DOI:
10.1039/C7NR06415B
(Paper)
Nanoscale, 2018,
10, 239-249
Lectin-gated and glycan functionalized mesoporous silica nanocontainers for targeting cancer cells overexpressing Lewis X antigen†
Received
28th August 2017
, Accepted 21st November 2017
First published on 22nd November 2017
Abstract
Gated mesoporous silica nanoparticles can deliver payload upon the application of a predefined stimulus, and therefore are promising drug delivery systems. Despite their important role, relatively low emphasis has been placed on the design of gating systems that actively target carbohydrate tumor cell membrane receptors. We describe herein a new Lewis X (Lex) antigen-targeted delivery system comprising mesoporous silica nanoparticles (MSNs) loaded with ATTO 430LS dye, functionalized with a Lex derivative (1) and capped with a fucose-specific carbohydrate-binding protein (Aleuria aurantia lectin (AAL)). This design takes advantage of the affinity of AAL for Lex overexpressed receptors in certain cancer cells. In the proximity of the cells, AAL is detached from MSNs to bind Lex, and selectins in the cells bind Lex in the gated MSNs, thereby inducing cargo delivery. Gated MSNs are nontoxic to colon cancer DLD-1 cells, and ATTO 430LS dye delivered correlated with the amount of Lex antigen overexpressed at the DLD-1 cell surface. This is one of the few examples of MSNs using biologically relevant glycans for both capping (via interaction with AAL) and targeting (via interaction with overexpressed Lex at the cell membrane).
Introduction
Within the wide variety of functional materials for drug delivery applications, mesoporous silica nanoparticles (MSNs) present a unique combination of features, including high stability, biocompatibility, homogeneous porosity, tunable size, large storage capacity and versatile functionalization.1 MSNs can be functionalized to obtain gated nanoparticles, which display “zero release” unless a predefined stimulus is applied. This renders a suitable technology for the design of effective drug-delivery nanodevices.2–12 Chemical, physical and biochemical triggers have been used to develop gated materials with controlled-release features. The use of biomolecules in capped mesoporous nanostructures has been recently demonstrated to allow for efficient drug delivery in more realistic biological settings, additionally providing selectivity in gated devices for highly specific applications.13–21 Biomolecules can act not only as gates but also as ligands to target specific cells and tissues which display over-expressed receptors. Hence, the use of site or cell-specific delivery systems can improve therapy effectivity, and the required dose can be lowered and side effects can be reduced. The targeting ability of bio-gated MSNs is usually based on surface-bound antibodies,22 peptides23 or aptamers.24 However, despite their abundance and biological importance, less emphasis has been placed on the third group of relevant biomolecules, namely glycans (carbohydrates or saccharides). Cell surface glycans and glycoproteins play an important role in cell fate related to physiological and pathological processes such as cancer.25–28 Moreover, glycosylation modifications are universal features of malignant transformation and tumor progression.27 Glycan changes in malignant cells can occur in diverse ways, such as the lack of expression or excessive expression of certain structures, the persistence of incomplete or truncated structures, the accumulation of precursors and the appearance of novel structures.27 Despite the great significance of this group of molecules, only simple saccharides29–34 or the polysaccharide hyaluronic acid35,36 have been employed in MSNs so far, to actively target carbohydrate tumor cell membrane receptors. Surprisingly, the use of glycan/protein interactions has rarely been studied in this context. To the best of our knowledge, only the interactions of Concanavalin A with mannose and glucose have been used to prepare gated MSNs.37,38 Nonetheless, in spite of their relevance, the use of multifunctional systems involving complex glycans, such as Lewis X (Lex) antigen and lectins as targeting and gating moieties, has not yet been addressed. In particular it has been reported that the expression of such carbohydrate antigens (particularly the overexpression of sialyl-Lewis X (sLex)) and associated proteins (glycosidases, glycosyltransferases) changes significantly in malignant cells (aberrant glycosylation),39–41 thereby promoting metastasis.42 Moreover, the antigenic epitope of sLex carbohydrate antigen has been clinically used as a tumor marker for pancreatic cancer, colorectal cancer, bladder cancer and other malignancies.43–45 sLex plays an important role in cancer cells, regarding E-selectin-mediated cell adhesion to vascular endothelial cells during the course of tumor angiogenesis and distant metastasis.46 Furthermore, Lex is known to be involved in selectin-mediated adhesion of cancer cells to the vascular endothelium and is thought to be closely associated with hematogenous metastasis.47,48 Changes in these carbohydrate–antigen expression profiles are especially remarkable in human colon cancer cells, where both sLex and Lex antigens are highly expressed.49
With this background and inspired by such changes in cancer cells, we successfully addressed the design of a new biogated-MSN that targets and delivers cargo in tumoral cells, using a double reciprocal recognition approach. The design is based on the expression of significant amounts of (i) Lex antigen on the surface of certain cancer cells, which is recognized by fucose-binding proteins (lectins) and (ii) the presence of selectins (Lex antigen binding proteins) on the surface of cancer cells, which thus recognize the Lex antigen. The gated system consists of MSNs loaded with a dye (ATTO 430LS), functionalized with a Lex derivative (1) and capped with a fucose-specific carbohydrate-binding protein (Aleuria aurantia lectin (AAL)). Gated nanoparticles were tested in PBS buffer and in the DLD-1 human colorectal adenocarcinoma cell line, with a high expression of sLex and Lex antigen-binding sites. AAL-gated MSNs did not show toxicity toward colon cancer DLD-1 cells, whereas ATTO 430LS dye delivery was found to be dependent on the amount of Lex antigen overexpressed on the DLD-1 cell surface.
Results and discussion
Design and synthesis of lectin-capped MSNs
The design of the controlled-release nanomaterial is depicted in Fig. 1a. MSNs were loaded with the ATTO 430LS dye and surface-functionalized with the fucose-containing Lex (Galβ1–4[Fucα1–3]GlcNAcβ-1) antigen derivative 1 (S2 in Fig. 1a).
