DOI:
10.1039/C7FO00341B
(Paper)
Food Funct., 2017,
8, 1810-1821
In vivo fermentation of a Pleurotus eryngii polysaccharide and its effects on fecal microbiota composition and immune response†
Received
1st March 2017
, Accepted 26th April 2017
First published on 28th April 2017
Abstract
A novel, homogeneous Pleurotus eryngii polysaccharide (PEP) (molecular weight 426 kDa, purity 91.25 ± 3.14%) which mainly consisted of glucose with β-type glycosidic linkages was used to investigate in vivo fermentation behavior and effects on immune response in mice. Different doses (0.2, 0.4, 0.8 g per kg body weight) were orally administered to the mice for a period of six weeks. The results showed that the SCFA concentration, pH value, and moisture contents of cecum and colon contents were significantly altered with high-dose PEP treatment compared to the control group (P < 0.05). Moreover, the fecal microbiota in the PEP treated group was found to be structurally different compared to the control group; especially, the Porphyromonadaceae, Rikenellaceae, Bacteroidaceae and Lactobacillaceae abundances were all increased at the family level. In addition, the exerted immune response was significantly altered after the high-dose PEP oral administration. This exploratory study indicated that intake of PEP could have a positive role in gastrointestinal tract health.
1. Introduction
As the main substance with multiple biological functions, including antioxidant,1 immune-enhancing,2 hypoglycemic,3 renal protection,4 and gastrointestinal regulating activities,5 polysaccharides isolated from natural sources have become one of the most significant active components in numerous life processes. It has been proved in various studies that due to the deficiency of carbohydrate-active enzymes, most polysaccharides, which are also called non-digestible polysaccharides, cannot be digested or absorbed by the body directly.6,7 In recent years, with the deepening of the understanding of the relationships between food components and gastrointestinal function, scientists have found that the gastrointestinal tract could be one of the most important loci for the functional performance of natural polysaccharides.8 So far, considerable experimental research has shown that natural polysaccharides exert activities mainly through fermentation reaction by the colonial microbiota in the gastrointestinal tract.9 The polysaccharides will be decomposed and used by the gut microbiota in the gastrointestinal tract to serve as an energy source for diverse beneficial bacterial groups, including the promotion of their propagation and production of beneficial compounds. These compounds are mostly short-chain fatty acids (SCFAs), including acetate, propionate, butyrate and valerate acid. As the essential nutritional sources for the colonic epithelium, SCFAs not only can modulate gastrointestinal inflammatory conditions, but also can increase the blood flow, maintain colonic homeostasis and affect the composition of the mucus layer.10 Besides their biological functions, existence of more SCFAs has been shown to lower the pH value of the gut luminal environment and this has a deep relationship with the health of gastrointestinal tracts. Above all, SCFAs can particularly reduce the risk of some diseases including the irritable bowel syndrome,11 inflammatory bowel disease (IBD)12 and cardiovascular diseases.13 On the other hand, non-digestible polysaccharides could also increase the gut content moisture to generate softer fecal matter, which is beneficial for the host health.
Edible mushrooms seem to be potential candidates for non-digestible polysaccharides as they contain different functional carbohydrates which are all resistant to acid hydrolysis in the stomach and remain unbroken by human digestive enzymes. Pleurotus eryngii, as a new kind of important species of edible mushrooms, displays multiple biological functions due to its unique variety of bioactive compounds. P. eryngii polysaccharide has been shown to exhibit remarkable activities and has attracted the attention of many researchers.14,15 In recent years, the antitumor, antioxidant, hepatoprotective, hypolipidemic, anti-obesity and the immuno-stimulating activities and mechanisms of P. eryngii polysaccharide have been illustrated in cell and animal models.15,16 However, the fermentation behavior of P. eryngii polysaccharide and its effects on fecal microbiota composition and immune response have not been studied.
In the present study, we extracted and purified a P. eryngii polysaccharide fraction named PEP and elucidated its structural characterization. The fermentation behaviors, including the regulation of SCFA concentration, pH value and moisture content of colon and cecum luminal contents, were evaluated using animal experiments. The fecal microbiota composition was also evaluated using a MiSeq PE250 Illumina next generation sequencing platform after sequencing the 16S DNA V4 fragment of fecal DNA. In addition, the effects of PEP gavage on immune response were studied. Consequently, the beneficial effects of PEP administration on the gastrointestinal tract's health were evaluated through all the experimental results.
2. Materials and methods
2.1 Materials and chemicals
Fresh P. eryngii was purchased from a local market (Nanjing, China). All the six high purity SCFA standards (acetic, propionic, i-butyric, n-butyric, i-valeric and n-valeric acid) were purchased from Sigma (St Louis, MO, USA) and used for the preparation of standard solutions. T-series dextran standards (T-500, T-200, T-100, T-50, and T-10) were purchased from Amersham Pharmacia (Uppsala, Sweden). The monosaccharide standards 1-phenyl-3-methyl-5-pyrazolone (PMP) and acetonitrile (HPLC grade) were purchased from Sigma Chemical Co. (St Louis, MO, USA). All other reagents were of analytical grade.
2.2 Animals
C57BL/6 male mice (6 weeks old), weighing 20.00 ± 2.0 g, were purchased from the Comparative Medicine Center of Yangzhou University (Jiangsu, China), certificate no. SCXK (Su) 2012-0004, and housed in the laboratory animal center of Nanjing Agricultural University (Jiangsu, China). The animals were acclimatized to an ambient temperature of 25 ± 2 °C, relative humidity (50 ± 5%), and a 12/12 h light–dark cycle for 2 weeks and received a standard chow diet throughout the experiment. All effort was made to minimize the number of animals used and to minimize their suffering.
