Incorporation of large guest molecules into liposomes via chemical reactions in lipid membranes†
Received
28th October 2016
, Accepted 25th November 2016
First published on 25th November 2016
Abstract
The incorporation of hydrophobic guest molecules into lipid membranes by the exchange of the guest molecule from a cyclodextrin (CDx) complex to a liposome is limited to guest molecules that can be included in CDxs. To solve this problem, large guest molecules were incorporated into liposomes by chemical reactions of guest molecules in lipid membranes. Stable lipid-membrane-incorporated fullerene derivatives with large substituent(s) were prepared by Diels–Alder reactions in lipid membranes.
1. Introduction
Since the 1970s, liposomes have shown considerable promise as drug carriers, and have been used in clinical applications such as cancer chemotherapy and treatment of fungal infections.1–6 However, problems arise in the preparation of lipid-membrane-incorporated guest molecules (LMIGs): (i) liposomes are labilised by incorporation of hydrophobic guest molecules at high concentrations in lipid membranes; and (ii) some of the hydrophobic guest molecules can precipitate out of the lipid membranes. To prevent these problems, we recently developed a new method for the preparation of LMIGs based on the exchange reaction of a hydrophobic π-molecule via its cyclodextrin (CDx) complex to a liposome; we call this the ‘exchange method’.7–20 The exchange method enables the preparation of stable LMIGs with high concentrations of large π-guest molecules such as C60, C70 and porphyrin derivatives, which cannot be prepared by conventional methods.12–20 However, the exchange method has limitations because it requires the use of guest molecules in CDxs at high concentrations.19 For example, the use of CDxs containing C60 derivatives is limited because large or hydrophobic substituents prevent complexation with γ-CDx.19 Other methods for the incorporation of large guest molecules into liposomes are therefore needed. To solve this problem, we prepared large guest molecules by chemical reactions of guest molecules in lipid membranes (Scheme 1). To the best of our knowledge, there are few previous reports of chemical reactions inside or on the surfaces of lipid membranes to enhance the morphological stability of liposomes21–23 and enzymatic reactions by membrane proteins.24 However, there is no precedent for a reaction among guest molecules inside a lipid membrane. In this paper, we describe the preparation of lipid-membrane-incorporated 2 and 3 (LMI2 and LMI3) via a [4 + 4] photoreaction and a Diels–Alder reaction, respectively, in lipid membranes (Fig. 1).
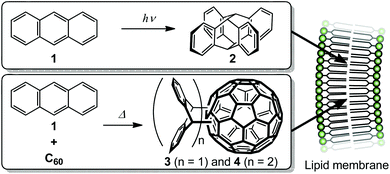 |
| Scheme 1 Reactions in lipid membranes. | |
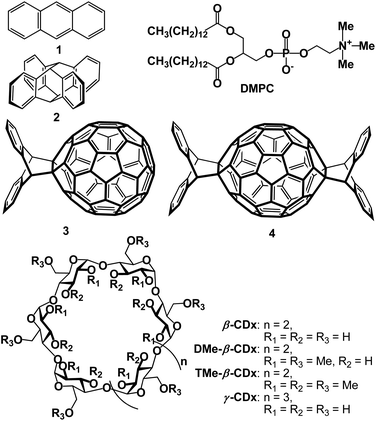 |
| Fig. 1 Compound structures. | |
2. Results and discussion
2.1 Formation of lipid-membrane-incorporated 2 (LMI2) by the photoreaction
We attempted to prepare LMI2via a photoreaction of LMI1. We previously reported that hydrophobic π-guest molecules such as azobenzene, pyrene, or naphthol can be incorporated by premixing at [guest]/[lipid] = 10 mol%.25,26 LMI1 was prepared in the same manner as these guest molecules. The solubility of 1 in the liposomes was determined based on the absorbance of LMI1 at 381 nm after subtraction of scattering by the DMPC liposomes (Fig. S1†). The inset in Fig. S1† shows that the absorbance of LMI1 at 381 nm increased linearly with increasing [1]/[DMPC] below 30 mol% (Fig. S1†). The absorbance of LMI1 decreased after incubation for several hours, indicating that the aqueous solution of LMI1 was unstable. The absorbance at 254 nm became constant after 2 h of incubation, as shown in the inset in Fig. 2A, showing that the aqueous solution of LMI1 was stable at a concentration of 1 of 0.014 mM. The concentration was determined based on the absorbance of 1 after addition of DMSO (2.7 mL) to a sample solution (0.3 mL) to collapse the liposomes ([1]/[DMPC] = 1.4 mol%). UV-vis absorption spectra were recorded to determine the stability of the LMI1 solution prepared by premixing at [1]/[DMPC] = 1.4 mol%. The LMI1 solution was stable and gave no precipitation for at least 10 d at room temperature (Fig. 2B); it was used to prepare LMI2.
