DOI:
10.1039/C6FO01289B
(Paper)
Food Funct., 2017,
8, 111-121
Differentiated Caco-2 cell monolayers exhibit adaptation in the transport and metabolism of flavan-3-ols with chronic exposure to both isolated flavan-3-ols and enriched extracts†
Received
31st August 2016
, Accepted 13th October 2016
First published on 3rd November 2016
Abstract
The relatively low oral bioavailability of flavan-3-ols from acute doses is commonly highlighted as a limitation when considering the biological significance of these compounds. However, evidence suggests that the absorption of flavan-3-ols may be enhanced during periods of repeated exposure which is more representative of dietary patterns. To explore changes occurring in the upper small intestine from repeated exposure to dietary flavan-3-ols, Caco-2 human intestinal cells were cultured and differentiated in the presence of isolated flavan-3-ols epigallocatechin gallate (EGCG) and epicatechin (EC) or flavan-3-ol-rich green tea and grape seed extracts. Following differentiation, cellular accumulation, Phase II metabolism, and transcellular transport were assessed from a final acute dose of the respective pretreated compound or extract. 10 μM EGCG pretreatment significantly (P < 0.05) decreased the appearance rate of both sulfated and methyl sulfate EGCG metabolites compared to the control. In contrast, 10 μM EC pretreatment resulted in a significantly greater appearance of methylated EC from acute treatment. After 4 h, 10 μM green tea extract pretreatment resulted in a significant (P < 0.05) 38% greater cumulative transport of EC, in addition to 44–60% increased transport of EGCG and epicatechin gallate (ECG) at 60 min compared to the control. For monolayers pretreated with 10 μM grape seed extract, there was a significant (P < 0.05) 17–56% greater cumulative transport of C and EC after 4 h. Assessment of the mRNA expression of select xenobiotic and metabolizing genes revealed that pretreatment with green tea and grape seed extracts significantly (P < 0.05) increased the expression of COMT, ABCC2 and ABCB1. Overall, these results suggest that intestinal adaptation to both isolated flavan-3-ols and extracts rich in these compounds alters their intestinal transport and metabolism.
Introduction
Flavan-3-ols are key flavonoids widely distributed in food supplies and are naturally abundant in foods such as tea, cocoa, and grapes. These compounds include monomeric forms such as catechin (C) and epicatechin (EC), along with complex proanthocyanidin (PAC) oligomers and polymers, present in grapes and cocoa. Additionally, gallated forms including epigallocatechin gallate (EGCG) and epicatechin gallate (ECG) are abundant in tea. Data from epidemiological studies support the view that diets rich in flavan-3-ols are associated with a decreased risk of specific chronic diseases.1–3 Supporting these epidemiological associations, flavan-3-ols, and especially EC, have demonstrated the ability to improve clinical markers related to cardiovascular function such as blood pressure, cholesterol, and flow-mediated dilation.4,5 However, translation of flavan-3-ols for use in disease preventative strategies is limited by several factors including the notion that, excluding microbial metabolites, less than 5% of the parent compound is typically absorbed from an acute oral dose.6
While acute bioavailability studies are most common, evidence from human and animal studies indicates that repeated exposure to flavan-3-ols, more representative of widespread dietary exposure, can significantly enhance the overall bioavailability. The ten-day administration of a grape seed extract product (GSE) to Sprague-Dawley rats was found to improve the plasma response of C and EC by >250%.7 Similarly, chronic GSE treatment in rodents increased Phase II metabolites as much as 600–700% for C/EC glucuronides and approximately 140–240% for methyl-C/EC-glucuronides.8 In addition, clinical research has demonstrated that the 4-week administration of 800 mg EGCG per day before a pharmacokinetic assessment resulted in EGCG plasma AUC values 60% greater than those not exposed to EGCG.9 Collectively these data suggest that single acute pharmacokinetic assessments may not fully represent the complex response observed from longer-term dietary exposure. Having been observed in both pre-clinical and clinical paradigms, the implication of this adaption remains to be better understood. Then perhaps this adaptation can be leveraged in a manner consistent with understanding how flavan-3-ol bioavailability is altered with long-term dietary patterns.
While the exact mechanisms behind this phenomenon are not completely understood, what is known is that the upper intestinal absorption and metabolism of flavonoids rely on both inducible and non-inducible metabolizing and transport systems10 that may be impacted by previous exposure to these compounds. Since the gastrointestinal tract is the main barrier to the delivery of flavonoids, we hypothesize that adaptation occurring in the upper gastrointestinal epithelium is largely controlling the absorption of ingested compounds and may be partially responsible for the observed increase in flavan-3-ol absorption and metabolism with chronic exposure. Though gut microbiota have been reported to affect the systemic delivery of phenolic compounds,11 we are focusing here on the parent flavonoids and their Phase II metabolites since their relevance to bioactivity has been more widely studied.