2.3 Preparation and characterization of PEP
The preparation of PEP was carried out according to our previous studies.17,18 Characterization analyses of PEP, including purity detection, homogeneity and molecular weight determination, monosaccharide composition analysis, determination of sulfate and uronic acid contents and ultraviolet (UV) and Fourier-transform infrared spectroscopy (FT-IR) assays, were carried out as described in the ESI.†
2.4 Animal experiment
2.4.1 Experiment design.
Each mouse was individually housed in a cage and all mice were randomly divided into four groups of 10 mice each: (1) PEP low-dose group (LP), mice were orally administered a PEP dose of 0.2 g per kg body weight; (2) PEP middle-dose group (MP), mice were orally administered a PEP dose of 0.4 g per kg body weight; (3) PEP high-dose group (HP), mice were orally administered a PEP dose of 0.8 g per kg body weight; (4) control group (Blank), mice were given distilled water of the same volume as the average value volume of the PEP treated group. All mice were given oral administration of PEP or distilled water (control) by gavage at about 9:00 a.m. during the experimental period. The oral administration volume of each mouse was adjusted according to the daily weight, which was weighed before administration every day. The physical health condition was also observed based on the body weight. After 6 weeks of the designated experimental period, the mouse feces were collected individually and used for stool DNA extraction. Blood was drawn from the eyes of each mouse, and the serum was isolated by centrifugation at 1000g at 4 °C for 15 min. Then, all mice were sacrificed by cervical dislocation under diethyl ether anesthesia before oral administration and the colons and ceca were aseptically removed immediately, longitudinally opened on an ice-cold plate to obtain the luminal contents. Contents from each segment were divided into 3 equal portions and kept at −20 °C for the determination of the SCFA concentration, moisture content, and pH value.
2.4.2 SCFA concentration determination.
The determination of the SCFA concentration of the luminal contents of each mouse was carried out by chromatographic analysis (GC) based on a previous description19 with some modifications. Specifically, the standard curves for acetic acid were made in the range of 0.025–0.8 mol L−1, and the other standard curves of propionic, i-butyric, n-butyric, i-valeric and n-valeric acid were made in the range of 0.0125–0.4 mol L−1 and by adding 4-methylvaleric acid as the internal standard.
2.4.3 pH value measurements.
The pH value was determined under the following description. Briefly, the contents were dissolved in distilled water in a ratio of 1
:
10 (w/v), after which the solution was vortexed for 3 min to make it homogeneous, and a micro-pH meter was used for the measurements of the pH value.
2.4.4 Moisture content detection.
The last portion of the luminal contents was used for the determination of moisture content. Samples were weighed (recorded as WB) and dried to a constant weight (recorded as WA) at 110 °C. The moisture content was calculated as:
Moisture content = (WB − WA)/WB × 100% |
2.4.5 Fecal DNA extraction and high throughput sequencing.
The genomic DNA of mice feces was extracted using a QIAamp DNA stool kit (QIAGEN, Inc., Shanghai, China) according to the manufacturer's instructions. DNA quality was checked on a 1.0% agarose gel and its concentration was measured using a UV–vis spectrophotometer (NanoDrop 2000, Waltham, MA, USA). All the DNA samples were stored at −20 °C.
Illumina libraries were prepared using a NEXTflex 16S V4 Amplicon-Seq Kit. Briefly, from 50 ng of DNA template for each sample, the bacterial V4 region of the 16S ribosomal gene was amplified using the universal primers V3F (GTGCCAGCMGCCGCGGTAA) and V4R (GGACTACHVGGGTWTCTAAT) tailed with Illumina barcoded adapters under the following touchdown PCR conditions: A 20 μL mixture was prepared for each reaction and included a 1× reaction buffer (TAKARA), 2 mM Mg2+, 0.2 mM dNTP, 0.1 μM of each primer, 1 U HotStarTaq polymerase (TAKARA) and 2 μL template DNA. The cycling programme was: 95 °C for 2 min; 35 cycles of 94 °C for 20 s, 55 °C for 40 s, 72 °C for 1 min, 72 °C for 2 min. PCR products were purified using Agencourt XP AmpureBeads. The quality of the final products was assessed using a Bioanalyzer 2100. After their quantification with Qubit (Invitrogen, Inc., USA), the samples were pooled in equal proportions and sequenced paired-end in an Illumina MiSeq with 312 cycles (150 cycles for each paired read and 12 cycles for the barcode sequence) at IGA Technology Services (Inc., Italy). To prevent focusing and phasing problems due to sequencing of “low diversity” libraries such as 16S amplicons, 30% PhiX genome was spiked in the pooled library.
2.4.6 Bioinformatics and statistical analysis.
The high-quality sequences were assigned to samples according to barcodes. The alpha-diversity analyses, including indexes of Chao, Simpson, Shannon, and Coverage, were performed using the Mothur software. The valid sequences were clustered into operational taxonomic units (OTUs) at 97% sequence identity using UPARSE embedded in Quantitative Insights in the Microbial Ecology software package (QIIME, version 1.8.0, http://bio.cug.edu.cn/qiime/). Community structure comparisons with Jaccard tree clustering analysis, UniFrac analysis and heatmap were performed using the Mothur and R software packages (http://www.R-project.org).
2.4.7 Viscera index and immune cytokine detection.
The viscera indices, which could reflect the body immune function alteration, were calculated using the formula: Liver, Spleen or Thymus index = Liver or Spleen or Thymus weight (g)/body weight (g). The concentrations of immune factors TNF-α, IFN-γ, IL-1, IL-2, IL-6, and IL-12 in the serum and SlgA in the cecum and colon contents were detected using a commercial Enzyme-Linked Immunosorbent Assay (ELISA) kit (Jiancheng BioEngineering, Nanjing, China) according to the manufacturer's instructions.
2.4.8 Ethics statement.
Animals were maintained in accordance with the guidelines of the National Institutes of Health for the care and use of laboratory animals (NIH Publication No. 8023, revised 1978), U.K., and all treatments were approved by the Ethical Committee of Experimental Animal Center of Nanjing Agricultural University with permission no. SYXK (SU) 2011-0036.
2.5 Statistical analysis
Statistical analysis was performed with one-way analysis of variance (ANOVA) using the SPSS 18.0 software (Statistical Package for Social Sciences, SPSS Inc., Chicago). The differences of SCFA concentration, pH value, moisture content, and immune factor concentrations between PEP treated groups and the control group were estimated as the mean ± standard deviation and compared using a Tukey's test at the 5% confidence level.