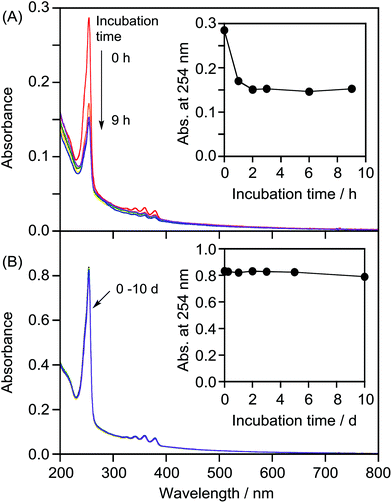 |
| Fig. 2 UV-vis absorption spectra of LMI1 prepared by premixing in water (A) with incubation times of 0 (red line), 1 (orange line), 2 (yellow line), 3 (green line), 6 (blue line) and 9 (purple line) h at 25 °C ([DMPC] = 1.0 mM, [1] = 0.1 mM) (all samples were taken after centrifugation at 18 000g for 3 min) and (B) with incubation times of 0 (red line), 0.04 (orange line), 0.25 (yellowish orange line), 1 (yellow line), 2 (light-green line), 3 (green line), 5 (blue line) and 10 (purple line) d at 25 °C ([DMPC] = 4.00 mM, [1] = 0.056 mM). Insets show changes in LMI1 absorption at 254 nm. | |
LMI2 was prepared via a photoreaction by irradiating an aqueous solution of LMI1 at 365 nm (1.5 W m−2, Scheme 1).27 A precipitate was formed during irradiation. The mixture was centrifuged and the 1H NMR spectrum of the precipitate in CDCl3 was recorded. As shown in Fig. S2,† the precipitate consisted of 2 and a small amount of DMPC. DMPC was removed from the precipitate by washing with water. The absorption at 250 nm from the benzene rings of 2 expected for light scattering by DMPC liposomes was not observed (Fig. S3†). This result suggests that all the 2 produced was released from the lipid membranes and formed a precipitate in water. The formation of 2 after irradiation for 12 h was confirmed by the 1H NMR spectrum in CDCl3 of the freeze-dried precipitate. Fig. 3B and S4B† show that most of 1 in the liposomes reacted and 2 was formed. We measured the photodimerisation rate of 1 based on the changes in the UV-vis absorption spectra at 375 nm in hexane and 381 nm in liposomes (Fig. 4). DMSO (2.7 mL) was added to a sample solution (0.3 mL) to collapse the liposomes and dissolve the precipitated 2 to remove the effect of light scattering by DMPC liposomes from the LMI1 spectrum ([1] = 0.07 mM, [DMPC] = 5.0 mM). The calculated rate constant was k = 34.6 M−1 s−1 (r = 0.994) (Fig. 4B). Peaks from 2 are observed in Fig. 4B because all the resulting 2 was dissolved in DMSO. The rate constant was compared with that in an organic solvent by photodimerising 1 at the same concentration in hexane (Fig. 4A). The rate constant was determined to be k = 1.76 M−1 s−1 (r = 0.998). The rate in liposomes was about 20 times faster than that in hexane ([1] = 0.07 mM), indicating that a concentration effect operated in the lipid membrane. These results indicate that the resulting 2 was recovered in the precipitate.
