DOI:
10.1039/C7NJ02102J
(Paper)
New J. Chem., 2017,
41, 14259-14265
Determination of molar refractions and Abraham descriptors for tris(acetylacetonato)chromium(III), tris(acetylacetonato)iron(III) and tris(acetylacetonato)cobalt(III)†
Received
12th June 2017
, Accepted 20th October 2017
First published on 23rd October 2017
Abstract
We have determined molar refractions of tris(acetylacetonato)chromium(III), tris(acetylacetonato)iron(III) and tris(acetylacetonato)cobalt(III). Although the d-electron structures of the three metal centres differ significantly, the three molar refractions are actually quite close to each other. We then used these molar refractions to determine the Abraham E-descriptor, we calculated the V-descriptor by McGowan's method, and then used literature data on solubilities and water–solvent partitions to obtain the rest of the set of descriptors for the three tris(acetylacetonato) complexes. If we take E as the average of those for the chromium, iron and cobalt complexes, we can use limited literature data to obtain the full set of Abraham descriptors for the tris(acetylacetonates) of vanadium(III), yttrium(III), samarium(III), lanthanum(III) and neodymium(III). For the eight complexes, the descriptors vary regularly with complex molecular weight. These show that the complexes are quite polarizable, have zero hydrogen-bond acidity and significant hydrogen bond basicity. From the sets of Abraham descriptors, a very large number of physicochemical properties can be predicted for the eight acetonylacetonates.
Introduction
Methods are now available for the calculation or prediction of numerous physico-chemical properties of organic compounds. Water–octanol partition coefficients can easily be calculated through programs such as BioLoom,1 the EPI Suite TM,2 ACD ChemSketch,3 the ACD Absolv suite4 and SPARC.5 Some of these programs2,5 can be used to calculate numerous other physico-chemical properties. However, extension to compounds other than organic compounds is either very limited or non-existent. We have developed a system of properties or ‘descriptors’ of solute molecules, known as Abraham descriptors or Absolv descriptors.6–13 These descriptors, together with a large set of equations we have constructed enables predictions to be made of all sorts of physicochemical, environmental and biological properties. Initially the system was applied to organic compounds and to a few simple inorganic compounds, but we have since applied it to organometallic compounds such mercury compounds14 and the tetraphenyl derivatives of silicon, germanium, tin and lead.15 Recently we showed that the system could include derivatives of ferrocene.16 This suggests that we might be able to include inorganic complexes into our system. If so, this would mark a very significant extension of predictive methods into the vast area of inorganic complex chemistry. We start with the tris(acetylacetonato) complexes of chromium(III), cobalt(III) and iron(III) because there was a reasonable amount of data on the solubilities of these complexes that we could use. We refer to the complexes as Cr(acac)3, Co(acac)3 and Fe(acac)3. One of the descriptors we need can be obtained from the refractive index or molar refractivity of a compound. No such data were available for the three tris(acetylacetonato) complexes, and so we decided to determine their molar refractions experimentally.
Experimental
Compounds
The three tris(acetylacetonato) complexes, were purchased from Sigma-Aldrich. The purity of the iron compound was ≥99.9%, while that of the chromium and cobalt was 99.99%.
Solutions
For each series of measurements, the pure solvent and ten solutions of the metal compound in 200 proof ethyl alcohol, ACS/USP grade, were used. The solutions ranged from 20 mg of compound in 45 mL of solvent up to 200 mg, in 20 mg increments. The iron compound, a fine powder, dissolved readily and yielded a highly colored red solution. The chromium and cobalt compounds, in crystalline form, required sonication to completely dissolve the higher concentration solutions to give violet and green solutions, respectively.
Measurements
Density and refractive index were measured at 20.00 ± 0.01 °C with an Anton Paar DMA 4500 density meter mated to an Anton Paar RXA 170 refractometer through plastic tubing. The techniques, calibration, accuracy, and precision are given elsewhere.17 The ranges for the density and refractive index are 0.79101 to 0.79639 kg L−1 and 1.36135 to 1.36290, respectively. These ranges fall well within the manufacturer's recommendations for the calibration methods used here. The system was calibrated before each series of measurements with deionized water at 20 °C. For each case a value of 0.99821 g mL−1 were measured and agree with the accepted value for water at 20 °C of 0.998206 g mL−1 (one additional significant figure).
Data treatment
Table 1 reports the mole fractions, densities, and refractive index values for the ethanolic solutions of the complexes. The molar volumes, VM, for each solution were calculated from | 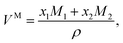 | (1) |
where xi and Mi are the mole fractions and molar masses of the solutions’ components, respectively, and where i = 1, 2 refer to the solvent and solute, respectively. The molar refractions of the solutions, R, were calculated from the molar volumes and refractive indexes (n) according to | 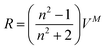 | (2) |
Table 1
x
2, ρ(g cm−3), n, VM (cm3 mol−1), and R (cm3 mol−1) for ethanolic solutions of M(acac)3 [M = Cr, Fe, and Co]
x
2
|
ρ
|
n
|
V
M
|
R
|
M = Cr |
7.63 × 10−5 |
0.79119 |
1.36145 |
58.25799 |
12.90393 |
0.000152 |
0.79137 |
1.36154 |
58.27361 |
12.91027 |
0.000223 |
0.79151 |
1.36163 |
58.29077 |
12.91696 |
0.000299 |
0.79167 |
1.36171 |
58.30788 |
12.92332 |
0.0003755 |