3. Results
3.1 Primary structure of PEP
The purity, molecular weight, monosaccharide composition and sulfate contents of PEP are shown in Table 1. From the absence of absorbance peaks at 260 nm and 280 nm, the UV spectra analysis revealed that no nucleic acid or protein existed in PEP (Fig. 1A). Moreover, Fig. 1B shows the FT-IR spectra of PEP ranging from 500 to 4000 cm−1; the broad stretching peak around 3400 cm−1 (3399.9 cm−1) represented the hydroxyl groups (–OH), which reveals the strong inter- and intra-molecular interactions of the polysaccharide chains of PEP. The weak band at 2942.8 cm−1 is attributed to C–H stretching vibrations. The peaks around 1640 cm−1 and 1580 cm−1 correspond to the vibrations of C
O from carboxylates. The absorption bands with weak intensities around 1420 and 1374 cm−1 of PEP could be due to the deforming vibrations of the C–H bond.20 Three distinct absorption peaks within the range of 1100–1010 cm−1 indicated the possible presence of the furanose ring in PEP. In particular, small characteristic absorptions at about 941.1 and 862.0 cm−1 suggested that the sugar units of PEP were mainly β-type glycosidic linkages.17
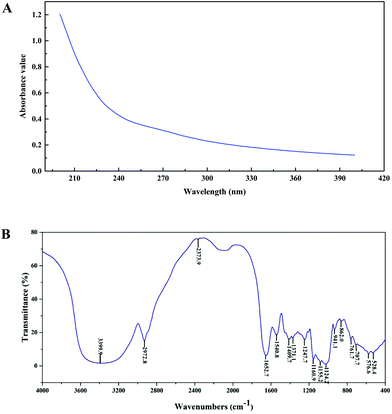 |
| Fig. 1 Ultraviolet (UV) and Fourier-transform infrared (FT-IR) spectra of PEP in the range of 200–400 nm (A) and 500–4000 cm−1 (B). | |
Table 1 Preliminary characteristics (purity, molecular weight, uronic acid, sulfate, and monosaccharide composition) of PEP
|
Purity (%) |
Molecular weight (kDa) |
Sulfate (%) |
Monosaccharide compositiona (%) |
Data are expressed as mol% for each sugar.
|
PEP |
91.25 ± 3.14 |
426 |
0.72 |
Uronic acid |
Glc |
Gal |
Man |
Xyl |
1.36 |
79.11 |
10.37 |
5.75 |
1.77 |
3.2 SCFA determination
The gas chromatograms of the standard and sample solutions are given in ESI Fig. 1.† The total SCFA concentration from mice with different doses of PEP oral administration compared with the control group is presented in Fig. 2. It was found that groups treated with 0.4 g per kg and 0.8 g per kg body weight PEP exhibited significantly higher concentrations of total SCFAs compared to the control group (P < 0.05) in the cecum. However, the total SCFAs of colon contents exhibited significantly lower concentration than those of cecum contents (P < 0.05). With the increase in the PEP dose, the total SCFA concentrations increased from 13.41 ± 1.78 mmol L−1 to 22.08 ± 1.74 mmol L−1 and 5.91 ± 1.23 mmol L−1 to 7.40 ± 1.65 mmol L−1, for cecum and colon contents, respectively.
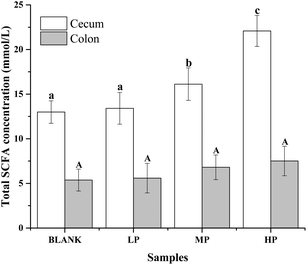 |
| Fig. 2 Total SCFA concentrations (mmol L−1) of the luminal contents in the mice colon and cecum of PEP treated groups with different doses compared to the control group. Values are expressed as the mean ± standard deviation of ten mice. Different lowercase letters mean significant difference (P < 0.05) of the contents in the cecum, and capital letters mean significant difference (P < 0.05) of the contents in the colon. | |
It can be seen from Fig. 3A that the acetic acid concentration in the cecum contents of the high-dose PEP dose group (12.52 ± 1.95 mmol L−1) was significantly (P < 0.05) increased compared to the control group (5.67 ± 1.30 mmol L−1). However, there was no remarkable change in the colon contents for acetic acid levels between the control and the PEP-treated groups. As shown in Fig. 3B, the concentration of propionic acid in cecum contents increased to 1.77 ± 0.16 mmol L−1 in the HP group, which was significantly different (P < 0.05) from the control group. No significant increases were observed in the colon contents for propionic acid values. As shown in Fig. 3(C and D), two configurations of butyrate, i-butyrate and n-butyrate, existed and increased in a PEP dose dependent manner in cecum contents of mice, with the concentrations reaching peaks of 1.12 ± 0.20 mmol L−1 and 4.73 ± 0.47 mmol L−1, respectively, which were remarkably higher compared to the control group (P < 0.05). No i-butyrate was detected and no significant concentration change of n-butyrate was found in colon contents throughout the whole experimental period. Fig. 3(E and F) revealed the different amounts of i-valeric and n-valeric in the cecum and colon contents for the different treatments. No differences were observed between the cecum contents of the control and the PEP administered groups for the n-valeric concentration (P > 0.05). However, the concentrations of i-valeric in the PEP high dose group increased significantly not only in the cecum contents (2.02 ± 0.19 mmol L−1), but also in the colon contents (0.71 ± 0.16 mmol L−1) (P < 0.05), while i-valeric in the cecum and colon contents of the control group were only 1.24 ± 0.09 mmol L−1 and 0.19 ± 0.04 mmol L−1, respectively.