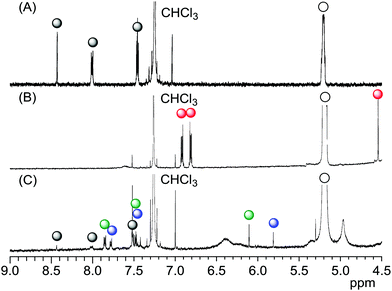 |
| Fig. 3 Partial 1H NMR spectra of (A) LMI1 (●: 1, ○: DMPC), (B) LMI1 after irradiation at 365 nm for 3 h (1.5 W m−2, : 2, ○: DMPC) and (C) LMIC60–1 after heating at 55 °C for 18 h (●: 1, : 3, : 4, ○: DMPC) ([1] = 0.042 mM, [C60] = 0.042 mM, [1]/[DMPC] = 1.4 mol%). All spectra were obtained using CDCl3 solutions of freeze-dried samples. | |
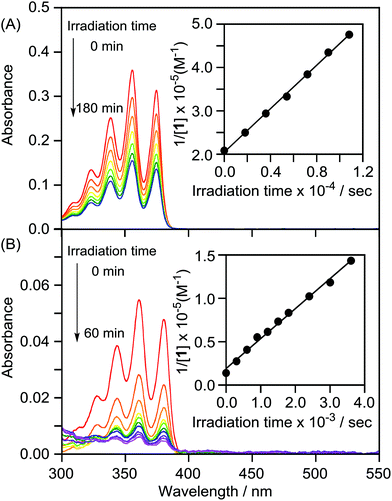 |
| Fig. 4 UV-vis absorption spectra of (A) 1 in hexane at 25 °C for irradiation times of 0, 30, 60, 90, 120, 150 and 180 min ([1] = 0.07 mM) and (B) LMI1 in water at 25 °C for irradiation times of 0, 5, 10, 15, 20, 25, 30, 40, 50 and 60 min ([1] = 0.07 mM, [1]/[DMPC] = 1.4 mol%). Insets: Plots of 1/[1] versus irradiation time. | |
2.2 Formation of LMI3 by the Diels–Alder reaction
We then attempted to prepare LMI3via a Diels–Alder reaction in the lipid membrane. First, a solution of the γ-CDx·C60 complex (prepared from C60 and γ-CDx using the exchange method) and LMI1 in water was heated at 80 °C for 1 h. After this exchange reaction, the reaction mixture was heated at 55 °C, which was above the optimum temperature,28 for 18 h. The reaction afforded 3 and 4 in 23% and 19% yields, respectively, in the liposomes (Fig. 3C and S4c†). These values were almost identical to those previously reported for the reaction of C60 and anthracene in benzene (3: 25% yield, 4: 24% yield).28,29 It was too difficult to determine the rate constant because some of the C60 reacted with 1 during heating at 80 °C for 1 h in the exchange method. The average hydrodynamic diameters in LMI3–4 were 77 nm, suggesting that the liposome morphology did not change after the chemical reaction (Table 1).
Table 1 Average hydrodynamic diameters (Dhy, nm) determined by dynamic light scattering at 25 °C
|
Average Dhy/nm |
PDIa |
PDI: polydispersity index.
|
DMPC liposome |
78.2 |
0.073 |
LMI1 |
76.7 |
0.063 |
LMI3–4 |
77.4 |
0.084 |
The stability of the LMI3–4 solution was determined based on changes in the absorbance of 3–4 at 254 nm (Fig. S5†). An aqueous solution of LMI3–4 was stable for more than 10 d (Fig. S5†).