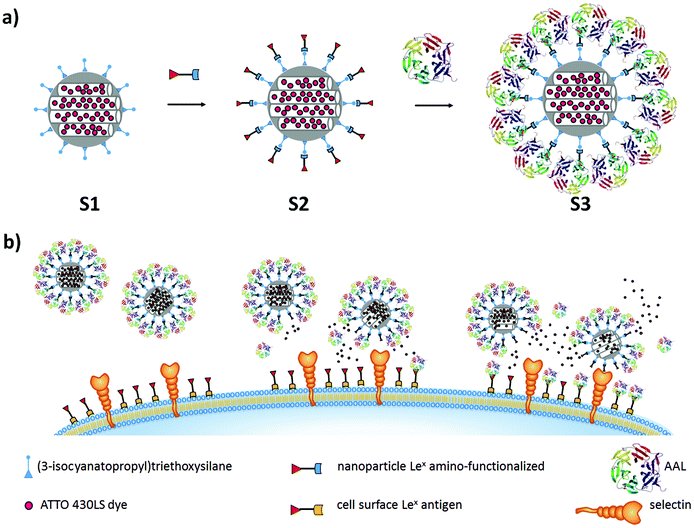 |
| Fig. 1 (a) Representation of the solids synthesized to obtain the lectin-gated and Lex antigen-functionalized MSNs (S3). (b) Opening of the gated system in the presence of specific cellular receptors and cargo release. AAL is detached from MSNs to bind Lex at the cell membrane, and selectins in the cells bind Lex in the gated MSNs, thereby favoring internalization and inducing cargo delivery. | |
As a capping system, the fucose-specific carbohydrate-binding protein AAL was selected. AAL was expected to interact with the Lex antigen via multivalent fucose–lectin interactions, thereby inhibiting cargo release (S3 in Fig. 1a). The opening of gated MSNs would be based on a displacement reaction via AAL binding onto overexpressed Lex receptors, at the cell membrane. Moreover, the anchored Lex antigen derivative 1 on the MSNs was expected to additionally bind fucose-binding proteins (selectins) on the cell surface, favoring endocytosis of the nanoparticles (Fig. 1b). Therefore, the Lex antigen plays a double role: on the one hand as a linking molecule to AAL, so that the pores are capped, and on the other hand as a targeting agent toward selectins. At the same time, the AAL lectin also plays a double role: capping the pores via interaction with Lex derivative 1, and targeting overexpressed Lex receptors on the cell membrane (see Fig. 1).
In the present work, MCM-41-based MSNs were selected.50 The mesoporous nanoparticles were prepared by using a precursor and the surfactant hexadecyl-trimethylammonium bromide (CTABr) as the porogen species. Bare MSNs were obtained upon surfactant removal by extraction. The inorganic support was then loaded with the fluorescent dye ATTO 430LS, and the outer surface of the nanoparticles was functionalized with (3-isocyanatopropyl)triethoxysilane to obtain solid S1 (Fig. 1a). The reaction of the isocyanate groups with the amino-functionalized Lex antigen derivative 1 yielded solid S2. Finally, the nanoparticles were capped in PBS, in the presence of AAL (solid S3). Cargo delivery from S3 was anticipated to occur only in the presence of increased amounts of fucose or fucose-containing glycans. Buffered suspensions of solid S3 can be stored at 4° C for weeks without any loss of functionality.
The amino-functionalized Lex antigen derivative 1 was obtained by following established protocols,51 with two main modifications (Fig. 2). For the synthesis of trisaccharide Lex, 3,4,6-tri-O-acetyl-2-deoxy-2-(2,2,2-trichloroethoxycarbonylamino)-1-(2-N-benzyloxycarbonylaminoethyl)-D-glucopyranoside (1), pertrimethylsilyl-L-fucose (2) and 2,3,4,6-tetra-O-acetyl-D-galactopyranose (3) were prepared as monosaccharide building blocks for the Lex synthesis (Fig. 2). First, trichloroethyloxycarbonyl (Troc) was employed as the amino protecting group, which ensures high glycosyl accepting properties of the C-3 hydroxyl of the glucosamine unit52 and offers efficient neighboring group participation to yield stereoselective formation of β-glycoside. The trisaccharide Lex was chemically synthesized by bearing an amino-ended linker for attaching to the mesoporous surface using a thiourea linkage.53
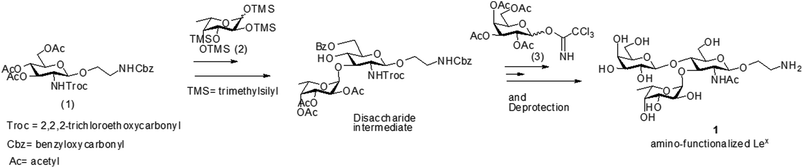 |
| Fig. 2 Synthesis of Lex antigen derivative 1. | |
Materials characterization
MSNs and solids S1, S2 and S3 were characterized by standard techniques. Powder X-ray diffraction (PXRD) of the as-made MSNs (see Fig. S1 in the ESI†) showed four low-angle peaks, corresponding to the (100), (110), (200) and (210) reflections of a hexagonal array, typical of MCM-41-type mesoporous materials. After extraction of the surfactant template, a slight shift of the main peak (assigned to the (100) Brag reflection) was observed, corresponding to a cell contraction of ca. 2 Å (see Fig. S1 in the ESI†). From N2 adsorption–desorption isotherm studies, a typical curve for mesoporous materials was observed for calcined MSNs. The application of the BET model yielded a total specific surface area of 955.2 m2 g−1 and a pore volume of 0.75 cm3 g−1. The BJH model was also used at intermediate relative pressures, resulting in a narrow pore distribution centered at 2.52 nm (see Fig. S5 in the ESI†). A cell parameter a0 of 4.35 nm and a wall thickness of 1.83 nm were calculated. Nanoparticles S1, S2 and S3 were obtained in relatively low amounts and could not be characterized by N2 isotherm studies.
All materials were characterized by Transmission Electron Microscopy (TEM). The presence of spherical mesoporous nanoparticles with a diameter of ca. 200 nm was clearly observed in all samples (see Fig. S3 in the ESI†). Dynamic light scattering (DLS) was used to assess the hydrodynamic diameter of the particles. Table 1 summarizes the obtained data for the starting MSNs, S1, S2, and S3. A gradual increase in diameter was observed from bare nanoparticles (202 nm) to solid S2 (255 nm) and from solid S2 to S3 (260 nm). Finally, the organic content of S1, S2 and S3 was determined by thermogravimetric and elemental analysis (TGA). Table 2 shows the organic content of ATTO 430LS dye, 3-isocyanatopropyl, Lex antigen derivative and AAL in %wt.
Table 1 Mean hydrodynamic diameter obtained from DLS studies of MSN and solids S1, S2 and S3
MSN (nm) |
S1 (nm) |
S2 (nm) |
S3 (nm) |
202 |
243 |
255 |
260 |
Table 2 Organic content in wt% for solids S1, S2 and S3
|
ATTO 430LS dye |
3-Isocyanatopropyl |
Lex antigen derivative |
AAL |
S1
|
3.50 |
2.77 |
— |
— |
S2
|
3.26 |
2.59 |
6.08 |
— |
S3
|
3.11 |
2.47 |
5.80 |
3.89 |
Delivery studies in solution
Retaining cargo inside a carrier until the target is reached is a crucial requirement in drug delivery systems, so as to reduce side effects and increase drug efficacy. Therefore cargo release studies of S3 were carried out, taking advantage of the high affinity of AAL for L-fucose. In a typical experiment, S3 was suspended in water and the suspension was fractionated into two parts. To one fraction L-fucose was added. Both suspensions were stirred and aliquots were taken at the scheduled times. After centrifugation to remove the solid, the fluorescence of the ATTO 430LS dye delivered to the solution was measured at 547 nm (λex = 433 nm). The drug delivery profile for both experiments is displayed in Fig. 3.