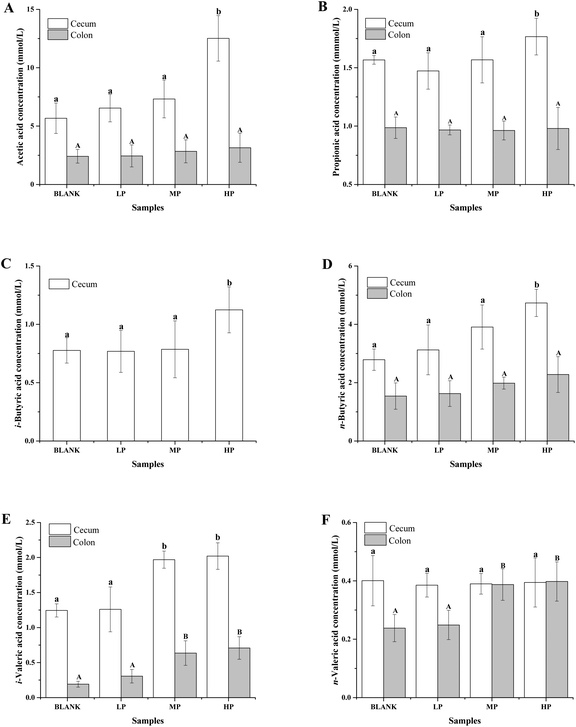 |
| Fig. 3 The concentrations (mmol L−1) of acetic (A), propionic (B), i-butyric (C), n-butyric (D), i-valeric (E), and n-valeric acids (F) of the luminal contents in the mice colon and cecum of PEP treated groups with different doses compared to the control group. Values are expressed as the mean ± standard deviation of ten mice. Different lowercase letters mean significant difference (P < 0.05) of the contents in the cecum, and capital letters mean significant difference (P < 0.05) of the contents in the colon. | |
3.3 pH value and moisture contents of luminal contents in the colon and cecum
As shown in Fig. 4A, the pH value of cecum and colon contents in mice of the control group were 7.65 ± 0.14 and 7.67 ± 0.20, respectively. After the oral administration of PEP for 6 weeks, the pH values of these two contents decreased in a dose-dependent manner, which may have been due to the PEP fermentation products from the microbiota in the gastrointestinal tracts. In particular, the cecum and colon contents of the mice with the high-dose PEP administration exhibited a significant pH reduction, 7.25 ± 0.24 in the cecum contents and 7.29 ± 0.24 in the colon contents, compared to the control group (P < 0.05).
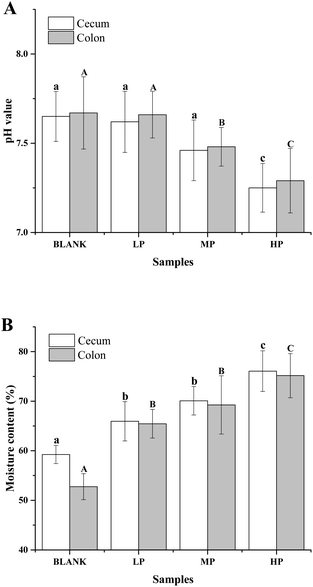 |
| Fig. 4 Regulation of the pH value (A) and moisture content (B) of the luminal contents in the mice colon and cecum by PEP treatment with different doses compared to the control group. Values are expressed as the mean ± standard deviation of ten mice. Different lowercase letters mean significant difference (P < 0.05) of the contents in the cecum, and capital letters mean significant difference (P < 0.05) of the contents in colon. | |
As shown in Fig. 4B, the moisture of luminal contents in the colon and cecum without PEP administration were 59.25 ± 0.02% and 52.76 ± 0.10%, respectively. With the dose of PEP intake increasing, higher moisture content was observed and increased in a dose-dependent manner. It was observed that the low-dose PEP group displayed a significant improvement in both the moisture of cecum and colon contents (P < 0.05) and this could be attributed to the strong water-holding capacity of PEP for the gastrointestinal tract contents throughout the whole experimental period.
3.4 Fecal microbiota composition analysis
The demonstration of the increased abundance of the fecal microbiota with the intake of PEP was analyzed and shown in ESI Table 1.†Fig. 5A shows the microbiota taxonomic composition at the phylum level, Firmicutes, Bacteroidetes, and Proteobacteria were the main dominant phyla, the relative abundance of Firmicutes significantly decreased, whereas the relative abundance of Bacteroidetes significantly increased in the high-dose PEP group compared to the control group (P < 0.05). The Proteobacteria relative abundance of PEP treated and control groups showed no significant difference. Fig. 5B shows the fecal microbiota diversity at the class level, from which the relative abundance of Bacterioidia and Bacilli can be revealed to have significantly increased (P < 0.05) in comparison to the control group. However, the Clostridia relative abundance displayed a decreasing trend with the increase in gavage PEP dose. At the order level (Fig. 5C), Bacteroidales, Clostridiales and Lactobacillales were the primary flora in mice fecal matter, and the ratio of Bacteroidales and Lactobacillales significantly increased, whereas the Clostridiales ratio significantly decreased with the intake of high-dose PEP (P < 0.05). The microbiota composition differences at the family level between the PEP administered administration group and the control group are shown in Fig. 5D. The relative abundance of Porphyromonadaceae, Rikenellaceae, Bacteroidaceae and Lactobacillaceae increased significantly in the high-dose PEP gavage group compared to the control group, whereas the relative abundance of Lachnospiraceae and Ruminococcaceae were changed in the reverse significantly.