2.3 Formation of LMI2 by the premixing method and the exchange method
We attempted to prepare LMI2 using a premixing method (Scheme S1A†), but the absorption bands in the UV-vis spectrum of the resulting solution were weaker than those of 2 in CHCl3 (Fig. 5A, orange and purple lines). This indicates that 1,2-dimyristoyl-sn-glycero-3-phosphocholine (DMPC) liposomes could not solubilise 2 in water by premixing because the large self-aggregates of 2 formed in dry thin lipid membranes during the concentration step were removed by filtration through a polytetrafluoroethylene filter (0.45 μm pore size) after addition of water.
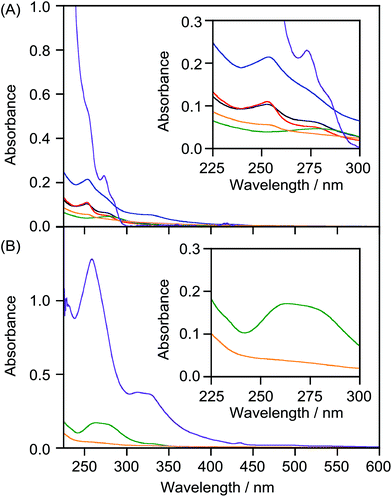 |
| Fig. 5 UV-vis absorption spectra of (A) LMI2 prepared by the premixing method (orange line, [2] = 0.2 mM), 2·β-CDx (black line), 2·DMe-β-CDx (red line), 2·TMe-β-CDx (blue line) and 2·γ-CDx (green line) mixtures ([2] = 7.0 mM, [CDx] = 14.0 mM) in water and 2 in CHCl3 (purple line, [2] = 0.14 mM) and (B) LMI3 pre-pared by premixing (orange line, [3] = 0.1 mM), the 3·γ-CDx (green line, [3] = 3.44 mM, [γ-CDx] = 13.9 mM) mixture in water and 3 in CHCl3 (purple line, [3] = 0.22 mM). Insets show the 225–300 nm region. | |
We tried to prepare LMI2 using the exchange method. For this method, the 2·β-CDx or γ-CDx complex needs to be prepared.7 However, β-CDx and γ-CDx barely solubilised 2, as shown by the 1H NMR (Fig. S6A and S6D†) and UV-vis absorption spectra (Fig. 5A, black and green lines), because 2 was larger than the cavities of β-CDx and γ-CDx. Furthermore, 2 was not solubilised even by heptakis(2,6-di-O-methyl)-β-cyclodextrin (DMe-β-CDx) and heptakis(2,3,6-tri-O-methyl)-β-cyclodextrin (TMe-β-CDx), which have flexible cavities (Fig. 1, 5A, red and blue lines, S6B and S6C†). LMI2 therefore could not be prepared using the exchange method (Scheme S1B†).
2.4 Formation of LMI3 by the premixing and exchange methods
The preparations of LMI3 using the premixing (Scheme S1a†) and exchange methods (Scheme S1b†) were both unsuccessful. The UV-vis spectrum of the material obtained using the premixing method had weak absorption bands (Fig. 5B, orange line); this indicates that the liposomes could not solubilise 3 in water using the premixing method. This result is similar to those for the preparations of LMIC60 and LMIC70 using the premixing method; extensive self-aggregation of 3 occurred.
A γ-CDx·3 complex was prepared for the exchange reaction. Komatsu et al. found that high-speed vibration milling was more efficient than the classical ball-milling method for supramolecular complexation of C60 or C70.14 However, because of the poor solubility of the γ-CDx·3 complex, the absorption bands in its UV-vis spectrum were very weak (Fig. 5B, green line). The 1H NMR spectrum of γ-CDx·3 showed a small peak at δ = 5.4 ppm, arising from self-aggregation30 and very weak peaks assignable to the 2
:
1 γ-CDx·C60 derivative complex (δ = 4.1 and 5.0 ppm) were observed (Fig. S7†). The concentration of 3 in the γ-CDx·3 complex was too low for the preparation of LMI3 using the exchange method.