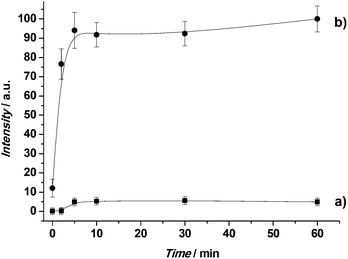 |
| Fig. 3 Release profile of ATTO 430LS dye from solid S3 in the absence (a) and in the presence (b) of L-fucose. | |
In the absence of L-fucose, a flat baseline was obtained, which indicates that ATTO 430LS remained in the pore voids of the nanoparticles, not being released during the time of the experiment. In particular, cargo delivery was lower than 10% after 1 h. In contrast, in the presence of L-fucose, ATTO 430LS release was indicated by an increase in fluorescence with time. The observed behavior is in agreement with a displacement reaction, in which AAL binds L-fucose, the pores are uncapped and payload delivered. To assess the performance of S3 in competitive media, delivery experiments were carried out in cell culture medium, in the presence and in the absence of L-fucose, with similar results (see Fig. S9 in the ESI†).
Evaluation of MSNs S3 in colon cancer cells with high expression of Lex antigen
Controlling cell targeting and penetrability of drugs is a major issue in nanomedicine. Therefore, after demonstrating the effective capping and cargo release of S3, we evaluated the ability of S3 nanoparticles to target human colorectal adenocarcinoma DLD-1 cells.34 In a first step, the presence of AAL-binding ligands (Lex) on the DLD-1 cells was confirmed using labelled AAL (i.e. AAL555). The fluorescence images of cells treated with AAL555 (Fig. 4a) showed a clear membrane staining pattern (red), which was not found in control DLD-1 cells treated only with media (Fig. 4b). These results corroborated the expression of AAL-binding ligands (i.e. Lex) on the DLD-1 membrane. In a second step, the effect of S3 on DLD-1 cell viability was studied. The DLD-1 cells were treated with different concentrations of S3 for 24 h and cell viability was determined by MTS assays.54,55 The results are shown in Fig. 5a, in which values are given as a mean of quadruplets ± S.D. The AAL-gated solid S3 did not affect the viability of the DLD-1 cells after 24 h incubation at concentrations up to 10 μg mL−1.
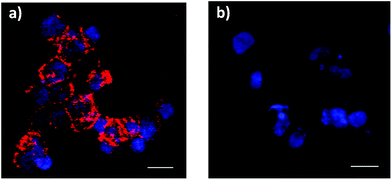 |
| Fig. 4 Fluorescence microscopy images of DLD-1 cells incubated with media with 40 μg mL−1 AAL555 (red) (a) or only media (b). Nuclei were stained with DAPI (blue). Magnification: 10×. Scale bar: 30 μm. | |
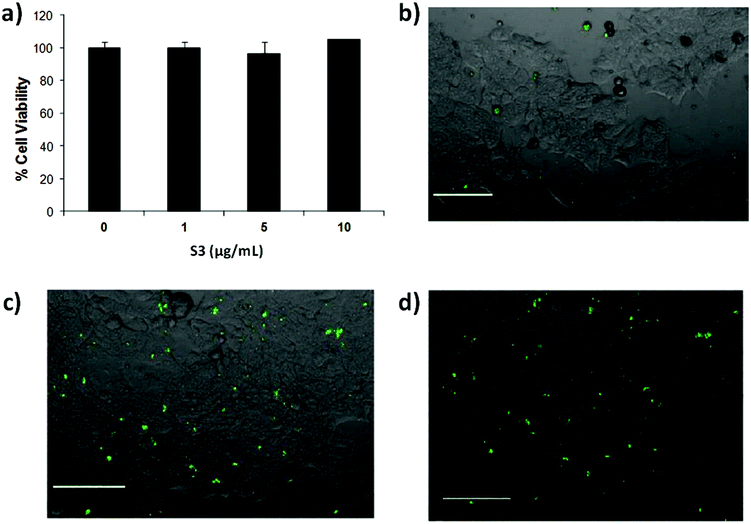 |
| Fig. 5 (a) S3-DLD-1 viability assay with the colorimetric MTS method. Experiments were carried out in quadruplets and data are plotted as mean ± SD. (b–d) S3-cell interaction assessment. Confocal microscopy imaging of DLD-1 cells after incubation with S3 for 4 h (b), 24 h (c, d), not washed (b, c) and washed with PBS (d). The same region was considered for images (c) and (d). Experiments were carried out in duplicates. 10× magnification. Scale bar: 100 μm. | |
Internalization of S3 on DLD-1 cells and dye delivery were also studied. Experiments were carried out in duplicates. Fluorescence microscopy experiments on the DLD-1 cells after 4 h incubation with S3 showed the release of ATTO 430LS inside the cells (Fig. 5b). Interestingly, the cells incubated with S3 for 24 h showed a brighter green staining (Fig. 5c and d), in agreement with a higher amount of ATTO 430LS released with time. The confirmation of cargo release induced by the selective uncapping of S3 – due to AAL displacement to bind the Lex ligands present on the DLD-1 membrane – was determined via similar experiments using DLD-1 cells in which Lex and sLex expressions were induced. The overexpression of Lex (and sLex) was prompted by culturing cells in the presence of an epidermal growth factor (EGF) and basic fibroblast growth factor (bFGF).56 From the analyses of these cultures by flow cytometry, it was concluded that the highest expression of Lex and sLex was achieved when DLD-1 cells were cultured for 3 days in the presence of EGF and bFGF (see Fig. 6).
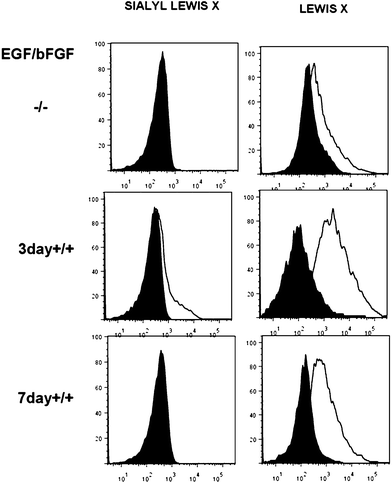 |
| Fig. 6 Expression of Lex and sLex on DLD-1 cells. DLD-1 cells were cultured for 3 or 7 days, in the presence or in the absence of EGF and bFGF. Data from PE-labelled anti-CD15 (Lex) mAb and BD Horizon™ BV711-labelled anti CD15S (sLex) are shown in empty histograms. Filled histograms show isotype fluorescently labeled control antibodies, used as negative controls. | |
Lex and sLex-overexpressing DLD-1 cells and untreated DLD-1 cells were incubated with solid S3 for 24 h. Experiments were carried out in duplicates. Fluorescence images showed a higher ATTO 430LS emission in cells treated with EGF and bFGF (Fig. 7a) than in control cells (Fig. 7b). Green pixels in fluorescence images were counted using the image processing program Image J, and a 3-fold higher ATTO 430LS emission was found in overexpressed Lex cells. This increase in fluorescence correlates well with the increase of Lex on the DLD-1 cells. Colocalization studies performed with overexpressed Lex and sLex DLD-1 cells and S3 were also carried out. Experiments were carried out in duplicates. Most ATTO 430LS dye (green) was found to be internalized and only a small fraction was localized with the plasma membrane (yellow) (Fig. 8a). The orthogonal view of the Z stack (Fig. 8b) confirmed that both ATTO 430LS and nuclei (DAPI-stained, blue) were present at the same planes in the cytoplasm, confirming successful internalization of the gated nanoparticles S3.