0.79193 |
1.36180 |
58.31811 |
12.92847 |
0.0004413 |
0.79205 |
1.36187 |
58.33449 |
12.93435 |
0.0005191 |
0.79220 |
1.36197 |
58.35320 |
12.94171 |
0.0005937 |
0.79239 |
1.36206 |
58.36778 |
12.94783 |
0.0006703 |
0.79253 |
1.36214 |
58.38679 |
12.95461 |
0.0007446 |
0.79271 |
1.36222 |
58.40192 |
12.96054 |
|
M = Fe |
7.46 × 10−5 |
0.79119 |
1.36154 |
58.25771 |
12.90675 |
0.000153 |
0.79136 |
1.36163 |
58.27570 |
12.91362 |
0.000222 |
0.79153 |
1.36173 |
58.29005 |
12.92001 |
0.000294 |
0.79170 |
1.36182 |
58.30533 |
12.92628 |
0.000370 |
0.79187 |
1.36192 |
58.32239 |
12.93327 |
0.0004486 |
0.79202 |
1.36202 |
58.34165 |
12.94075 |
0.0005131 |
0.79220 |
1.36211 |
58.35342 |
12.94625 |
0.0005826 |
0.79236 |
1.36219 |
58.36858 |
12.95218 |
0.0006546 |
0.79254 |
1.36229 |
58.38320 |
12.95863 |
0.0007446 |
0.79272 |
1.36238 |
58.40482 |
12.96632 |
|
M = Co |
7.386 × 10−5 |
0.79116 |
1.36144 |
58.25991 |
12.90403 |
0.000142 |
0.79142 |
1.36154 |
58.26738 |
12.90889 |
0.000219 |
0.79168 |
1.36165 |
58.27835 |
12.91485 |
0.000296 |
0.79188 |
1.36175 |
58.29407 |
12.92154 |
0.000364 |
0.79201 |
1.36184 |
58.31115 |
12.92821 |
0.0004389 |
0.79213 |
1.36194 |
58.33153 |
12.93594 |
0.0005135 |
0.79231 |
1.36203 |
58.34747 |
12.94236 |
0.0005862 |
0.79252 |
1.36213 |
58.36045 |
12.94845 |
0.0006493 |
0.79265 |
1.36221 |
58.37558 |
12.95437 |
0.0007486 |
0.79290 |
1.36233 |
58.39603 |
12.96276 |
The VM and R-values for each solution are also given in Table 1. Since VM is a homogeneous function of n1 and n2, following Euler, one gets
| VM = x1VM1 + x2VM2 = VM1 + x2(VM2 − VM1), | (3) |
where
VM1 and
VM2 are the partial molar volumes for the solvent and solute, respectively. An analogous equation obtains for molar refraction (
R).
18 All
VM and
R-values exhibit nearly linear dependence on the mole fraction of solute. Consequently, intercepts and slopes were calculated employing Excel's linear regression routine, and standard deviations were determined from Excel's ANOVA routine. The refractive index (
n2) for each complex was calculated according to
| 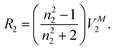 | (4) |
Descriptor methodology
Our method for the determination of descriptors for neutral solutes uses two linear free energy relationships, eqn (5) and (6). | log SP = c + eE + sS + aA + bB + vV | (5) |
| log SP = c + eE + sS + aA + bB + lL | (6) |
Eqn (5) is used when the dependent variable, log
SP, refers to condensed phase processes, such as the water–solvent partition coefficient for a series of solutes in a given system; then SP itself is the water–solvent partition coefficient. Eqn (6) is used when log
SP refers to a gas to system partition, where SP is the gas to system partition coefficient.
The independent variables in eqn (5) and (6) are solute descriptors as follows:6–13E is the solute excess molar refractivity in units of (cm3 mol−1)/10, S is the solute dipolarity/polarizability, A and B are the overall or summation hydrogen bond acidity and basicity, V is the McGowan characteristic volume in units of (cm3 mol−1)/100 and L is the logarithm of the gas-hexadecane partition coefficient, at 298 K. The coefficients in eqn (5) and (6) are obtained by multiple linear regression analysis, and serve to characterize the system under consideration.
In order to apply eqn (5) or (6), values of the dependent variable are needed. The most direct source is a directly determined water–solvent partition coefficient, P, as log
P. However, most of the relevant data consists of solubilities in (dry) solvents and in water. Then partition coefficients can be obtained indirectly through eqn (7), where Cw and Cs are solubilities in mol dm−3, in water and a given solvent. If a value of Cw is not known, or is perhaps doubtful, then log
Cw can be allowed to float, and becomes another unknown parameter to deduce.
We can greatly increase the number of simultaneous equations, by converting every log
P value from
eqn (7) into a corresponding log
Ks value through
eqn (8), where
Ks is the gas to solvent partition coefficient and
Kw is the gas to water partition coefficient. Now log
Kw itself is another variable to be determined.
This leaves
E,
S,
A,
B,
V,
L, and possibly log
Cw and log
Kw to be determined through a set of simultaneous equations with log
P and log
Ks as the dependent variables in
eqn (5) and (6). The Microsoft ‘Solver’ add-on is particularly useful, and any number of simultaneous equations can be solved to give a ‘best-fit’ solution.
Since we have as many as eight variables to obtain, it is useful to be able to deduce one or more variables independently, and so reduce the number that have to be obtained through the set of simultaneous equations. For organic compounds, E can be calculated through two programs4,19 and can also be obtained from a calculated liquid refractive index at 293 K.3 Now that we have experimental molar refractions there is no problem in obtaining values of E.
The volume descriptor, V, can very easily be calculated for organic compounds through McGowan's method,20 but as we showed in the case of ferrocene, it is not straightforward to use McGowan's method for inorganic complexes.
The McGowan volume, Vx = 100 × V, is calculated from atomic increments, as shown in Table 2,16,20,21 with 6.56 subtracted for each bond (single, double and triple bonds all counting as one bond). If the structure of an acac derivative of cobalt(III) is as shown in Fig. 1, then for tris(acetylacetonato)Co(III) there are 15 × 3 = 45 bonds. Then with Vx for Co(3+) = 0.78 mL mol−1, for the complex we have Vx = 21 × 8.71 + 15 × 16.35 + 6 × 12.43 + 0.78 − 6.56 × 45 = 208.3 cm3 mol−1. However, the C
O → Co coordinate bond is not a ‘McGowan’ bond, and the oxygen atom in
O → has three bonds instead of two. So there is a difficulty in calculating the McGowan volume for the cyclic structure.