3. Experimental
3.1 Materials
Anthracene (1), β-CDx, TMe-β-CDx and γ-CDx were purchased from Wako Pure Chemical Industries Ltd (Tokyo, Japan). DMe-β-CDx was purchased from Sigma-Aldrich Chemical Co., Inc. (St Louis, MO, USA). 1,2-Dimyristoyl-sn-glycero-3-phosphocholine (DMPC) was obtained from NOF Corp. (Tokyo, Japan). Compounds 2,273
28 and 4
28 were synthesised according to previously reported methods.
3.2 Preparation of LMI1 using the premixing method
A mixture of 1 (0.010 mg, 5.6 × 10−8 mol) and DMPC (2.71 mg, 4.0 × 10−6 mol) in CHCl3 (1 mL) was dried under a N2 gas flow. Water (1.0 mL) was added and the resulting mixture was shaken for 1 min on a vortex mixer. To achieve a change from multilamellar to unilamellar vesicles and the formation of a narrow size distribution, the solution was frozen and thawed eight times and extruded 11 times (LiposoFast-Basic; Avestin Inc., Ottawa, Canada) with two stacked polycarbonate membranes of a pore size of 50 nm. The final concentrations of 1 and DMPC in LMI1 were determined to be 0.056 and 4.0 mM, respectively, based on the peak intensities of 1 and DMPC relative to that of a DMSO peak (0.1 mM), which was adopted as an internal standard.
3.3 Attempts to prepare LMI2 and LMI3 using the premixing method
A mixture of 2 or 3 (0.071 or 0.18 mg, 2.0 × 10−7 mol) and DMPC (1.36 mg, 2.0 × 10−6 mol) in CHCl3 (1 mL) was dried under a N2 gas flow. Water (1.0 mL) was added and the resulting mixture was shaken for 1 min on a vortex mixer. To achieve a change from multilamellar to unilamellar vesicles and the formation of a narrow size distribution, the solution was frozen and thawed eight times and extruded 11 times (LiposoFast-Basic, Avestin Inc.) with two stacked polycarbonate membranes of a pore size of 50 nm. Neither compound 2 nor compound 3 was present in the aqueous solution of DMPC liposomes.
3.4 Preparation of 2·CDx complexes
Compound 2 (2.50 mg, 7.00 × 10−6 mol) and CDx (β-CDx, DMe-β-CDx, TMe-β-CDx or γ-CDx, 1.40 × 10−5 mol) were placed in an agate capsule with two agate mixing balls and the resulting mixture was vigorously agitated at 30 Hz for 20 min using a high-speed vibration mill (MM 200; Retsch Co., Ltd, Haan, Germany). The solid mixture was suspended in pure water (1.0 mL), producing a dark-purple emulsion. Non-dispersed 2 was removed from the solution by centrifugation (18
000g, 25 °C, 20 min).
3.5 Preparation of the 3·γ-CDx complex
Compound 3 (3.10 mg, 3.44 × 10−6 mol) and γ-CDx (18.0 mg, 1.39 × 10−5 mol) were placed in an agate capsule with two agate mixing balls and the resulting mixture was vigorously agitated at 30 Hz for 20 min using a high-speed vibration mill (MM 200, Retsch Co., Ltd). The solid mixture was suspended in pure water (1.0 mL), producing a dark-purple emulsion. Non-dispersed 3 was removed by centrifugation (18
000g, 25 °C, 20 min).
3.6 Preparation of LMI2 by photoirradiation
An aqueous solution of LMI1 (6.0 mL, [1] = 0.056 mM, [DMPC] = 4.0 mM) was photoirradiated at 365 nm for 3 h in a 1 cm quartz cell (1.5 W m−2). After the reaction, DMSO was added (DMSO
:
H2O = 9
:
1, v/v) and the UV-vis absorption spectrum was recorded. The final products were identified from the 1H NMR spectrum of the freeze-dried mixture dissolved in CDCl3 (1.0 mL).