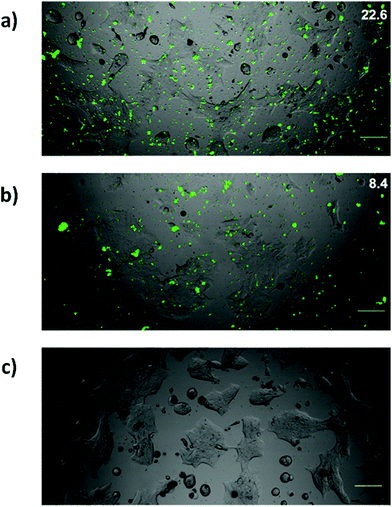 |
| Fig. 7 DLD-1 cultured for 3 days in the presence (a) or in the absence (b, c) of growth factors, and incubated with 8 μg mL−1 of solid S3 (a, b) or only media (c), for 24 h. Scale bar: 100 μm. The green color pixel percentage is shown in the top right corner. Experiments were carried out in duplicates. | |
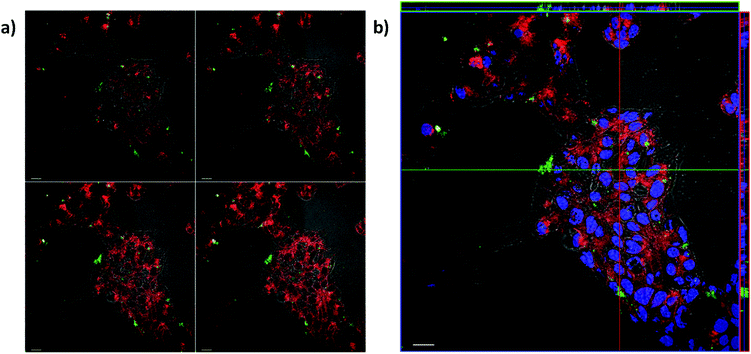 |
| Fig. 8 Colocalization study of S3 and the DLD-1 cell plasma membrane. Cells were cultured for 3 days, in the presence of growth factors and incubated for 24 h with 8 μg mL−1 of the gated solid S3 (ATTO 430LS, green), and stained for the plasma membrane (red) and nuclei (blue). Z-Stack images of 2 μm sections were obtained with a 40× oil objective. (a) Gallery view, (b) orthogonal view. Scale: 20 μm. Experiments were carried out in duplicates. | |
Conclusions
In summary, we have developed a gated Lex-targeted delivery system (S3), using MSNs functionalized with a Lex derivative and capped with AAL. Lex in solid S3 acts as an ideal linker to promote the positioning of AAL onto the surface of the nanoparticle and subsequent pore blockage. In this nanosystem, AAL was able to cap the pores in MSNs and block cargo release (the ATTO 430LS dye). The gated solid displayed a poor cargo delivery, whereas the presence of L-fucose readily induced pore opening and payload delivery. The observed behavior is in agreement with a displacement reaction in which AAL binds L-fucose, the pores are uncapped and payload delivered. Cell viability studies showed that the gated solid S3, at concentrations up to 10 μg mL−1, did not affect the viability of the DLD-1 cells after 24 h incubation. Cargo release in cells was induced by selective uncapping of S3 deriving from the displacement of AAL, to bind Lex and sLex ligands present in the cell membrane. In a second step, when the particles are in the proximity of cancer cells, and AAL is detached from S3 to bind Lex overexpressed in certain cancer cells, Lex in the gated MSNs acts as a targeting agent which helps the nanoparticle's internalization assisted by its recognition by selectins. Then, once uncapped, the anchored Lex-derivative on MSNs would additionally bring the nanoparticle close to the membrane, by interaction with fucose-binding proteins on the cell surface (selectins). This dual targeting facilitates the specific release of cargo in tumoral cells, and helps the whole system to be internalized. Uncapping of S3 and cargo delivery was demonstrated to occur in DLD-1 cells, whilst enhanced internalization was observed in DLD-1 cells, in which Lex expression was enhanced. These results can be considered as promising towards adopting new therapeutic strategies to kill malignant cancer cells that overexpress Lex and sLex, which play important roles in tumor metastasis and angiogenesis. This is one of the few examples based on MSNs that use “saccharides” for capping (via interaction with AAL) and targeting (via interaction with Lex expressed on membranes). We propose that our synthetic strategy can contribute to further design improved delivery systems using highly specific saccharides, such as Lex, which play crucial roles in tumor angiogenesis and metastasis.
Methods
Chemicals
Tetramethyl orthosilicate (TMOS), n-cetyltrimethylammonium bromide (CTAB), sodium hydroxide (NaOH), and (3-isocyanatopropyl)triethoxysilane, triethylamine, as well as all chemicals and solvents for Lex antigen derivative synthesis were purchased from Sigma-Aldrich. Methanol, ethanol, hydrochloric acid (HCl) and acetonitrile were purchased from Scharlab. The dye ATTO 430LS was purchased from ATTO-TECH GmbH. Silica gel (0.041–0.0631) for flash column chromatography was purchased from Merck. AAL, AAL555, L-fucose and DAPI-containing hardening mounting oil were purchased from Vector Labs. Sodium pyruvate, glucose, Hepes, glutamine, paraformaldehyde and NaAz were purchased from Invitrogen. CDCl3 and TMS for NMR experiments were provided by Euriso-top (France). The human colorectal adenocarcinoma cell line (DLD-1) was purchased from American Type Culture Collection (ATTC). 3[4,5-Dimethylthiazol-2-yl]-5-(3-carboxymethoxyphenyl)-2-(4-sulfophenyl)2H-tetrazolium, inner salt (MTS) and phenazine ethosulfate (PES) were provided by CellTiter. Aqueous one solution cell proliferation assay kit was purchased from Promega, UK. Cell dissociation solution, cell culture RPMI-1640 medium and streptomycin/penicillin solution were provided by Sigma. Fetal bovine serum (FBS) and fetal calf serum were provided by Lonza. EGF and HBBS (without Ca or Mg) were provided by Gibco. bFGF was purchased from Invitrogen. All chemicals were of reagent grade and used without further purification. Solvents were dried using standard procedures and reactions requiring anhydrous conditions were performed under a nitrogen atmosphere. Thin layer chromatography was carried out on 0.25 mm pre-coated silica gel plates (Merck silica gel 60 F254) with detection by UV-light (254 nm) and/or heating with 10% sulfuric acid (aqueous solution) or p-anisaldehyde solution. Flash chromatography was carried out using the SP1 Flash Purification System by Biotage.