Table 2 Atomic volumes, Vx, in cm3 mol−1
16,20,21
H 8.71 |
|
|
|
|
|
C 16.35 |
N 14.39 |
O 12.43 |
F 10.48 |
Fe3+ 0.78 |
Y3+ 3.58 |
Si 26.83 |
P 24.87 |
S 22.91 |
Cl 20.95 |
Co3+ 0.78 |
Sm3+ 3.66 |
Ge 31.02 |
As 29.42 |
Se 27.81 |
Br 26.21 |
Cr3+ 1.08 |
Nd3+ 3.89 |
Sn 39.35 |
Sb 37.74 |
Te 36.14 |
I 34.53 |
V3+ 1.18 |
La3+ 5.11 |
Pb 43.44 |
|
|
|
|
|
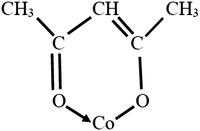 |
| Fig. 1 A possible configuration of Co(acac)3 for the calculation of McGowan's volume, illustrated for just one of the acac molecules. | |
Suppose we use the non-cyclic structure, Fig. 2. Now the number of bonds is 14 × 3 = 42, and Vx = 21 × 8.71 + 15 × 16.35 + 6 × 12.43 + 0.78 − 6.56 × 42 = 228.0 cm3 mol−1, substantially larger than that calculated for the cyclic structure.
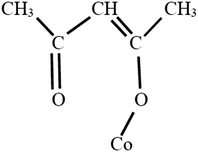 |
| Fig. 2 A possible ‘open chain’ configuration of Co(acac)3 for the calculation of McGowan's volume. | |
We can resolve this by using the equations we have constructed16 for the correlation of Vx against the partial molal volumes, VM2 (MeCN), of a series of organic and inorganic compounds in acetonitrile solvent,22eqn (5) and (6). For Co(acac)3 we have that VM2 (MeCN) = 256.6 cm3 mol−1 in acetonitrile,22 and so calculated values of Vx are 233.1 ± 6.2 on eqn (9) and 228.2 ± 7.9 on eqn (10), very close to the McGowan volume of 228.0 cm3 mol−1 as calculated for the non-cyclic structure in Fig. 2. Similar calculations for Cr(acac)3 and Fe(acac)3 confirm the use of the non-cyclic structure in the calculation of Vx. We make it clear that the structure in Fig. 2 is only for the purpose of calculating Vx and is not intended as the representation of the actual structure of Co(acac)3. However, now that we have shown that the structure in Fig. 2 can be used to calculate V for M(acac)3 complexes, we can use the same method to calculate V for complexes of various substituted acetylacetones.
| Vx = −10.237 + 0.9482VM2 (MeCN) N = 58, SD = 6.05, R2 = 0.993, F = 7531.6, PRESS = 2181.40, Q2 = 0.992, PSD = 6.24 | (9) |
| Vx = 0.8895VM2 (MeCN) N = 58, SD = 7.74, PRESS = 3534.0, PSD = 7.87 | (10) |
Results and discussion
Molar refractions
The molar volumes, molar refractions, and refractive indices are listed in Table 3. The molar volumes of the three tris-acac metal compounds follow the trend of Co < Fe ≈ Cr, which inversely follows the molecular masses of the three compounds. This observation is in accord with the results of crystal structures23–25 of the compounds in which the metal oxygen bond distances increase in roughly the same direction: Co 1.888 (4) Å, Cr 1.951 (7) Å, Fe 1.95 (1) Å. Assuming that the other bond (C–H, C–C, and C–O) distances within the acac ligand itself do not vary appreciably, then the cobalt compound would be expected to have the smallest volume.
Table 3
V
M2, R2, and n2 for M(acac)3 complexesa
M |
V
M2, cm3 mol−1 |
R
2, cm3 mol−1 |
n
2
|
Standard deviations in parentheses.
|
Cr |
272.9(2.9) |
97.6(0.7) |
1.6338 |
Fe |
276.3(1.4) |
102.3(0.4) |
1.6638 |
Co |
269.3(5.9) |
101.9(1.1) |
1.6809 |
The d-electron structures of the three metal centers also differ significantly with the chromium(III) and iron(III) centers being paramagnetic high-spin d3 and d5, respectively, while the cobalt(III) center is diamagnetic low-spin d6.
Descriptors
Water–solvent partition coefficients into water–methanol mixtures, dimethylsulfoxide (DMSO) and dioxane for Cr(acac)3 have been listed by Alousy and Burgess.26 Watarai et al.,27 have determined partitions into dodecane, tetrachloromethane and benzene. Solubilities of Cr(acac)3 are known in water–ethanol mixtures28 but only in those of low ethanol content, and we did not use any of these values. Solubilities are also known in the solvents dimethylformamide29,30 dichloromethane, 1,1,1-trichloroethane and tetrahydrofuran.30 The solubility of Cr(acac)3 in water at 298 K is given as log
Cw = −2.55,29 with Cw in mol dm−3, and so the various solubilities can be converted into values of log
P through eqn (7). Then if we take log
Kw as unknown to be determined, we can convert all the log
P values into log
Ks values through eqn (8). We can calculate Vx = 2.2830 by McGowan's method, and from the refractive index in Table 3 calculate that E = 2.222. This leaves the descriptors S, A, B, L and log
Kw to be obtained from a set of simultaneous equations in log
P. In Table 4 are the values of log
P that we used. A preliminary analysis showed that three values were out of line, leaving 14 values of log
P and 14 values of the corresponding log
Ks. We also had two equations in log
Kw giving a total of 30 simultaneous equations. The equation coefficients16,31–33 for eqn (5) and (6) are collected in Tables 5 and 6. The best fit solution of the 30 simultaneous equations yielded the descriptors shown in Table 7, and the calculated values of log
P from these descriptors are in Table 4.