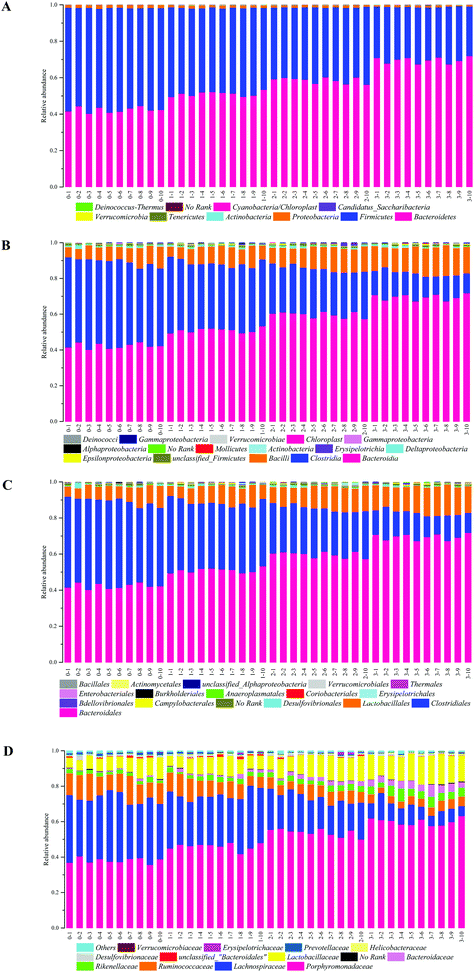 |
| Fig. 5 Bacterial taxonomic profiling in the phylum (A), class (B), order (C) and family (D) levels of fecal microbiota from different treatment groups. Bars marked with 0–1 to 0–10, 1–1 to 1–10, 2–1 to 2–10 and 3–1 to 3–10 show the Blank group, LP group, MP group and HP group individual variations, respectively. | |
The heatmap of mice fecal microbiota is presented in Fig. 6. The fecal microbiota structures of mice with different treatments were presented directly, and the cluster analysis for bacterial communities showed that bacterial communities in the low-dose PEP gavage group clustered close to the control group, while the bacterial communities between middle-dose and high-dose administration groups displayed a close relationship. The differences and similarities between microbiota communities for all groups were clustered by principal coordinate analysis (PCoA) and utilized the unweighted UniFrac distances, which showed significant variation between individuals (Fig. 7).
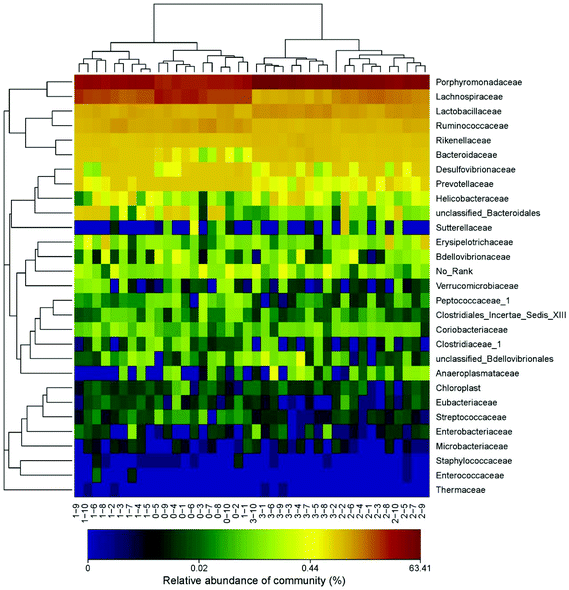 |
| Fig. 6 Heatmap analysis at the family level of fecal microbiota with different treatment. Bars marked with 0–1 to 0–10, 1–1 to 1–10, 2–1 to 2–10 and 3–1 to 3–10 show the Blank group, LP group, MP group and HP group individual variations, respectively. | |
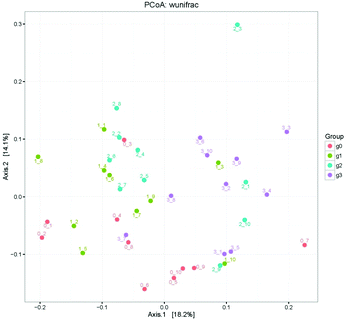 |
| Fig. 7 Principal component analysis for different bacterial communities using unweighted UniFrac distance PCoA. Points marked with 0–1 to 0–10, 1–1 to 1–10, 2–1 to 2–10 and 3–1 to 3–10 show the Blank group, LP group, MP group and HP group individual variations, respectively. | |
3.5 The immune response analysis
ESI Fig. 2† shows the mice body weight change for each week in different groups with no significant differences during the whole gavage process, which indicated the same health situation of each mouse with different treatments. Moreover, the spleen, liver and thymus indices were determined and presented in Table 2. The thymus index of mice treated with high-dose PEP displayed a very significant difference compared to the control group (P < 0.01), whereas the liver and spleen indices showed a significant difference in the high-dose PEP gavage group (P < 0.05). In addition, the secretion of immune cytokines in serum and gut contents of all groups were determined and displayed in Fig. 8. The IL-12 concentrations in serum exhibited no significant change after the PEP administration. However, the concentrations of TNF-α, IFN-γ, IL-1, IL-2 and IL-6 in serum and SlgA in the cecum and colon increased significantly in the high-dose treatment group (P < 0.05), suggesting immune function regulation effects of the PEP administration.