Techniques
PXRD, TEM, isothermal N2 adsorption–desorption, DLS, FTIR spectroscopy and TGA were used to characterize the synthesized materials. PXRD measurements were performed on a Bruker AXS D8 Advance diffractometer using Cu-Kα radiation. TEM images were acquired with a JEOL TEM-1010 electron microscope working at 100 kV. N2 adsorption–desorption isotherms were recorded on a Micromeritics TriStar II sorption analyzer. The samples were degassed overnight at 120 °C under vacuum. The specific surface areas were calculated from adsorption data within the low pressure range, using the BET model. The pore size was determined by the BJH method. DLS measurements were performed on a ZetaSizer Nano instrument from Malvern. FTIR spectroscopy was performed with a Tensor 27 FT-IR spectrometer from Bruker. TGA was carried out on a TGA/SDTA 851e Mettler Toledo, under an oxidant atmosphere (air, 80 mL min−1), with a heating program consisting of a heating ramp of 10 °C per minute from 393 K to 1273 K and an isothermal heating step at this temperature for 30 minutes. Fluorescence measurements were carried out in a JASCO FP-8500 Spectrophotometer. 1H-NMR spectra were recorded in CDCl3 at 300 K on a Bruker AVANCE 400 MHz using TMS as the internal reference for chemical shifts. Mass spectra were obtained with an ESI apparatus Bruker Esquire 3000 plus.
Synthesis of MSNs
MSNs were synthesized using CTAB as porogen species and TMOS as a silicon source. In brief, 3.94 g of CTAB was dissolved in a solution containing 800 g of methanol/water (0.4/0.6 = w/w) and 2.8 mL of an aqueous solution of 1 M NaOH, under vigorous stirring. Once dissolved, 1.3 mL of TMOS was added under ambient conditions. After being stirred for 8 h, the mixture was aged overnight at rest. The resulting white precipitate was washed with ethanol and water five times each and finally dried at 70 °C to obtain the as-made mesostructured nanoparticles. Hence, the obtained solid was suspended in a solution containing 20 mL of ethanol and 4 mL of HCl. The suspension was refluxed overnight to extract the surfactant template. The resulting white solid was filtered out and thoroughly washed with ethanol and water and dried under vacuum to obtain the MSNs.
Synthesis of the trisaccharide Lex derivative (1)
The Lex derivative was obtained by following the established procedure described in the literature51,52 and in Fig. 2. A step-wise glycosylation strategy of the adequate building blocks was used. The attachment of the fucosyl residue to the 3-hydroxy group of the glucosamine moiety was followed by the introduction of the galactosyl residue to the 4-hydroxyl group of the disaccharide obtained in the first glycosidation. The obtained trisaccharide was deprotected, purified and characterized by 1H-NMR and mass spectrometry. 1H-NMR (500 MHz, D2O, 300 K): δ 1.16 ppm (d, 3H, J = 6.6 Hz, CH3 Fuc), 2.02 ppm (s, 3H, OCOCH3), 2.94 ppm (m, 2H, –CH2–NH2), 3.51 ppm (dd, 1H, J = 7.4, 3.9 Hz), 3.61 ppm (m, 2H), 3.78–3.64 ppm (m, 5H), 3.80 ppm (d, 1H, J = 2.4 Hz), 3.98–3.84 ppm (m, 7H), 4.02 ppm (m, 1H), 4.44 ppm (d, 1H, J = 7.8 Hz, H1′′Gal), 4.55 (d, 1H, J = 7.8 Hz, H1′ Glc), 4.82 ppm (m, 1H, H5 Fuc), 5.10 ppm (d, 1H, J = 3.9 Hz, H1 Fuc) (see the complete assignment and the NMR spectra in ESI, Fig. S6–S8 and Tables S1 and S2†). Mass spectrometry data identified the following ions with a m/z ratio of 573.55 (M + H)+ and 287.28 (M + 2H)2+.
Synthesis of S1
50 mg of MSNs was suspended in 500 μL of an aqueous solution of ATTO 430LS dye (500 μg). The suspension was kept overnight under stirring at room temperature, to achieve maximum loading in the pores of the MSN scaffolding. Then, the solid was filtered and dried under vacuum. Subsequently, 30 mg of the dry solid was suspended in 900 μL of acetonitrile and 8 μL of (3-isocyanatopropyl)triethoxysilane was added. The mixture was stirred at room temperature for 5.5 h. Finally, the solid was filtered and dried under vacuum to obtain the solid S1 (Fig. 1a).
Synthesis of S2
25 mg of the isocyanate-functionalized nanoparticles S1 and 2 mg of the Lex derivative 1 were suspended in 1 mL of deionized water. Immediately, 1 μL of triethylamine (0.003 mmol) was added as a catalyst and the suspension was stirred for 2 hours. The nanoparticles were then centrifuged and washed, and the resulting Lex-derivatized nanoparticles S2 were finally dried under vacuum (Fig. 1a).
Synthesis of S3
1000 μg of solid S2 was suspended in 980 μL of PBS buffer (10 mM, pH 7.4, supplemented with 1 mM calcium chloride and 2 mM magnesium chloride) and then, 20 μL of AAL aqueous solution (1.241 μg μL−1) was added. The suspension was kept stirring for 1 hour at room temperature. After that time, the solid was centrifuged, washed 5 times with buffer solution and dried under vacuum to obtain the final AAL-gated solid S3 (Fig. 1a).
Delivery studies in solution
Delivery studies of ATTO 430LS dye were performed in solution with S3, in the presence and in the absence of L-fucose. In a typical experiment, 500 μg of S3 was suspended in 1000 μL of deionized water. Then, 490 μL of the suspension was mixed with 510 μL of PBS buffer (10 mM, pH 7.4) and used as a control. 490 μL of the same suspension was mixed with 510 μL of a solution of L-fucose (5 M) in PBS buffer. In both cases, the suspensions were stirred at room temperature and aliquots (130 μL) were taken at scheduled times (0, 2, 5, 10, 30, and 60 min, respectively). The aliquots were then centrifuged to remove the solid and the amount of ATTO 430LS dye delivered was measured by fluorescence spectroscopy (λex: 433 nm, λem: 547 nm). To assess the performance in competitive media, delivery experiments were also carried out in cellular medium in the presence and in the absence of L-fucose. Solid S3 (0.25 mg mL−1) was incubated for 24 h at room temperature, in the presence and in the absence of 2.5 M L-fucose in DLD-1 complete media. The amount of delivered ATTO 430LS dye in the supernatant solution was measured by fluorescence spectroscopy (λex: 433 nm, λem: 547 nm).