Table 4 Calculated and observed values of water–solvent partition coefficients, as log
P, for Cr(acac)3
Solvent |
log P (calc) |
log P (obs) |
Ref. |
|
Dichloromethane |
2.988 |
2.500 |
30
|
Not used |
Tetrachloromethane |
1.975 |
2.041 |
27
|
|
Dodecane |
0.322 |
−0.733 |
27
|
Not used |
Benzene |
2.397 |
2.534 |
27
|
|
Tetrahydrofuran |
1.946 |
1.740 |
30
|
Not used |
Dioxane |
1.865 |
1.870 |
26
|
|
Dimethylfomamide |
1.915 |
1.934 |
30
|
|
Dimethylsulfoxide |
1.627 |
1.610 |
26
|
|
90% methanol–water |
1.729 |
1.600 |
26
|
|
80% methanol–water |
1.498 |
1.510 |
26
|
|
70% methanol–water |
1.288 |
1.350 |
26
|
|
60% methanol–water |
1.095 |
1.160 |
26
|
|
50% methanol–water |
0.902 |
0.860 |
26
|
|
40% methanol–water |
0.733 |
0.560 |
26
|
|
30% methanol–water |
0.536 |
0.330 |
26
|
|
20% methanol–water |
0.385 |
0.140 |
26
|
|
10% methanol–water |
0.211 |
0.070 |
26
|
|
Table 5 Coefficients in eqn (5) for water–solvent partitions as log
P
Solvent |
Coefficients |
c
|
e
|
s
|
a
|
b
|
v
|
Hexane |
0.333 |
0.560 |
−1.710 |
−3.578 |
−4.939 |
4.463 |
Heptane |
0.297 |
0.634 |
−1.755 |
−3.571 |
−4.946 |
4.488 |
Cyclohexane |
0.159 |
0.784 |
−1.678 |
−3.740 |
−4.929 |
4.577 |
Formamide |
−0.171 |
0.070 |
0.308 |
0.589 |
−3.152 |
2.432 |
Dimethylformamide |
−0.305 |
−0.058 |
0.343 |
0.358 |
−4.865 |
4.486 |
Dimethylacetamide |
−0.271 |
0.084 |
0.209 |
0.915 |
−5.003 |
4.557 |
Acetonitrile |
0.413 |
0.077 |
0.326 |
−1.566 |
−4.391 |
3.364 |
Nitromethane |
0.023 |
−0.091 |
0.793 |
−1.463 |
−4.364 |
3.460 |
Dimethylsulfoxide |
−0.194 |
0.327 |
0.791 |
1.260 |
−4.540 |
3.361 |
Propylene carbonate |
−0.149 |
0.754 |
−0.966 |
0.684 |
−3.134 |
3.247 |
Propanone |
0.313 |
0.312 |
−0.121 |
−0.608 |
−4.753 |
3.942 |
Tetrahydrofuran |
0.223 |
0.363 |
−0.384 |
−0.238 |
−4.932 |
4.450 |
1,2-Dichloroethane |
0.183 |
0.294 |
−0.134 |
−2.801 |
−4.291 |
4.180 |
Benzene |
0.142 |
0.464 |
−0.588 |
−3.099 |
−4.625 |
4.491 |
Toluene |
0.125 |
0.431 |
−0.644 |
−3.002 |
−4.748 |
4.524 |
Chlorobenzene |
0.065 |
0.381 |
−0.521 |
−3.183 |
−4.700 |
4.614 |
Nitrobenzene |
−0.152 |
0.525 |
0.081 |
−2.332 |
−4.494 |
4.187 |
Ethylene glycol |
−0.270 |
0.578 |
−0.511 |
0.715 |
−2.619 |
2.729 |
2-Ethoxyethanol |
0.133 |
0.392 |
−0.419 |
0.125 |
−4.200 |
3.888 |
2-Butoxyethanol |
−0.055 |
0.377 |
−0.607 |
−0.080 |
−4.371 |
4.234 |
Octan-1-ol, wet |
0.088 |
0.562 |
−1.054 |
0.034 |
−3.460 |
3.814 |
Ethanol |
0.222 |
0.471 |
−1.035 |
0.326 |
−3.596 |
3.857 |
96% ethanol |
0.238 |
0.353 |
−0.833 |
0.297 |
−3.533 |
3.724 |
95% ethanol |
0.239 |
0.328 |
−0.795 |
0.294 |
−3.514 |
3.697 |
90% ethanol |
0.243 |
0.213 |
−0.575 |
0.262 |
−3.450 |
3.545 |
80% ethanol |
0.172 |
0.175 |
−0.465 |
0.260 |
−3.212 |
3.323 |
70% ethanol |
0.063 |
0.085 |
−0.368 |
0.311 |
−2.936 |
3.102 |
60% ethanol |
−0.040 |
0.138 |
−0.335 |
0.293 |
−2.675 |
2.812 |
50% ethanol |
−0.142 |
0.124 |
−0.252 |
0.251 |
−2.275 |
2.415 |
40% ethanol |
−0.221 |
0.131 |
−0.159 |
0.171 |
−1.809 |
1.918 |
30% ethanol |
−0.269 |
0.107 |
−0.098 |
0.133 |
−1.316 |
1.414 |
20% ethanol |
−0.252 |
0.042 |
−0.040 |
0.096 |
−0.823 |
0.916 |
10% ethanol |
−0.173 |
−0.023 |
−0.001 |
0.065 |
−0.372 |
0.454 |
Methanol |
0.276 |
0.334 |
−0.714 |
0.243 |
−3.320 |
3.549 |
95% methanol |
0.270 |
0.278 |
−0.520 |
0.230 |
−3.368 |
3.365 |
90% methanol |
0.258 |
0.250 |
−0.452 |
0.229 |
−3.206 |
3.175 |
80% methanol |
0.172 |
0.197 |
−0.319 |
0.241 |
−2.912 |
2.842 |
70% methanol |
0.098 |
0.192 |
−0.260 |
0.266 |
−2.558 |
2.474 |
60% methanol |
0.053 |
0.207 |
−0.238 |
0.272 |
−2.157 |
2.073 |
50% methanol |
0.023 |
0.223 |
−0.222 |
0.264 |
−1.747 |
1.662 |
40% methanol |
0.020 |
0.222 |
−0.205 |
0.218 |
−1.329 |
1.259 |
30% methanol |
0.016 |
0.187 |
−0.172 |
0.165 |
−0.953 |
0.898 |
20% methanol |
0.022 |
0.142 |
−0.138 |
0.088 |
−0.574 |
0.559 |
10% methanol |
0.012 |
0.072 |
−0.081 |
0.026 |
−0.249 |
0.266 |
Table 6 Coefficients in eqn (6) for gas−solvent partitions as log
K
Solvent |
Coefficients |
c
|
e
|
s
|