Differentiated Caco-2 monolayers are highly predictive of human absorption and have been employed to study the absorption of a number of pharmaceuticals and phytochemicals because they differentiated into enterocyte-like cells.12 However, many studies involving the Caco-2 model are limited to acute studies and do not consider how chronic exposure typical of dietary patterns modulates cellular processes associated with transport across the gut epithelium. It is critical to better understand the extent to which such a widely applied gut model such as Caco-2 can be adapted to study such phenomena. To address this issue, the present study exposed Caco-2 cell monolayers throughout differentiation to isolated flavan-3-ols and extracts rich in flavan-3-ols to determine if adaptation in the transport and metabolism of these compounds could be induced.
Materials and methods
Materials
Standards of (−)-epicatechin, (+)-catechin, (−)-epigallocatechin gallate, and (−)-epigallocatechin were obtained from Sigma (St Louis, MO). Teavigo® (>90% EGCG) was used for cell culture treatments. Green tea extract (GTE) was provided by Nestlé (Marysville, OH) and grape seed extract (GSE) (MegaNatural-BP®, Polyphenolics, Madera, CA) was purchased from an online retailer. Solvents for liquid chromatography were of mass spectrometry grade and all other reagents were either cell culture or at least analytical grade.
Cell culture and treatments
Caco-2 TC7 cells (passages 81–84) were kindly provided by Dr James Fleet, Purdue University and were maintained in DMEM media with 10% v/v FBS, 1% v/v nonessential amino acids, 1% v/v HEPES, 1% v/v penicillin/streptomycin, and 0.1% v/v gentamicin. Cells were incubated at 37 °C under a 5% CO2/95% air atmosphere at constant humidity. For two compartment model experiments, cells were seeded at a density of 6.4 × 104 cells per well onto 6-well plates and allowed to differentiate over a period of up to 12 d post-confluency. For cells cultured on Transwell® inserts (Corning® polyester membrane, 24 mm diameter, pore size 0.4 μm), cells were seeded at a density of 4.8 × 104 cells per well and differentiated over a period of 21–25 d post-confluency. Cells treated with isolated compounds were cultured in growth media with 0 μM (control), 1 μM, or 10 μM of EC or EGCG added to the apical chamber (see ESI Fig. 1† for an outline of the experimental design). Preliminary experiments indicated that cell monolayers could be treated with these concentrations without significantly decreasing cell viability (>95%) as determined by MTT assay (Biotium, Hayward, CA). Cell monolayer integrity was confirmed by determining the transepithelial electrical resistance (TEER) values of the monolayers using the Millicell ERS-2 Voltohmmeter (EMD Millipore, Darmstadt, Germany) before assaying the transport of flavan-3-ols. Cells treated with extracts were cultured in growth media containing GTE rich in EGCG and ECG or GSE rich in C and EC monomers and polymers added to the apical chamber. Growth media with no added flavan-3-ols were placed in the basolateral chamber of all treatment groups. Media containing extracts were prepared using the same concentrations as pure compounds as determined by the Folin–Ciocalteu total phenolic method13 and changed every 48 h.
Flavan-3-ol transport, uptake, and metabolism
24 h before the flavan-3-ol uptake and metabolism was assessed, cell monolayers underwent a washout period where all treatment media were replaced with growth media containing no added flavan-3-ols (control media) to eliminate the possibility of any significant carry forward from pretreatment. Cell monolayers were rinsed with 0.1% fatty-acid free albumin in PBS, followed twice with PBS only before assessing the uptake and metabolism of pure compounds by an acute dose of either 50 μM EC or EGCG in PBS (adjusted to pH = 5.5 in order to prevent degradation of flavan-3-ols). Cells were harvested at 0 (media immediately removed after loading onto monolayer), 10, 30, 60, and 90 min. The transport of GTE and GSE flavan-3-ols using the Transwell® system was assessed by an acute dose of 100 μM total phenolics in PBS (pH = 5.5). 1 mL of PBS that was placed in the basolateral chamber was collected and replaced with fresh PBS at 0, 15, 30, 60, 120, and 240 min. Preliminary experiments indicated that cell monolayers could be treated at these concentrations over the experimental time period and the treatments were without significantly decreasing cell viability (>95%) as determined by MTT assay (Biotium, Hayward, CA). Experiments were performed in triplicate. After the uptake period, cells were rinsed according to the above procedure and then collected in ice cold PBS (pH = 5.5). The bicinchoninic acid (BCA) method using a Pierce BCA Protein Assay Kit (Thermo Fisher Scientific, Waltham, MA) was used to determine protein levels.