Experiments with cells
The human colorectal adenocarcinoma cell line DLD-1 was grown in RPMI-1640 medium supplemented with 10% fetal calf serum, 1 mM sodium pyruvate, 4.5 g L−1 glucose, 10 mM HEPES, 2 mM L-glutamine and streptomycin/penicillin (100 U mL−1 penicillin and 100 μg mL−1 streptomycin) solution. Cells were cultured at 37 °C and 5% CO2 in 25 cc TC flasks. Fluorescence microscopy was used to assess the presence of AAL-binding ligands on the DLD-1 plasma membrane and to test the interaction with AAL. Round coverslips (13 mm-diameter) were deposited into 24-well plates and 2 × 105 DLD-1 cells were seeded in each well. Following overnight culture, the cell monolayers were rinsed with PBS and media containing 40 μg mL−1 fluorescent AAL (AAL555) or media alone was added to the wells. Following a 2 h incubation at 37 °C and 5% CO2, cells were washed carefully, not to lift cells off the coverslip, and fixed with PBS containing 1% paraformaldehyde for 30 min at 37 °C. The wells were then extensively washed and the cover slips mounted with DAPI-containing hardening mounting oil. Following a 30 min-incubation of the slides at 4 °C the cells were imaged using the 10× magnification objective of a Leica DMI6000b microscope (λex 560/40 nm, 595 nm dichromatic mirror, λem 645/75 nm) with a Leica DFC300FX color camera.
Cell viability experiments
Cell viability was measured with the colorimetric MTS method. The MTS assay is based on the conversion of a tetrazolium salt into a colored, aqueous soluble formazan product by the mitochondrial activity of viable cells at 37 °C. The amount of formazan produced by dehydrogenase enzymes is directly proportional to the number of living cells in culture and can be measured at 492 nm.54,55 104 DLD-1 cells were seeded in 96-well plates and grown for 24 h at 37 °C and 5% CO2. The DLD-1 cells were incubated with media containing 1, 5 or 10 μg mL−1 of solid S3 (50 μL) or media alone for 24 h at 37 °C and 5% CO2. Experiments were carried out in quadruplets. 1 h prior to absorbance measurement, the media were replaced by fresh media containing 1
:
20 (v
:
v) 3[4,5-dimethylthiazol-2-yl]-5-(3-carboxymethoxyphenyl)-2-(4-sulfophenyl)2H-tetrazolium, inner salt (MTS) and phenazine ethosulfate (PES) and incubated at 37 °C and 5% CO2. The absorbance of the MTS product was detected in a microplate reader (GENios Pro, TECAN) at 492 nm. The concentration of S3 in PBS employed for the cell assays was determined by inductively coupled plasma optical emission spectroscopy.
S3-cell interaction experiments
Confocal microscopy was used to assess S3-cell interactions. A Zeiss LSM 510 META confocal microscope was employed. DLD-1 cells were seeded in equal numbers per well and grown for 3 days in 16-well chamber slides (Lab Tek). After removal of the culture media, the DLD-1 cells were incubated with S3 (2.7 μg mL−1) in media for 4 h or 24 h at 37 °C and 5% CO2. Then the cell monolayers were or not washed with PBS for live cell imaging. Experiments were carried out in duplicates. The sample was excited at 488 nm and the emission light collected from 520–700 nm. The chamber slide was examined under the confocal microscope with a 10× objective in channel mode at the excitation wavelength of 488 nm for ATTO 430LS dye. The green color pixels were counted using the image analysis software Image J and the ratio to the total pixels was calculated.
Overexpression of Lex (and sLex)
DLD-1 cell surface expression of the fucose-containing carbohydrate structures Lex and sLex was tested by flow cytometry. The DLD-1 cells were grown for 3 or 7 days in the presence or absence of EGF (20 ng mL−1) and bFGF (10 ng mL−1). Cell monolayers were lifted from the flask surface by 15 min incubation at 37 °C with cell dissociation solution (Sigma) and centrifuged at 1500g for 5 min. The cell pellet was re-suspended in 1% FBS, 0.09% NaAz (staining buffer) containing additional 10% of heat inactivated FBS, syringe-disaggregated and left at room temperature for 30 min to block Fc receptors. Then, 20 μL of phycoerythrin (PE)-labelled anti-human Lex (CD15, clone HI98) or 10 μL BD Horizon™ BV711 anti-human sLex antibodies (CD15S, clone CSLEX1) were added and incubated in ice for 30 min. As control, isotype-matched fluorophore-matched antibodies were used (BD biosciences). Before analysis, the cells were washed twice with staining buffer by centrifugation, re-suspended in HBBS without calcium or magnesium containing 10 μg mL−1 DAPI and placed in ice. As DAPI is an exclusion dye, only cells negative for DAPI staining (live cells) were analyzed. Cell doublets were also excluded from the analysis. Experiments were acquired in a BD Canto II flow cytometer (Becton Dickinson) maintaining the same voltage settings for all the parameters analyzed. Analysis of the data was performed using the FlowJo program (Miltenyibiotec).
Colocalization studies
DLD-1 cells were grown for 3 days in 16-well chamber slides (Lab Tek) in the presence or absence of growth factors, then incubated with S3 (8 μg mL−1) in media for 24 h at 37 °C and 5% CO2 and cooled for 30 min at 4 °C. The cells were washed twice very carefully, drop-wise, to avoid lifting cells off the glass with cold PBS without calcium or magnesium containing 0.09% sodium azide (PBS/NaAz) and incubated with 30 μg mL−1 FM-464 in PBS/NaAz for 1 min at 4 °C for membrane staining. Medium was removed and the cells were fixed by incubation at 4 °C with 4% paraformaldehyde-containing PBS/NaAz for 30 min. Then the chamber gasket was removed from the slide and the cells were rinsed twice with PBS. Experiments were carried out in duplicates. A drop of DAPI-containing mounting oil was added to each cell spot and the slide was mounted with a coverslip of 0.13–0.16 mm (25 × 60 mm, Menzel-Glaser). The oil in the mounted slide was allowed to harden by a 30 min incubation at 4 °C, and the slide was examined under a Zeiss LSM 510 META confocal microscope with a 40× oil immersion objective in a lambda or channel mode at the excitation wavelengths of 488 nm and 514 nm for ATTO 430LS dye and FM4-64 (Life Technologies), respectively. Z-Stacks of 2 μm thickness planes were taken under the described conditions. Linear unmixing of the overlapping two spectra was performed and the resulting image was overlaid with the image of the transmitted light channel and the DAPI stain channel (excitation at 405 nm/emission filter 420–480 nm). For the overlays the AxioVision image program was employed.