a
|
b
|
l
|
Hexane |
0.320 |
0.000 |
0.000 |
0.000 |
0.000 |
0.945 |
Heptane |
0.284 |
0.000 |
0.000 |
0.000 |
0.000 |
0.950 |
Cyclohexane |
0.163 |
−0.110 |
0.000 |
0.000 |
0.000 |
1.013 |
Formamide |
−0.800 |
0.310 |
2.292 |
4.130 |
1.933 |
0.442 |
Dimethylformamide |
−0.391 |
−0.869 |
2.107 |
3.774 |
0.000 |
1.011 |
Dimethylacetamide |
−0.308 |
−0.736 |
1.802 |
4.361 |
0.000 |
1.028 |
Acetonitrile |
−0.007 |
−0.595 |
2.461 |
2.085 |
0.418 |
0.738 |
Nitromethane |
−0.340 |
−0.297 |
2.689 |
2.193 |
0.514 |
0.728 |
Dimethylsulfoxide |
−0.556 |
−0.223 |
2.903 |
5.037 |
0.000 |
0.719 |
Propylene carbonate |
−0.356 |
−0.413 |
2.587 |
2.207 |
0.455 |
0.719 |
Propanone |
0.127 |
−0.387 |
1.733 |
3.060 |
0.000 |
0.866 |
Tetrahydrofuran |
0.189 |
−0.347 |
1.238 |
3.289 |
0.000 |
0.982 |
1,2-Dichloroethane |
0.017 |
−0.337 |
1.600 |
0.774 |
0.637 |
0.921 |
Benzene |
0.107 |
−0.313 |
1.053 |
0.457 |
0.169 |
1.020 |
Toluene |
0.085 |
−0.400 |
1.063 |
0.501 |
0.154 |
1.011 |
Chlorobenzene |
0.064 |
−0.399 |
1.151 |
0.313 |
0.171 |
1.032 |
Nitrobenzene |
−0.296 |
0.092 |
1.707 |
1.147 |
0.443 |
0.912 |
2-Ethoxyethanol |
−0.064 |
−0.257 |
1.452 |
3.672 |
0.662 |
0.843 |
2-Butoxyethanol |
−0.109 |
−0.304 |
1.126 |
3.407 |
0.660 |
0.914 |
Octan-1-ol, wet |
−0.222 |
0.088 |
0.701 |
3.473 |
1.477 |
0.851 |
Ethanol |
0.222 |
0.471 |
−1.035 |
0.326 |
−3.596 |
3.857 |
96% ethanol |
0.238 |
0.353 |
−0.833 |
0.297 |
−3.533 |
3.724 |
95% ethanol |
0.239 |
0.328 |
−0.795 |
0.294 |
−3.514 |
3.697 |
90% ethanol |
0.243 |
0.213 |
−0.575 |
0.262 |
−3.450 |
3.545 |
80% ethanol |
0.172 |
0.175 |
−0.465 |
0.260 |
−3.212 |
3.323 |
70% ethanol |
0.063 |
0.085 |
−0.368 |
0.311 |
−2.936 |
3.102 |
60% ethanol |
−0.040 |
0.138 |
−0.335 |
0.293 |
−2.675 |
2.812 |
50% ethanol |
−0.142 |
0.124 |
−0.252 |
0.251 |
−2.275 |
2.415 |
40% ethanol |
−0.221 |
0.131 |
−0.159 |
0.171 |
−1.809 |
1.918 |
30% ethanol |
−0.269 |
0.107 |
−0.098 |
0.133 |
−1.316 |
1.414 |
20% ethanol |
−0.252 |
0.042 |
−0.040 |
0.096 |
−0.823 |
0.916 |
10% ethanol |
−0.173 |
−0.023 |
−0.001 |
0.065 |
−0.372 |
0.454 |
Methanol |
0.276 |
0.334 |
−0.714 |
0.243 |
−3.320 |
3.549 |
95% methanol |
0.270 |
0.278 |
−0.520 |
0.230 |
−3.368 |
3.365 |
90% methanol |
0.258 |
0.250 |
−0.452 |
0.229 |
−3.206 |
3.175 |
80% methanol |
0.172 |
0.197 |
−0.319 |
0.241 |
−2.912 |
2.842 |
70% methanol |
0.098 |
0.192 |
−0.260 |
0.266 |
−2.558 |
2.474 |
60% methanol |
0.053 |
0.207 |
−0.238 |
0.272 |
−2.157 |
2.073 |
50% methanol |
0.023 |
0.223 |
−0.222 |
0.264 |
−1.747 |
1.662 |
40% methanol |
0.020 |
0.222 |
−0.205 |
0.218 |
−1.329 |
1.259 |
30% methanol |
0.016 |
0.187 |
−0.172 |
0.165 |
−0.953 |
0.898 |
20% methanol |
0.022 |
0.142 |
−0.138 |
0.088 |
−0.574 |
0.559 |
10% methanol |
0.012 |
0.072 |
−0.081 |
0.026 |
−0.249 |
0.266 |
Table 7 Determined descriptors for the tris(acetylacetonates)
Complex |
E
|
S
|
A
|
B
|
V
|
L
|
log Kw |
N
|
SD |
Cr(acac)3 |
2.222 |
1.67 |
0.00 |
1.74 |
2.2830 |
11.77 |
10.89 |
30 |
0.123 |
Fe(acac)3 |
2.524 |
2.25 |
0.00 |
1.74 |
2.2800 |
12.34 |
12.38 |
46 |
0.136 |
Co(acac)3 |
2.694 |
2.21 |
0.00 |
1.91 |
2.2800 |
12.69 |
13.36 |
68 |
0.205 |
V(acac)3 |
2.480 |
2.55 |
0.00 |
1.57 |
2.2840 |
12.82 |
12.57 |
6 |
0.013 |
Y(acac)3 |
2.480 |
2.85 |
0.00 |
1.94 |
2.2834 |
13.26 |
15.13 |
10 |
0.182 |
Sm(acac)3 |
2.480 |
2.83 |
0.00 |
2.36 |
2.2835 |
13.27 |
17.07 |
12 |
0.196 |
La(acac)3 |
2.480 |
3.01 |
0.00 |
2.38 |
2.3233 |
13.53 |
17.61 |
10 |
0.190 |
Nd(acac)3 |
2.480 |
2.98 |
0.00 |
2.37 |
2.3088 |
13.49 |
17.49 |
12 |
0.158 |
The 14 calculated and observed values of log
P for Cr(acac)3 in Table 4 yield an average error AE of 0.042, an average absolute error AAE of 0.094 and a standard deviation of 0.125
log units. For the total of 30 simultaneous equations the SD is 0.123
log units. This is shown in Table 7, where N is the total number of equations used. We left out data in three solvents in Table 4, where the difference between log
P(calc) and log
P(obs) was very large.