3.7 Preparation of LMI3–4
An aqueous solution of LMI1 (2.0 mL, [1] = 0.084 mM, [DMPC] = 6.0 mM) was mixed with an aqueous solution of the C60·γ-CDx complex (2.0 mL, [C60] = 0.084 mM). Heating at 80 °C for 1 h gave an aqueous solution of LMIC60–1 (4.0 mL, [1] = 0.042 mM, [C60] = 0.042 mM, [DMPC] = 3.0 mM). The mixture was heated at 55 °C for 18 h and freeze-dried. The final products were identified from the 1H NMR spectrum of the resulting mixture dissolved in CDCl3 (1.0 mL).
3.8 UV-vis absorption spectra
UV-vis spectra were recorded using a UV-3600PC spectrophotometer (Shimadzu Corp., Kyoto, Japan). All experiments were performed at 25 °C using a 1 cm cell.
3.9
1H NMR spectroscopy
1H NMR data were recorded using a Varian 400-MR (400 MHz) spectrometer (Varian Associates Inc., Palo Alto, CA, USA).
3.10 Dynamic light scattering
The hydrodynamic diameters of LMI1 and LMI3–4 were measured using an electrophoretic light-scattering instrument with a laser Doppler system (Zetasizer Nano ZS, Malvern Instruments Ltd, Malvern, UK).
4. Conclusions
We prepared LMI3–4via a chemical reaction in lipid membranes. An LMI3–4 solution had high long-term stability. In contrast, LMI3–4 could not be prepared by either the premixing or the exchange method. Compound 2 was released from the lipid membranes after the photoreaction of 1 because of its low affinity for lipid membranes. The chemical reaction rate in the liposomes was 20 times those in organic solvents because of a concentration effect. We believe that the method using chemical reactions will become a general technique for the preparation of large molecules incorporated within lipid membranes.
Acknowledgements
This work was supported by the Japanese Society for the Promotion of Science (JSPS) KAKENHI Grant-in-Aid for Scientific Research (B) (Grant No. JP16H04133) and a Grant-in-Aid for Challenging Exploratory Research (Grant No. JP16K13982).
Notes and references
- A. D. Bangham, Prog. Biophys. Mol. Biol., 1968, 18, 29–36 CrossRef CAS PubMed.
- D. D. Lasic, Trends Biotechnol., 1998, 16, 307–321 CrossRef CAS PubMed.
- G. Bozzuto and A. Molinari, Int. J. Nanomed., 2015, 10, 975–999 CrossRef CAS PubMed.
- Y. Kaneda, Adv. Drug Delivery Rev., 2000, 43, 197–205 CrossRef CAS PubMed.
- T. M. Allen and P. R. Cullis, Adv. Drug Delivery Rev., 2013, 65, 36–48 CrossRef CAS PubMed.
-
Liposomes: A Practical Approach, ed. V. P. Torchilin and V. Weissig, Oxford University Press, Oxford, 2nd edn, 2003 Search PubMed.
- A. Ikeda, Chem. Rec., 2016, 16, 249–260 CrossRef CAS PubMed.
- A. Ikeda, T. Sato, K. Kitamura, K. Nishiguchi, Y. Sasaki, J. Kikuchi, T. Ogawa, K. Yogo and T. Takeya, Org. Biomol. Chem., 2005, 3, 2907–2909 CAS.
- A. Ikeda, Y. Doi, K. Nishiguchi, K. Kitamura, M. Hashizume, J. Kikuchi, K. Yogo, T. Ogawa and T. Takeya, Org. Biomol. Chem., 2007, 5, 1158–1160 CAS.
- A. Ikeda, Y. Doi, M. Hashizume, J. Kikuchi and T. Konishi, J. Am. Chem. Soc., 2007, 129, 4140–4141 CrossRef CAS PubMed.
- A. Ikeda, T. Hida, T. Iizuka, M. Tsukamoto, J. Kikuchi and K. Yasuhara, Chem. Commun., 2014, 50, 1288–1291 RSC.