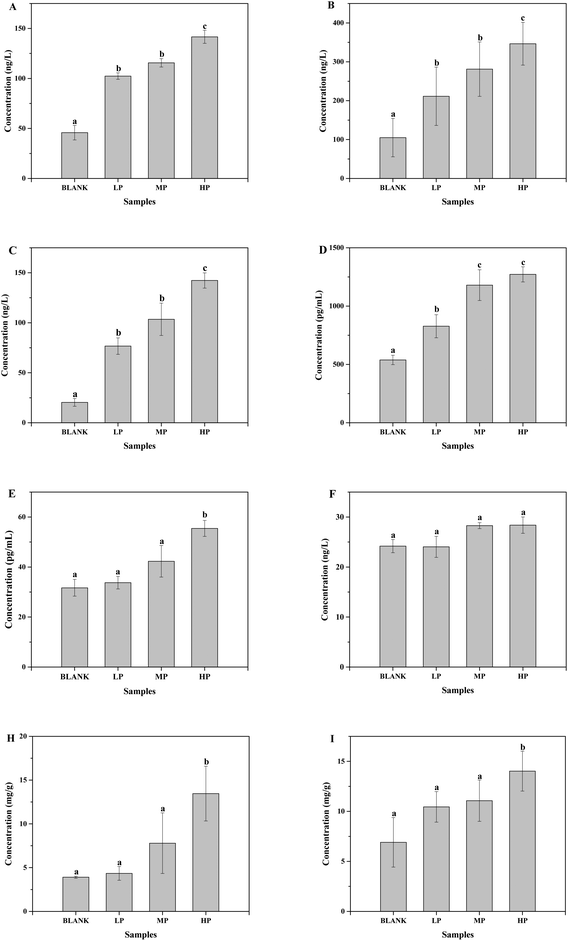 |
| Fig. 8 The effects of PEP on the concentrations of immune cytokines (A, TNF-α; B, IFN-γ; C, IL-1; D, IL-2; E, IL-6; F, IL-12) in serum and SlgA in cecum content (H) and colon content (I). Values are expressed as the mean ± standard deviation of ten mice. Different lowercase letters mean significant difference (P < 0.05). | |
Table 2 The effect of PEP treatments on viscera index
Group |
n
|
Liver index |
Spleen index |
Thymus index |
*P < 0.05, **P < 0.01. |
Blank |
10 |
0.0381 ± 0.004 |
0.0034 ± 0.0002 |
0.0003 ± 0.0001 |
LP |
10 |
0.0392 ± 0.005 |
0.0036 ± 0.0005 |
0.0006 ± 0.0003 |
MP |
10 |
0.0409 ± 0.003* |
0.0037 ± 0.0001 |
0.0007 ± 0.0003* |
HP |
10 |
0.0429 ± 0.003* |
0.0038 ± 0.0006* |
0.0009 ± 0.0003** |
4. Discussion
In the present study, we evaluated the fermentation features of a homogeneous polysaccharide fraction PEP obtained from P. eryngii. The results demonstrated that the intake of PEP could upregulate the total SCFA concentration. As the fermentation products of microbiota utilizing the non-digestible polysaccharides, SCFAs are chemically composed of a carboxylic acid moiety and a small hydrocarbon chain and are found to be important to the gut health and normal metabolic processes.21 Based on our study, PEP was attributed to a high production of SCFAs during the fermentation process in the gastrointestinal tracts, which may play an important role in the development of its bioactivities. The most active locations of the gastrointestinal tract for the fermentation were cecum and proximal colon, which show the highest concentration of SCFAs and the lowest pH value. It is commonly known that the higher the SCFA concentrations in gastrointestinal tracts, the lower the pH value in the gut lumen, which leads to an inhibiting effect on the propagation of some maleficent microorganisms, eventually accelerating the beneficial bacteria function.
In particular, the concentrations of acetic, propionic, i-butyric, n-butyric and i-valeric acids increased significantly in mice cecum contents with the high-dose PEP administration in comparison to the control group. As the most abundant SCFA in the gastrointestinal tracts, it has been shown by some studies that acetic acid can be oxidized by peripheral tissues, brain and heart during metabolism to exert its activities.22 Most bacteria have the ability to utilize the non-digestible carbohydrates as the fermentation substrate to produce acetic acid. On the other hand, acetic acid could be synthesized by some acetogenic bacteria from carbon dioxide and hydrogen or formic acid via the Wood–Ljungdahl pathway.23 As a novel non-digestible polysaccharide, PEP may show its acetic acid producing ability through the above two routes. Some discoveries have revealed that propionic acid was a major metabolite produced from dietary complex carbohydrate fibers in the gut as products of microbiota fermentation and displayed some direct beneficial effects on adipose tissue by reducing obesity-associated inflammation and increasing lipogenesis and glucose uptake.24 According to previous reports, the primary source of propionic acid was the fermentation of glucose, xylose, and arabinose,25 and PEP consists of a relatively higher ratio of glucose, which could explain the results. The propionic acid generation in gastrointestinal tracts mainly depended on acrylate, succinate and propanodiol pathways.26 The increased propionic acid concentration in PEP gavage mice may be explained by the propanodiol pathway where the deoxy-sugars could be converted to propionate by the bacteria, including the Proteobacteria and members of the Lachnospiraceae family.26 The fiber component in the daily food was the main source of butyrate acid in the gastrointestinal tracts. In addition, the low pH value of the gut environment could also have a positive role in the butyrate acid production.
An appropriate lowing of the pH value has been shown to be of assistance to the gastrointestinal tract health by decreasing the risk of bowel cancer.19 The decreasing pH value of the cecum and colon contents, resulting from the intake of PEP, was speculated to be helpful for the intestinal beneficial bacteria proliferation. This may inhibit the proliferation of undesirable pathogens which would therefore be an indicator of the function assessment of the P. eryngii polysaccharide on the gastrointestinal tract health. Moisture contents in the cecum and colon contents were measured to make an evaluation of the effect of PEP on the hardness of the fecal matter. Relevant reports have pointed out that appropriate higher moisture contents in the gut lumen can increase the fecal bulk and impart a beneficial effect on gut health.27
The effects of PEP oral administration on the bacterial communities of mice feces were also analyzed in our study, and the results showed that the intake of PEP exerts a significant impact on the fecal microbiota composition. Bacteroidetes and Firmicutes were the two main phyla in the fecal microbiota and both have a large range of carbohydrate utilizing enzymes, which play the most important role in the non-digestible polysaccharide utilization for both phyla. Previous studies reported that Bacteroidetes were able to produce several SCFAs, such as acetic and propionic acid based on the broad range of glycoside hydrolases and carbohydrate metabolic pathways.28 On the other hand, the ratio of Firmicutes to Bacteroidetes in the PEP gavage group decreased compared to the control group, which also suggested that PEP played a beneficial role in gastrointestinal tract health.29 At the family level, the animal-derived Porphyromonadaceae family members are often β-lactamase producers,30 which may play an important role in the degradation of PEP. In addition, the Porphyromonadaceae abundance has a negative correlation with the fluke infection ratio, and was generally very susceptible to most of the antimicrobial agents commonly used for the treatment of anaerobic infections because of its immune simulating behavior, such as amoxicillin-clavulanate and metronidazole. The Rikenellaceae family, which has proved challenging to cultivate, uses mucin as a carbon and energy source, and appears to outcompete the other members of the digestive-tract microbiome when grown on a medium containing mucin instead of simple carbohydrates.31 PEP oral administration may display a positive role in the production of the mucosa protein, thus giving credence to the increasing abundance of Rikenellaceae. A recent study demonstrated that the increase in Bacteroides, which was classified under Bacteroidaceae, has the function of promoting the secretion of IL-6 in dendritic cells.32 Abundance of Lactobacillaceae was associated with the T cell counts in peripheral blood, and it is well known that Lactobacillus, which is classified under Lactobacillaceae could have effectively enhanced the innate and adaptive immunity of the host. Therefore, the increase of Bacteroidaceae and Lactobacillaceae abundance as a result of PEP administration may partly contribute to the host immune response. Lachnospiraceae and Ruminococcaceae were the most abundant Firmicutes families in gut environments, accounting for roughly 50% and 30% of phylotypes, respectively. Members of these microbial families were better equipped to cleave the cellulose and hemicellulose components of plant material. The decreased abundance of these two families may be due to the monosaccharide composition of PEP. All the microbiota diversity differences between the fecal matter of mice with PEP gavage treatment and the control group need a deeper study in the future, especially the association of the specific characterization of PEP and the metabolic products of the microbiota in the gastrointestinal tracts.