For Fe(acac)3, water–solvent partition coefficients are known into propan-2-ol, DMSO, and various water–methanol mixtures.26 Solubilities have been determined in several solvents30,34 but apparently not in water itself. However, we can take log
Cw as another descriptor to be determined through our analysis. We find that with log
Cw = −2.38, the observed values of log
P and those calculated from log
Cs through eqn (7) are very consistent. We calculate V = 2.2800 and from the refractive index that we have determined, Table 2, we calculate E = 2.524; then the unknowns to be found by solution of the set of simultaneous equations in log
P are S, A, B, L, log
Kw and log
Cw. We used a total of 22 values of log
P, 22 values of the corresponding log
Ks through eqn (8) and two equations in log
Kw leading to a set of 46 simultaneous equations. These 46 equations yielded the descriptors shown in Table 7 and log
Cw = −2.37, with a standard deviation between calculated and observed dependent variables of 0.136
log units. The calculated and observed values of log
P are in Table 8. For the 22 used values, AE = 0.018, AAE = 0.117 and SD = 0.140
log units.
Table 8 Calculated and observed values of water–solvent partition coefficients, as log
P, for Fe(acac)3
Solvent |
log P(calc) |
log P(obs) |
Ref. |
|
Hexane |
−0.519 |
−0.367 |
34
|
|
Heptane |
−0.425 |
−0.430 |
34
|
|
Cyclohexane |
0.221 |
−0.005 |
34
|
|
Dichloromethane |
2.898 |
2.313 |
30
|
Not used |
Tetrachloromethane |
1.447 |
1.900 |
34
|
Not used |
Benzene |
2.182 |
2.263 |
34
|
|
Toluene |
1.817 |
1.923 |
34
|
|
Chlorobenzene |
2.196 |
2.309 |
34
|
|
Tetrahydrofuran |
1.818 |
1.858 |
30
|
|
Dimethylformamide |
2.083 |
2.060 |
30
|
|
Dimethylsulfoxide |
2.175 |
1.890 |
26
|
|
Propan-1-ol |
1.379 |
0.930 |
26
|
Not used |
Propan-2-ol |
1.143 |
0.928 |
26
|
|
tert-Butanol |
0.772 |
0.648 |
26
|
|
Methanol |
1.827 |
1.840 |
26
|
|
95% methanol |
1.614 |
1.761 |
26
|
|
90% methanol |
1.633 |
1.689 |
26
|
|
80% methanol |
1.364 |
1.489 |
26
|
|
70% methanol |
1.187 |
1.350 |
26
|
|
60% methanol |
1.013 |
1.104 |
26
|
|
50% methanol |
0.836 |
0.791 |
26
|
|
40% methanol |
0.677 |
0.508 |
26
|
|
30% methanol |
0.490 |
0.333 |
26
|
|
20% methanol |
0.346 |
0.193 |
26
|
|
10% methanol |
0.185 |
0.096 |
26
|
|
In the case of Co(acac)3, solubilities are known in water (log
Cw = −2.41),29 dimethylformamide,29 dodecane,27 various ethanol–water mixtures28 and alkoxyethanols.35 Alousy and Burgess.26 have listed partition coefficients into methanol–water mixtures and into a large number of pure solvents. The solubilities were converted into log
P values, and we were able to use 33 such values. We also had 33 of the corresponding log
Ks values, and two equations in log
Kw giving a total of 68 simultaneous equations. These were solved to yield the descriptors in Table 7 with an SD between the 68 calculated and observed values of 0.205
log units. The observed and calculated values of log
P are in Table 9. For the 33 calculated and observed values that we used, AE = 0.006, AAE = 0.136 and SD = 0.158
log units.
Table 9 Calculated and observed values of water–solvent partition coefficients, as log
P, for Co(acac)3
Solvent |
log P(calc) |
log P(obs) |
Ref. |
|
Decane |
−1.004 |
−0.84 |
26
|
|
Dodecane |
−1.115 |
−1.090 |
27
|
|
Tetrachloromethane |
0.801 |
1.556 |
26
|
Not used |
Dimethylformamide |
1.233 |
1.282 |
29
|
|
Dimethylsulfoxide |
1.427 |
1.156 |
26
|
|
Propan-1-ol |
0.849 |
0.280 |
26
|
Not used |
Propan-2-ol |
0.593 |
0.280 |
26
|
Not used |
Butan-1-ol |
0.672 |
0.928 |
26
|
|
Hexan-1-ol |
0.689 |
0.948 |
26
|
|
Octan-1-ol |
0.504 |
0.543 |
26
|
|
Decan-1-ol |
0.510 |
0.367 |
26
|
|
2-Ethoxyethanol |
1.106 |
0.910 |
35
|
|
2-Butoxyethanol |
0.924 |
0.690 |
35
|
|
Methanol |
1.348 |
1.346 |
26
|
|
95% methanol |
1.109 |
1.240 |
26
|
|
90% methanol |
1.048 |
1.210 |
26
|
|
80% methanol |
0.916 |
1.190 |
26
|
|
70% methanol |
0.796 |
1.000 |
26
|
|
60% methanol |
0.691 |
0.808 |
26
|
|
50% methanol |
0.586 |
0.664 |
26
|
|
40% methanol |
0.497 |
0.420 |
26
|
|
30% methanol |
0.367 |
0.221 |
26
|
|
20% methanol |
0.278 |
0.140 |
26
|
|
10% methanol |
0.158 |
0.065 |
26
|
|
Ethanol |
1.129 |
1.051 |
28
|
|
96% ethanol |
1.091 |
0.932 |
28
|
|
95% ethanol |
1.084 |
0.999 |
28
|
|
90% ethanol |
1.039 |
1.195 |
28
|
|
80% ethanol |
1.057 |
1.201 |
28
|
|
70% ethanol |
0.944 |
1.027 |
28
|
|
60% ethanol |
0.894 |
0.832 |
28
|
|
50% ethanol |
0.796 |
0.642 |
28
|
|
40% ethanol |
0.698 |
0.472 |
28
|
|
30% ethanol |
0.513 |
0.325 |
28
|
|
20% ethanol |
0.289 |
0.201 |
28
|
|
10% ethanol |
0.087 |
0.093 |
28
|
|
The determined descriptors for the complexes of Cr, Fe and Co, Table 7, do not very greatly between the three. All the complexes are quite polarizable, with S ranging from 1.67 to 2.25, as evidenced also by their dipole moments that vary from 0.95 to 1.10.36 There is little variation in hydrogen bond basicity, and all the complexes have A as zero. It might have been expected that a concentrated positive charge in the middle of the molecule would induce some hydrogen bond acidity for the –CH
hydrogen atom, but there is no doubt that A = 0.