Conflicts of interest
There are no conflicts to declare.
Acknowledgements
The authors thank the Spanish Government (Projects MAT2015-64139-C4-1-R and MAT2013-46101-R (MINECO/FEDER)), Fondo de Investigación Sanitaria (PI15/00480) and Generalitat Valenciana (Project PROMETEOII/2014/047 and project GVA/2014/13) for support. R. B. is thankful to Svagata.Eu (Erasmus Mundus Action-II program) for his fellowship. The authors also thank the Electron Microscopy Service at the UPV for support.
Notes and references
- C. Argyo, V. Weiss, C. Bräuchle and T. Bein, Chem. Mater., 2014, 26, 435–451 CrossRef CAS.
- E. Aznar, R. Martínez-Máñez and F. Sancenón, Expert Opin. Drug Delivery, 2009, 6, 643–655 CrossRef CAS PubMed.
- E. Aznar, M. Oroval, L. Pascual, J. R. Murguía, R. Martínez-Máñez and F. Sancenón, Chem. Rev., 2016, 116, 561–718 CrossRef CAS PubMed.
- X. Wang, L.-L. Tan, X. Li, N. Song, Z. Li, J.-N. Hu, Y.-M. Cheng, Y. Wang, Y.-W. Yang, Y.-W. Yang, N. A. Kotov, L. M. Liz-Marzán, H. Mattoussi, P. Mulvaney, C. B. Murray, A. L. Rogach, P. S. Weiss, I. Willner and W. J. Parak, Chem. Commun., 2016, 52, 13775–13778 RSC.
- X. Chen, H. Sun, J. Hu, X. Han, H. Liu and Y. Hu, Colloids Surf., B, 2017, 152, 77–84 CrossRef CAS PubMed.
- R. Prasad, S. Aiyer, D. S. Chauhan, R. Srivastava and K. Selvaraj, Nanoscale, 2016, 8, 4537–4546 RSC.
- A. Agostini, L. Mondragón, C. Coll, E. Aznar, M. D. Marcos, R. Martínez-Máñez, F. Sancenón, J. Soto, E. Pérez-Payá and P. Amorós, ChemistryOpen, 2012, 1, 17–20 CrossRef CAS PubMed.
- A. García-Fernández, G. García-Laínez, M. L. Ferrándiz, E. Aznar, F. Sancenón, M. J. Alcaraz, J. R. Murguía, M. D. Marcos, R. Martínez-Máñez, A. M. Costero and M. Orzáez, J. Controlled Release, 2017, 248, 60–70 CrossRef PubMed.
- A. Ultimo, C. Giménez, P. Bartovsky, E. Aznar, F. Sancenón, M. D. Marcos, P. Amorós, A. R. Bernardo, R. Martínez-Máñez, A. M. Jiménez-Lara and J. R. Murguía, Chem. – Eur. J., 2016, 22, 1582–1586 CrossRef CAS PubMed.
- L. Polo, N. Gómez-Cerezo, E. Aznar, J.-L. Vivancos, F. Sancenón, D. Arcos, M. Vallet-Regí and R. Martínez-Máñez, Acta Biomater., 2017, 50, 114–126 CrossRef CAS PubMed.
- Z. Luo, X. Ding, Y. Hu, S. Wu, Y. Xiang, Y. Zeng, B. Zhang, H. Yan, H. Zhang, L. Zhu, J. Liu, J. Li, K. Cai and Y. Zhao, ACS Nano, 2013, 7, 10271–10284 CrossRef CAS PubMed.
- Q. Zhang, K. Gee Neoh, L. Xu, S. Lu, E. Tang Kang, R. Mahendran and E. Chiong, Langmuir, 2014, 30, 6151–6161 CrossRef CAS PubMed.
- R. Guillet-Nicolas, A. Popat, J.-L. Bridot, G. Monteith, S. Z. Qiao and F. Kleitz, Angew. Chem., Int. Ed., 2013, 52, 2318–2322 CrossRef CAS PubMed.
- E. Bringas, Ö. Köysüren, D. V. Quach, M. Mahmoudi, E. Aznar, J. D. Roehling, M. D. Marcos, R. Martínez-Máñez, P. Stroeve and D. S. Kohane, Chem. Commun., 2012, 48, 5647–5649 RSC.
- M. Oroval, E. Climent, C. Coll, R. Eritja, A. Aviñó, M. D. Marcos, F. Sancenón, R. Martínez-Máñez and P. Amorós, Chem. Commun., 2013, 49, 5480–5482 RSC.
- D. He, X. He, K. Wang, M. Chen, Y. Zhao, Z. Zou, J. Shi, Y. Kondo, R. Sawa, T. Fujimoto, T. Machinami and A. Ono, J. Mater. Chem. B, 2013, 1, 1552–1560 RSC.
- L. Chen, X. Zhou, W. Nie, Q. Zhang, W. Wang, Y. Zhang and C. He, ACS Appl. Mater. Interfaces, 2016, 8, 33829–33841 CAS.
- J. G. Croissant, D. Zhang, S. Alsaiari, J. Lu, L. Deng, F. Tamanoi, A. M. AlMalik, J. I. Zink and N. M. Khashab, J. Controlled Release, 2016, 229, 183–191 CrossRef CAS PubMed.
- M. Oroval, P. Díez, E. Aznar, C. Coll, M. D. Marcos, F. Sancenón, R. Villalonga and R. Martínez-Máñez, Chem. – Eur. J., 2017, 23, 1353–1360 CrossRef CAS PubMed.
- C. Chen, J. Geng, F. Pu, X. Yang, J. Ren and X. Qu, Angew. Chem., Int. Ed., 2011, 50, 882–886 CrossRef CAS PubMed.
- X. Yang, X. Liu, Z. Liu, F. Pu, J. Ren and X. Qu, Adv. Mater., 2012, 24, 2890–2895 CrossRef CAS PubMed.
- Z. Deng, Z. Zhen, X. Hu, S. Wu, Z. Xu and P. K. Chu, Biomaterials, 2011, 32, 4976–4986 CrossRef CAS PubMed.
- L. Palanikumar, E. S. Choi, J. Y. Cheon, S. H. Joo and J.-H. Ryu, Adv. Funct. Mater., 2015, 25, 957–965 CrossRef CAS.
- L.-L. Li, M. Xie, J. Wang, X. Li, C. Wang, Q. Yuan, D.-W. Pang, Y. Lu and W. Tan, Chem. Commun., 2013, 49, 5823–5825 RSC.