- A. Ikeda, S. Hino, T. Mae, Y. Tsuchiya, K. Sugikawa, M. Tsukamoto, K. Yasuhara, H. Shigeto, H. Funabashi, A. Kuroda and M. Akiyama, RSC Adv., 2015, 5, 105279–105287 RSC.
- A. Ikeda, S. Hino, K. Ashizawa, K. Sugikawa, J. Kikuchi, M. Tsukamoto and K. Yasuhara, Org. Biomol. Chem., 2015, 13, 6175–6182 CAS.
- K. Komatsu, K. Fujiwara, Y. Murata and T. Braun, J. Chem. Soc., Perkin Trans. 1, 1999, 2963–2966 RSC.
- A. Ikeda, T. Genmoto, N. Maekubo, J. Kikuchi, M. Akiyama, T. Mochizuki, S. Kotani and T. Konishi, Chem. Lett., 2010, 39, 1256–1257 CrossRef CAS.
- A. Ikeda, R. Aono, N. Maekubo, S. Katao, J. Kikuchi and M. Akiyama, Chem. Commun., 2010, 47, 12795–12797 RSC.
- A. Ikeda, M. Ishikawa, R. Aono, J. Kikuchi, M. Akiyama and W. Shinoda, J. Org. Chem., 2013, 78, 2534–2541 CrossRef CAS PubMed.
- A. Ikeda, T. Iizuka, N. Maekubo, R. Aono, J. Kikuchi, M. Akiyama, T. Konishi, T. Ogawa, N. Ishida-Kitagawa, H. Tatebe and K. Shiozaki, ACS Med. Chem. Lett., 2013, 4, 752–756 CrossRef CAS PubMed.
- A. Ikeda, A. Hirata, M. Ishikawa, J. Kikuchi, S. Mieda and W. Shinoda, Org. Biomol. Chem., 2013, 11, 7843–7851 CAS.
- A. Ikeda, M. Ishikawa, J. Kikuchi and K. Nobusawa, Chem. Lett., 2013, 42, 1137–1139 CrossRef CAS.
- E. Lopez, D. F. O'Brien and T. H. Whitesides, J. Am. Chem. Soc., 1982, 104, 305–307 CrossRef CAS.
- K. Katagiri, R. Hamasaki, K. Ariga and J. Kikuchi, J. Am. Chem. Soc., 2002, 124, 7892–7893 CrossRef CAS PubMed.
- K. Yasuhara, T. Kawataki, S. Okuda, S. Oshima and J. Kikuchi, Chem. Commun., 2013, 49, 665–667 RSC.
- W. Wickner, A. J. Driessen and F. U. Hartl, Annu. Rev. Biochem., 1991, 60, 101–124 CrossRef CAS PubMed.
- A. Ikeda, T. Hida, T. Nakano, S. Hino, K. Nobusawa, M. Akiyama and K. Sugikawa, Chem. Lett., 2014, 43, 1551–1553 CrossRef CAS.
- A. Ikeda, K. Ashizawa, Y. Tsuchiya, M. Ueda and K. Sugikawa, RSC Adv., 2016, 6, 78505–78513 RSC.
- H. Bouas-Laurent, A. Castellan and J.-P. Desvergne, Pure Appl. Chem., 1980, 52, 2633 CrossRef CAS , and references cited therein.
- M. Tsuda, T. Ishida, T. Nogami, S. Kurono and M. Ohashi, J. Chem. Soc., Chem. Commun., 1993, 1296–1298 RSC.
- A. Simonyan and I. Gitsov, Langmuir, 2008, 24, 11431–11441 CrossRef CAS PubMed.
- Z. Yoshida, H. Takekuma, S. Takekuma and Y. Matsubara, Angew. Chem., Int. Ed. Engl., 1994, 33, 1597–1599 CrossRef.
Footnote |
† Electronic supplementary information (ESI) available: Schematic representation of the premixing and exchange methods, 1H NMR spectra and UV-vis absorption spectra. See DOI: 10.1039/c6ob02343f |
|
This journal is © The Royal Society of Chemistry 2017 |