There are limited data for other M(acac)3 complexes, but only in a few cases are there enough to attempt to deduce descriptors. Even then, without a knowledge of E and V, little can be done. Values of V can be calculated exactly as for Fe(acac)3 with the atomic increments for M3+ atoms as shown in Table 2,21 but without any experimental values of molar refraction, E cannot be determined. However, E does not seem to alter very much with the nature of the M3+ atom, and in the case of the Cr, Fe and Co complexes, values of the obtained descriptors vary only slightly with change in the taken value of E. We therefore took E as 2.48, the average of the Cr, Fe and Co complexes,
Imura and N. Suzuki37 have determined log
P values for V(acac)3, and there is just enough information to obtain the descriptors given in Table 7. There is a little more data for the M(acac)3 complexes of yttrium, samarium, lanthanum and neodymium38 and these yield the descriptors in Table 7. The descriptors S, B, L and log
Kw for these extra five complexes fall into the same pattern as shown by the Cr, Fe and Co complexes. All four descriptors alter regularly with increase in the complex molecular weight, MW, over the eight complexes. The B-descriptor varies the most regularly, see eqn (11). This equation might be useful in the assignment of descriptors to other M(acac)3 complexes.
| B = −0.904 + 0.00750MW N = 8, SD = 0.087, R2 = 0.937, F = 89.72 | (11) |
By comparison to organic compounds, there have been comparatively few studies on physicochemical properties of inorganic complexes. Analysis of solubilities has provided estimates of the solubility parameter of complexes,27,34,39 but the various predictive methods that have been so useful for organic compounds1–5 have not been applied to inorganic complexes. Now that we have descriptors for the three complexes, Table 7, these can be used to predict log
P values from the gas phase and from water to a very large number of both dry and wet solvents, as well as to several ionic liquids. We can illustrate this by the calculation of the water to wet octanol partition coefficient, as log
Poct, commonly used as a measure of hydrophobicity. It is a simple matter to combine the descriptors in Table 7 with the equation coefficients given in Table 5 to yield the values given in Table 10. Our calculated log
Poct values vary regularly with complex molecular weight, from the somewhat hydrophobic Cr(acac)3 to the decidedly hydrophilic Sm, La and Nd complexes, as shown in eqn (12) and by Fig. 3.
| Log Poct = 13.989 − 0.0345MW N = 8, SD = 0.230, R2 = 0.979, F = 247.2 | (12) |
Table 10 Values of log
Poct for acetylacetonate complexes and some organic compounds
Compound |
log Poct |
Cr(acac)3 |
2.26 |
Fe(acac)3 |
1.81 |
Co(acac)3 |
1.36 |
V(acac)3 |
2.07 |
Y(acac)3 |
0.47 |
Sm(acac)3 |
−0.96 |
La(acac)3 |
−1.06 |
Nd(acac)3 |
−1.05 |
|
|
Glycerol tributanoate |
3.60 |
Dibutyl adipate |
3.88 |
Diheptyl ether |
6.42 |
Pentadecane |
8.56 |
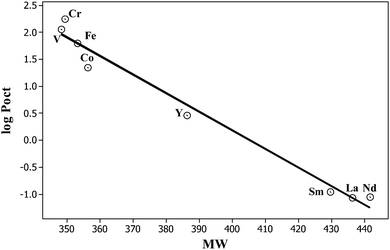 |
| Fig. 3 A plot of calculated values of the water–octanol partition coefficient, as log Poct, for the eight M(acac)3 complexes against the complex molecular weight. | |
The quite good statistics of eqn (12) suggest that our assignment of E = 2.48 for five of the complexes is at least reasonable.
We can compare the values of log
Poct for the complexes with those for organic compounds with around 15 carbon atoms. This indicates that all the M(acac)3 complexes are much less hydrophobic than even glycerol tributanoate, which also has six oxygen atoms. Indeed, several of the complexes are hydrophilic.
Conclusions
We have shown that it is possible to employ the same methodology used to obtain Abraham descriptors for organic compounds to obtain Abraham descriptors for inorganic complexes. Then the various equations we have constructed for organic compounds can be used to predict a very large range of physicochemical properties for inorganic complexes. We have already shown40 that the methods used here can be extended to obtain descriptors for electrolytes and there is no fundamental reason why our extended method cannot be used to obtain descriptors for charged inorganic complexes (electrolytes) as well as for inorganic complexes that are nonelectrolytes, The main difficulty in obtaining descriptors for the inorganic complexes is in the estimation of the E-descriptor. In the present work we have determined the E-descriptor from our experimentally determined molar refractions for the tris(acetylacetonato)chromium(III), tris(acetylacetonato)iron(III) and tris(acetylacetonato)cobalt(III) complexes. This is a time-consuming procedure and at the moment is a limiting factor on the determination of the Abraham descriptors.
Conflicts of interest
There are no conflicts to declare.
References
- BioLoom, BioByte Corp, 201 W. 4th Street, #204 Claremont, CA 91711-4707, USA.
- EPI Suite TM (2000–2012).
- ChemSketch. ACD Advanced Chemistry Development, 110 Yonge Street, 14th Floor, Toronto, Ontario, M5C 1T4, Canada.