Furthermore, we evaluated the immune response caused by the intake of PEP by determining the immune cytokines in serum and gut contents, and the indices of liver, spleen and thymus. The results showed that PEP could effectively regulate the host immune function. The results presented may have been induced by the fermentation products and the gastrointestinal tract microbiota composition alteration by the intake of PEP, which necessitates a further study of the immune response mechanisms in the future.
5. Conclusion
In the present study, a homogeneous P. eryngii polysaccharide, named PEP with a molecular weight of 426 kDa, was obtained. Analysis revealed that PEP mainly consisted of glucose with β-type glycosidic linkages without nucleic acid and protein presence. The high-dose PEP administration could alter the SCFA concentrations of luminal contents in both the cecum and colon significantly. In addition, pH value and moisture content determination indicated that its intake resulted in a more beneficial lumen environment and a higher water-holding capacity by mice fecal matter. Moreover, the fecal microbiota composition was significantly altered in the high-dose PEP gavage group compared to the control group. Lastly, the immune factor concentration analysis revealed that PEP could remarkably regulate the immune response of the host. Hence, the presented results show that the ingestion of PEP may play an important role in gastrointestinal tract health through its fermentation process by the gut microbiota.
Conflict of interest
There are no conflicts of interest to declare.
Acknowledgements
This work was supported by the China Agriculture Research System (CARS-24) and the Priority Academic Program Development of Jiangsu Higher Education Institutions (PAPD).
References
- J. Jiang, F. Kong, N. Li, D. Zhang, C. Yan and H. Lv, Purification, structural characterization and in vitro antioxidant activity of a novel polysaccharide from Boshuzhi, Carbohydr. Polym., 2016, 147, 365–371 CrossRef CAS PubMed.
- Z. Yu, M. Kong, P. Zhang, Q. Sun and K. Chen, Immune-enhancing activity of extracellular polysaccharides isolated from Rhizopus nigricans, Carbohydr. Polym., 2016, 148, 318–325 CrossRef CAS PubMed.
- C. Chen, L. You, A. M. Abbasi, X. Fu, R. H. Liu and C. Li, Characterization of polysaccharide fractions in mulberry fruit and assessment of their antioxidant and hypoglycemic activities in vitro, Food Funct., 2016, 7, 530–539 CAS.
- Q. Zhao, J. Li, J. Yan, S. Liu, Y. Guo, D. Chen and Q. Luo, Lycium barbarum polysaccharides ameliorates renal injury and inflammatory reaction in alloxan-induced diabetic nephropathy rabbits, Life Sci., 2016, 157, 82–90 CrossRef CAS PubMed.
- T. Zuo, N. Zhang, Q. Zhang, H. Shi, S. Lu, C. Xue and Q. Tang, Transportation of squid ink polysaccharide SIP through intestinal epithelial cells and its utilization in the gastrointestinal tract, J. Funct. Foods, 2016, 22, 408–416 CrossRef CAS.
- M. M. L. Grundy, C. H. Edwards, A. R. Mackie, M. J. Gidley, P. J. Butterworth and P. R. Ellis, Re-evaluation of the mechanisms of dietary fibre and implications for macronutrient bioaccessibility, digestion and postprandial metabolism, Br. J. Nutr., 2016, 116, 816–833 CrossRef CAS PubMed.
- C. Moraes, N. A. Borges and D. Mafra, Resistant starch for modulation of gut microbiota: Promising adjuvant therapy for chronic kidney disease patients?, Eur. J. Nutr., 2016, 1813–1821 CrossRef CAS PubMed.
- S. Lu, T. Zuo, N. Zhang, H. Shi, F. Liu, J. Wu, Y. Wang, C. Xue and Q.-j. Tang, High throughput sequencing analysis reveals amelioration of intestinal dysbiosis by squid ink polysaccharide, J. Funct. Foods, 2016, 20, 506–515 CrossRef CAS.
- Q. Kong, S. Dong, J. Gao and C. Jiang,
In vitro fermentation of sulfated polysaccharides from E. prolifera and L. japonica by human fecal microbiota, Int. J. Biol. Macromol., 2016, 91, 867–871 CrossRef CAS PubMed.
- V. Morampudi, U. Dalwadi, G. Bhinder, H. Sham, S. Gill, J. Chan, K. Bergstrom, T. Huang, C. Ma and K. Jacobson, The goblet cell-derived mediator RELM-β drives spontaneous colitis in Muc2-deficient mice by promoting commensal microbial dysbiosis, Mucosal Immunol., 2016, 9, 1218–1233 CrossRef CAS PubMed.
- M. Camilleri, I. Oduyebo and H. Halawi, Chemical and molecular factors in irritable bowel syndrome: current knowledge, challenges and unanswered questions, Am. J. Physiol.: Gastrointest. Liver Physiol., 2016, 311, G777–G784 CrossRef PubMed.
- T. Takagi, Y. Naito, Y. Higashimura, C. Ushiroda, K. Mizushima, Y. Ohashi, Z. Yasukawa, M. Ozeki, M. Tokunaga and T. Okubo, Partially hydrolysed guar gum ameliorates murine intestinal inflammation in association with modulating luminal microbiota and SCFA, Br. J. Nutr., 2016, 116, 1–7 CrossRef PubMed.