- I. Häuselmann and L. Borsig, Front. Oncol., 2014, 4, 28 Search PubMed.
- J. B. Haltiwanger and R. S. Lowe, Annu. Rev. Biochem., 2004, 73, 491–537 CrossRef PubMed.
-
A. Varki, R. Kannagi and B. P. Toole, Glycosylation Changes in Cancer, Cold Spring Harbor Laboratory Press, 2009 Search PubMed.
-
A. Varki and J. B. Lowe, Biological Roles of Glycans, Cold Spring Harbor Laboratory Press, 2009 Search PubMed.
- M. Gary-Bobo, O. Hocine, D. Brevet, M. Maynadier, L. Raehm, S. Richeter, V. Charasson, B. Loock, A. Morère, P. Maillard, M. Garcia and J. O. Durand, Int. J. Pharm., 2012, 423, 509–515 CrossRef CAS PubMed.
- D. Brevet, M. Gary-Bobo, L. Raehm, S. Richeter, O. Hocine, K. Amro, B. Loock, P. Couleaud, C. Frochot, A. Morère, P. Maillard, M. Garcia and J.-O. Durand, Chem. Commun., 2009, 28, 1475–1477 RSC.
- O. Hocine, M. Gary-Bobo, D. Brevet, M. Maynadier, S. Fontanel, L. Raehm, S. Richeter, B. Loock, P. Couleaud, C. Frochot, C. Charnay, G. Derrien, M. Smaïhi, A. Sahmoune, A. Morère, P. Maillard, M. Garcia and J. O. Durand, Int. J. Pharm., 2010, 402, 221–230 CrossRef CAS PubMed.
- I. Y. Park, I. Y. Kim, M. K. Yoo, Y. J. Choi, M. H. Cho and C. S. Cho, Int. J. Pharm., 2008, 359, 280–287 CrossRef CAS PubMed.
- Z. Luo, K. Cai, Y. Hu, L. Zhao, P. Liu, L. Duan and W. Yang, Angew. Chem., Int. Ed., 2011, 50, 640–643 CrossRef CAS PubMed.
- J. Peng, K. Wang, W. Tan, X. He, C. He, P. Wu and F. Liu, Talanta, 2007, 71, 833–840 CrossRef CAS PubMed.
- M. Yu, S. Jambhrunkar, P. Thorn, J. Chen, W. Gu and C. Yu, Nanoscale, 2013, 5, 178–183 RSC.
- Q. He, M. Ma, C. Wei and J. Shi, Biomaterials, 2012, 33, 4392–4402 CrossRef CAS PubMed.
- S. Wu, X. Huang and X. Du, Angew. Chem., Int. Ed., 2013, 52, 5580–5584 CrossRef CAS PubMed.
- J. Li, X. Qu, G. F. Payne, C. Zhang, Y. Zhang, J. Li, J. Ren, H. Hong and C. Liu, Adv. Funct. Mater., 2015, 25, 1404–1417 CrossRef CAS.
- J. Burchell, R. Poulsom, A. Hanby, C. Whitehouse, L. Cooper, H. Clausen, D. Miles and J. Taylor-Papadimitriou, Glycobiology, 1999, 9, 1307–1311 CrossRef CAS PubMed.
- S. S. Pinho, C. A. Reis, J. Paredes, A. M. Magalhães, A. C. Ferreira, J. Figueiredo, W. Xiaogang, F. Carneiro, F. Gärtner and R. Seruca, Hum. Mol. Genet., 2009, 18, 2599–2608 CrossRef CAS PubMed.
- M. Takahashi, Y. Kuroki, K. Ohtsubo and N. Taniguchi, Carbohydr. Res., 2009, 344, 1387–1390 CrossRef CAS PubMed.
- M. Li, L. Song and X. Qin, J. Biosci., 2010, 35, 665–673 CrossRef CAS PubMed.
- K. Nagao, Y. Itoh, K. Fujita and M. Fujime, Int. J. Urol., 2007, 14, 795–799 CrossRef CAS PubMed.
- W. Gao, J. Liang and Y. Liang, OncoTargets Ther., 2016, 9, 3113–3125 CrossRef PubMed.
- P. Sozzani, R. Arisio, M. Porpiglia and C. Benedetto, Int. J. Surg. Pathol., 2008, 16, 365–374 CrossRef PubMed.
- A. Yusa, K. Miyazaki, N. Kimura, M. Izawa and R. Kannagi, Cancer Res., 2010, 70, 4064–4073 CrossRef CAS PubMed.
- A. Takada, K. Ohmori, T. Yoneda, K. Tsuyuoka, A. Hasegawa, M. Kiso and R. Kannagi, Cancer Res., 1993, 53, 354–361 CAS.
- D. Golijanin, Y. Sherman, A. Shapiro and D. Pode, Urology, 1995, 46, 173–177 CrossRef CAS PubMed.
- A. Hittelet, I. Camby, N. Nagy, H. Legendre, Y. Bronckart, C. Decaestecker, H. Kaltner, N. E. Nifant'ev, N. V. Bovin, J. Pector, I. Salmon, H. Gabius, R. Kiss and P. Yeaton, Lab. Invest., 2003, 83, 777–787 CrossRef CAS PubMed.
- C. de la Torre, I. Casanova, G. Acosta, C. Coll, M. J. Moreno, F. Albericio, E. Aznar, R. Mangues, M. Royo, F. Sancenón and R. Martínez-Máñez, Adv. Funct. Mater., 2015, 25, 687–695 CrossRef CAS.
- J. M. De la Fuente and S. Penadés, Tetrahedron: Asymmetry, 2002, 13, 1879–1888 CrossRef CAS.
- T. Zhu and G.-J. Boons, J. Am. Chem. Soc., 2000, 122, 10222–10223 CrossRef CAS.
- O. Martínez-Ávila, K. Hijazi, M. Marradi, C. Clavel, C. Campion, C. Kelly and S. Penadés, Chem. – Eur. J., 2009, 15, 9874–9888 CrossRef PubMed.
- A. H. Cory, T. C. Owen, J. A. Barltrop and J. G. Cory, Cancer Commun., 1991, 3, 207–212 CAS.
- G. Malich, B. Markovic and C. Winder, Toxicology, 1997, 124, 179–192 CrossRef CAS PubMed.
- K. Sakuma, M. Aoki and R. Kannagi, Proc. Natl. Acad. Sci. U. S. A., 2012, 109, 7776–7781 CrossRef CAS PubMed.
Footnote |
† Electronic supplementary information (ESI) available: PXRD patterns of the as-synthesized MSNs and extracted MSNs; TEM images of MSNs and solid S3; N2 adsorption–desorption isotherms for MSNs and pore size distribution; and the release profile of ATTO 430LS dye from solid S3 in cellular medium. See DOI: 10.1039/c7nr06415b |
|
This journal is © The Royal Society of Chemistry 2018 |