- Absolv data base (2011), ADME Suite 5.0, Advanced Chemistry Development, 110 Yonge Street, 14th Floor, Toronto, Ontario, M5C 1T4, Canada.
- SPARC http://archemcalc.com/sparc.
- M. H. Abraham, Chem. Soc. Rev., 1993, 22, 73–83 RSC.
- M. H. Abraham, A. Ibrahim and A. M. Zissimos, J. Chromatogr. A, 2004, 1037, 29–47 CrossRef CAS PubMed.
- C. F. Poole, S. N. Atapattu, S. K. Poole and A. K. Bell, Anal. Chim. Acta, 2009, 652, 32–53 CrossRef CAS PubMed.
- C. F. Poole, T. C. Ariyasena and N. Lenca, J. Chromatogr. A, 2013, 1317, 85–104 CrossRef CAS PubMed.
-
E. D. Clarke and L. Mallon, The Determination of Abraham Descriptors and their Application to Crop Protection Research, in Modern Methods in Crop Protection Research, ed. P. Jeschke, W. Krämer, U. Schirmer and M. Witschel, Wiley-VCH Verlag GmbH & Co., 2012 Search PubMed.
- M. H. Abraham, R. E. Smith, R. Luchtefeld, A. J. Boorem, R. Luo and W. E. Acree, Jr, J. Pharm. Sci., 2010, 99, 1500–1515 CrossRef CAS PubMed.
- M. Brumfield, W. E. Acree Jr. and M. H. Abraham, Phys. Chem. Liq., 2015, 53, 25–37 CrossRef CAS.
- M. Brumfield, A. Wadawadigi, N. Kuprasertkul, S. Mehta, W. E. Acree Jr. and M. H. Abraham, Phys. Chem. Liq., 2015, 53, 10–24 CrossRef CAS.
- M. H. Abraham, J. Gil-Lostes, W. E. Acree, Jr., J. E. Cometto-Muñiz and W. S. Cain, J. Environ. Monit., 2008, 10, 435–442 RSC.
- M. H. Abraham and W. E. Acree, Jr., New J. Chem., 2012, 36, 626–631 RSC.
- M. H. Abraham and W. E. Acree, Jr., J. Mol. Liq., 2017, 232, 325–331 CrossRef CAS.
- A. Fucaloro, A. Zanella, S. Widjaja and J. Widjaja, J. Solution Chem., 2005, 34, 1357 CrossRef CAS.
- A. F. Fucaloro, J. Solution Chem., 2002, 31, 601–605 CrossRef CAS.
-
S. Endo, N. Watanabe, N. Ulrich, G. Bronner and K.-U. Goss, UFZ-LSER database v 2.1 [Internet], Leipzig, Germany, Helmholtz Centre for Environmental Research-UFZ. 2015, accessed on 04.08.2016, available from https://www.ufz.de/index.php?en=31698&contentonly=1&m=0&lserd_data[mvc]=Public/start.
- M. H. Abraham and J. C. McGowan, Chromatographia, 1987, 23, 243–246 CAS.
- Y. H. Zhao, M. H. Abraham and A. M. Zissimos, J. Chem. Inf. Comput. Sci., 2003, 43, 1848–1854 CrossRef CAS PubMed.
- D. Tran, J. P. Hunt and S. Wherland, Inorg. Chem., 1992, 31, 2460–2464 CrossRef CAS.
- G. J. Kruger and E. C. Reynhardt, Acta Crystallogr., 1974, B30, 822–824 CrossRef.
- B. Morosin, Acta Crystallogr., 1965, 19, 131–137 CrossRef CAS.
- R. B. Roof, Acta Crystallogr., 1956, 9, 781–786 CrossRef CAS.
- A. Alousy and J. Burgess, Polyhedron, 1992, 11, 531–539 CrossRef.
- H. Watari, H. Ochima and N. Suzuki, Quant. Struct.-Act. Relat., 1984, 3, 17–22 CrossRef.
- V. A. Fedorov, N. V. Vologdin, N. P. Samsonova, P. V. Fabinskii and A. V. Fedorova, Russ. J. Inorg. Chem., 2011, 56, 141–144 CrossRef CAS.
- N. V. Vologdin, P. V. Fabinskii and V. A. Fedorov, Russ. J. Phys. Chem., 2015, 89, 65–68 CrossRef CAS.
- S. Brandani, V. Brandani and G. Di Giacomo, Gazz. Chim. Ital., 1994, 124, 295–297 CAS.
- M. H. Abraham and W. E. Acree, Jr., J. Solution Chem., 2016, 45, 861–874 CrossRef CAS.
- I. A. Sedov, M. A. Stolov, E. Hart, D. Grover, H. Zettl, V. Koshevarova, C. Dai, S. Zhang, W. E. Acree, Jr. and M. H. Abraham, J. Mol. Liq., 2015, 209, 196–202 CrossRef CAS.
- I. A. Sedov, M. A. Stolov, E. Hart, D. Grover, H. Zettl, V. Koshevarova, W. E. Acree, Jr. and M. H. Abraham, J. Mol. Liq., 2015, 208, 63–70 CrossRef CAS.
- H. Koshimura, J. Inorg. Nucl. Chem., 1978, 40, 865–874 CrossRef CAS.
- Y. Yoshimura, Can. J. Chem., 1989, 67, 2108–2115 CrossRef CAS.
- V. Singh and R. Sahai, J. Macromol. Sci., Chem., 1985, A22, 33–42 CrossRef CAS.
- H. Imura and N. Suzuki, Bull. Chem. Soc. Jpn., 1986, 59, 2779–2783 CrossRef CAS.
- O. Erämetsä and S. Hämälä, Suom. Kemistil. B, 1958, 31, 204–207 Search PubMed.
- J. W. Mitchell and C. V. Banks, J. Inorg. Nucl. Chem., 1969, 31, 2105–2115 CrossRef CAS.
- M. H. Abraham and W. E. Acree, Jr., J. Org. Chem., 2010, 75, 1006–1015 CrossRef CAS PubMed.
|
This journal is © The Royal Society of Chemistry and the Centre National de la Recherche Scientifique 2017 |