for the generation of singlet oxygen from hydrogen peroxide, Chem. Commun., 2005, 927–929 RSC.
- X. F. Zhou, Application of zeolite-encapsulated Cu (II) H-4 salen derived from H-2 salen in oxidative delignification of pulp, RSC Adv., 2014, 4, 28029–28035 RSC.
- M. Ryo, Y. Wada, T. Okubo, T. Nakazawa, Y. Hasegawa and S. Yanagida, Spectroscopic study on strongly luminescent Nd(III) exchanged zeolite: TMA(+)-containing FAU type zeolite as a suitable host for ship-in-bottle synthesis, J. Mater. Chem., 2002, 12, 1748–1753 RSC.
- N. N. Wang, Y. Wang, H. F. Cheng, Z. Tao, J. Wang and W. Z. Wu, Impact of cationic lanthanum species on zeolite Y: an infrared, excess infrared and Raman spectroscopic study, RSC Adv., 2013, 3, 20237–20245 RSC.
- S. M. F. Vilela, D. Ananias, A. C. Gomes, A. A. Valente, L. D. Carlos, J. A. S. Cavaleiro, J. Rocha, J. P. C. Tome and F. A. Almeida Paz, Multi-functional metal-organic frameworks assembled from a tripodal organic linker, J. Mater. Chem., 2012, 22, 18354–18371 RSC.
- F. Schuessler, E. A. Pidko, R. Kolvenbach, C. Sievers, E. J. M. Hensen, R. A. van Santen and J. A. Lercher, Nature and location of cationic lanthanum species in high alumina containing faujasite type zeolites, J. Phys. Chem. C, 2011, 115, 21763–21776 CAS.
- M. Ryo, Y. Wada, T. Okubo, Y. Hasegawa and S. Yanagida, Intrazeolite nanostructure of Nd(III) complex giving strong near-infrared luminescence, J. Phys. Chem. B, 2003, 107, 11302–11306 CrossRef CAS.
- K. Sato, Y. Nishimura, N. Matsubayashi, M. Imamura and H. Shimada, Structural changes of Y zeolites during ion exchange treatment: effects of Si/Al ratio of the starting NaY, Microporous Mesoporous Mater., 2003, 59, 133–146 CrossRef CAS.
- H. Klein, H. Fuess and M. Hunger, Cation Location and Migration in Lanthanum-exchanged Zeolite NaY studied by X-Ray Powder Diffraction and MAS NMR spectroscopy, J. Chem. Soc., Faraday Trans., 1995, 91, 1813–1824 RSC.
- J. G. Nery, M. V. Giotto, Y. P. Mascarenhas, D. Cardoso, F. M. Z. Zotin and E. F. Sousa-Aguiar, Rietveld refinement and solid state NMR study of Nd-, Sm-, Gd-, and Dy-containing Y zeolites, Microporous Mesoporous Mater., 2000, 41, 281–293 CrossRef CAS.
- J. F. Wang, K. X. Wang, J. Q. Wang, L. Li and J. S. Chen, Decomposition of CO2 to carbon and oxygen under mild conditions over a zinc-modified zeolite, Chem. Commun., 2012, 48, 2325–2327 RSC.
- G. Cruciani, Zeolites upon heating: Factors governing their thermal stability and structural changes, J. Phys. Chem. Solids, 2006, 67, 1973–1994 CrossRef CAS.
- B. Thomas and S. Sugunan, Rare-earth (Ce3+, La3+, Sm3+, and Re3+) exchanged Na-Y zeolites and K-10 clay as solid acid catalysts for the synthesis of benzoxazole via Beckmann rearrangement of salicylaldoxime, Microporous Mesoporous Mater., 2006, 96, 55–64 CrossRef CAS.
- M. H. Ford, S. M. Auerbach and P. A. Monson, Further studies of a simple atomistic model of silica: thermodynamic stability of zeolite frameworks as silica polymorphs, J. Chem. Phys., 2007, 126, 144701 CrossRef PubMed.
- M. H. Ford, S. M. Auerbach and P. A. Monson, The mechanical properties and phase behavior of silica: a simple model based on low coordination and strong association, J. Chem. Phys., 2004, 121, 8415 CrossRef CAS PubMed.
- D. S. Shy, S. H. Chen, J. Lievens, S. B. Liu and K. J. Chao, Distribution of Cations in Lanthanum-exchanged NaY Zeolites, J. Chem. Soc., Faraday Trans., 1991, 87, 2855–2859 RSC.
- H. S. Park and K. Seff, Crystal structures of fully La3+-exchanged zeolite X: an intrazeolitic La2O3 continuum, hexagonal planar and trigonally monocapped trigonal prismatic coordination, J. Phys. Chem. B, 2000, 104, 2224–2236 CrossRef CAS.
- T. Frising and P. Leflaive, Extraframework cation distributions in X and Y faujasite zeolites, Microporous Mesoporous Mater., 2008, 114, 27–63 CrossRef CAS.
- F. E. Trigueiro, D. F. J. Monteiro, F. M. Z. Zotin and E. F. Sousa-Aguiar, Thermal stability of Y zeolites containing different rare earth cations, J. Alloys Compd., 2002, 344, 337–341 CrossRef CAS.
- M. Ryo, Y. Wada, T. Okubo and S. Yanagida, Near-IR emission of Nd(III) encaged in nanosized zeolites, Res. Chem. Intermed., 2004, 30, 191–205 CrossRef CAS.
- L. Hui, G. Sa Chu Rong, K. Imakita and M. Fujii, Enhanced near infrared emission from the partially vitrified Nd3+ and silver co-doped zeolite Y, J. Appl. Phys., 2014, 115, 033507 CrossRef.
Footnote |
† Electronic supplementary information (ESI) available: The characterization results for the NaY zeolite and NDG product. See DOI: 10.1039/c6qi00428h |
|
This journal is © the Partner Organisations 2017 |
Click here to see how this site uses Cookies. View our privacy policy here.