- M. Sanduzzi Zamparelli, D. Compare, P. Coccoli, A. Rocco, O. M. Nardone, G. Marrone, A. Gasbarrini, A. Grieco, G. Nardone and L. Miele, The metabolic role of gut microbiota in the development of nonalcoholic fatty liver disease and cardiovascular disease, Int. J. Mol. Sci., 2016, 17, 1225 CrossRef PubMed.
- S. Li and N. P. Shah, Anti-inflammatory and anti-proliferative activities of natural and sulphonated polysaccharides from Pleurotus eryngii, J. Funct. Foods, 2016, 23, 80–86 CrossRef CAS.
- D. Xu, H. Wang, W. Zheng, Y. Gao, M. Wang, Y. Zhang and Q. Gao, Charaterization and immunomodulatory activities of polysaccharide isolated from Pleurotus eryngii, Int. J. Biol. Macromol., 2016, 92, 30–36 CrossRef CAS PubMed.
- C. Zhang, S. Li, J. Zhang, C. Hu, G. Che, M. Zhou and L. Jia, Antioxidant and hepatoprotective activities of intracellular polysaccharide from Pleurotus eryngii SI-04, Int. J. Biol. Macromol., 2016, 91, 568–577 CrossRef CAS PubMed.
- G. Ma, W. Yang, A. M. Mariga, Y. Fang, N. Ma, F. Pei and Q. Hu, Purification, characterization and antitumor activity of polysaccharides from Pleurotus eryngii residue, Carbohydr. Polym., 2014, 114, 297–305 CrossRef CAS PubMed.
- G. Ma, W. Yang, Y. Fang, N. Ma, F. Pei, L. Zhao and Q. Hu, Antioxidant and cytotoxicites of Pleurotus eryngii residue polysaccharides obtained by ultrafiltration, LWT – Food Sci. Technol., 2016, 73, 108–116 CrossRef CAS.
- J. Hu, S. Nie, F. Min and M. Xie, Polysaccharide from seeds of Plantago asiatica L. increases short-chain fatty acid production and fecal moisture along with lowering pH in mouse colon, J. Agric. Food Chem., 2012, 60, 11525–11532 CrossRef CAS PubMed.
- K. Wang, W. Li, X. Rui, X. Chen, M. Jiang and M. Dong, Characterization of a novel exopolysaccharide with antitumor activity from Lactobacillus plantarum, 70810, Int. J. Biol. Macromol., 2014, 63, 133–139 CrossRef CAS PubMed.
- H. Mineo, N. Amita, M. Kawawake and A. Higuchi, Dicarboxylic acids with limited numbers of hydrocarbons stabilize cell membrane and increase osmotic resistance in rat erythrocytes, Biochim. Biophys. Acta, Biomembr., 2013, 1828, 2379–2384 CrossRef CAS PubMed.
- H. Ohno, Gut microbial short-chain fatty acids in host defense and immune regulation, Inflammation Regener., 2015, 35, 114–121 CrossRef CAS.
- P. Louis, G. L. Hold and H. J. Flint, The gut microbiota, bacterial metabolites and colorectal cancer, Nat. Rev. Microbiol., 2014, 12, 661–672 CrossRef CAS PubMed.
- H. Yuan, Y. Chen, H. Zhang, S. Jiang, Q. Zhou and G. Gu, Improved bioproduction of short-chain fatty acids (SCFAs) from excess sludge under alkaline conditions, Environ. Sci. Technol., 2006, 40, 2025–2029 CrossRef CAS PubMed.
- H. Sa'ad, M. P. Peppelenbosch, H. Roelofsen, R. J. Vonk and K. Venema, Biological effects of propionic acid in humans; metabolism, potential applications and underlying mechanisms, Biochim. Biophys. Acta, Mol. Cell Biol. Lipids, 2010, 1801, 1175–1183 CrossRef PubMed.
- N. Reichardt, S. H. Duncan, P. Young, A. Belenguer, C. M. Leitch, K. P. Scott, H. J. Flint and P. Louis, Phylogenetic distribution of three pathways for propionate production within the human gut microbiota, ISME J., 2014, 8, 1323–1335 CrossRef CAS PubMed.
- Y. Chung, C. Hsu, C. Ko and Y. Chan, Dietary intake of xylooligosaccharides improves the intestinal microbiota, fecal moisture, and pH value in the elderly, Nutr. Res., 2007, 27, 756–761 CrossRef CAS.
- P. J. Turnbaugh, R. E. Ley, M. A. Mahowald, V. Magrini, E. R. Mardis and J. I. Gordon, An obesity-associated gut microbiome with increased capacity for energy harvest, Nature, 2006, 444, 1027–1131 CrossRef PubMed.
- D. Mariat, O. Firmesse, F. Levenez, V. Guimarăes, H. Sokol, J. Doré, G. Corthier and J. Furet, The Firmicutes/Bacteroidetes ratio of the human microbiota changes with age, BMC Microbiol., 2009, 9, 123 CrossRef CAS PubMed.
- C. Vincent, M. A. Miller, T. J. Edens, S. Mehrotra, K. Dewar and A. R. Manges, Bloom and bust: intestinal microbiota dynamics in response to hospital exposures and Clostridium difficile colonization or infection, Microbiome, 2016, 4, 12 CrossRef PubMed.
- L. Bomar, M. Maltz, S. Colston and J. Graf, Directed culturing of microorganisms using metatranscriptomics, mBio, 2011, 2, e00012–e00011 CrossRef PubMed.
- H. Wang, Y. Li, X. Feng, Y. Li, W. Wang, C. Qiu, J. Xu, Z. Yang, Z. Li and Q. Zhou, Dysfunctional gut microbiota and relative co-abundance network in infantile eczema, Gut Pathog., 2016, 8, 36 CrossRef PubMed.
Footnote |
† Electronic supplementary information (ESI) available. See DOI: 10.1039/c7fo00341b |
|
This journal is © The Royal Society of Chemistry 2017 |
Click here to see how this site uses Cookies. View our privacy policy here.