Cell extraction and transport media preparation
Flavan-3-ols and metabolites were extracted from cells as previously described.14 Briefly, cells were lysed by sonication for 10 s, after which 1 mL of PBS and MeOH were added. The cells were extracted three times with 0.01% w/v BHT/ethyl acetate, organic layers were pooled, and then evaporated under a nitrogen stream. The extract was resolubilized in 100 μL of 80
:
20 0.4% formic acid (aq.)
:
acetonitrile (0.1% formic acid) (v
:
v) and then centrifuged at 18
000g for 5 min to remove particulates before injecting 20 μL in the LC-MS-TOF system. Transport media were acidified with 1% v/v formic acid/acetonitrile, left on ice for 10 min to precipitate proteins, centrifuged, and injected as described above.
Characterization of flavan-3-ols and metabolites
The analysis of flavan-3-ols and their Phase II metabolites was performed according to a pervious method, with some modifications.15 Briefly, analytes were separated using a Waters RP-C18 column (Xbridge BEH Shield, 2.5 μm, 2.1 × 100 mm) and characterized using an Agilent 1100 high-pressure liquid chromatography system in tandem with a Waters LCT Premier time-of-flight mass spectrometer (LC-TOF-MS) with electrospray ionization (ESI). The flow was set to 0.25 mL min−1 and the mobile phases were A: 0.4% formic acid (aq.) and B: acetonitrile (0.1% formic acid). The gradient conditions were 5% B at 0 min, 35% B at 15 min, 70% B at 17 min, 5% B at 19 min, and 5% B at 23 min to reset the gradient. ESI was set to the negative mode and collected ion data from 100 to 1000 m/z in the V-mode. The capillary and cone voltages were set to 3500 and 35 V, respectively, while the desolvation and source temperatures were set to 250 and 150 °C, respectively. The cone and desolvation nitrogen gas flow rates were set to 60 and 400 L h−1. Extracted ion chromatograms (EICs) are shown in Tables 1 and 2. Calibration curves were produced using authentic standards of the parent flavan-3-ols (C, EC, EGCG, and EGC) using a concentration range of 0.10–20 μmol L−1. These curves were used to quantify intracellular/transported flavan-3-ols and to estimate the intracellular metabolite concentrations that were tentatively identified by LC-MS through calculations based on the peak area relative to the native flavan-3-ol standard curve.
Table 1 Green tea extract (GTE) and grape seed extract (GSE) transport buffers flavan-3-ol composition (100 μM total phenolics)a
Compound |
m/z for quantitation |
GTE μM ± SEM |
GSE μM ± SEM |
Values were obtained using a LC-TOF-MS system in the negative ionization mode and are displayed as average ± SEM (n = 3).
|
Catechin (C) |
289 |
4.83 ± 0.28 |
3.25 ± 0.36 |
Epicatechin (EC) |
289 |
21.9 ± 0.58 |
6.34 ± 0.71 |
Epigallocatechin (EGC) |
305 |
35.2 ± 1.1 |
Not quantified |
Epicatechin gallate (ECG) |
441 |
0.94 ± 0.029 |
Not detected |
Epigallocatechin gallate (EGCG) |
457 |
24.1 ± 0.69 |
Not detected |
Proanthocyanidin dimer B2 (PAC B2) |
577 |
Not detected |
2.49 ± 0.24 |
Total |
|
86.9 |
12.1 |
Table 2 Rate (pmol min−1) of differentiated Caco-2 cell accumulation of EGCG/EC and the appearance of EGCG/EC Phase II metabolites in pretreated monolayers compared to the controla
Compound |
MW |
Theoretical [M] − H |
Detected nominal m/z |
m/z for quantitation |
Retention time |
Pretreatment |
Rate of cell accumulation/appearance ± SEM (pmol per min per mg protein) over 90 min |
Values were obtained on a LC-MS system as indicated in the Materials and methods section and rate values are presented as average (n = 3) ± SEM. Statistical pairwise comparison is between pretreatments and 0 μM. *P < 0.05; **P < 0.01; #P = 0.0602.
|
EGCG |
|
|
|
|
|
0 μM |
3.67 ± 0.334 |
458 |
457 |
456.7 |
457 |
12.8 min |
1 μM |
3.67 ± 0.337 |
|
|
|
|
|
10 μM |
2.67 ± 0.334 |
Methyl-EGCG |
|
|
|
|
|
0 μM |
3.33 ± 0.334 |
472 |
471 |
470.7 |
471 |
14.6 min |
1 μM |
3.67 ± 0.334 |
|
|
|
|
|
10 μM |
3.00 ± 0.578 |
EGCG sulfate |
|
|
|
|
|
0 μM |
0.267 ± 0.0334 |
538 |
537 |
536.5 |
537 |
14.4 min |
1 μM |
0.367 ± 0.0667 |
|
|
|
|
|
10 μM |
0.0633 ± 0.0120** |
Methyl-EGCG sulfate |
|
|
|
|
|
0 μM |
0.233 ± 0.0334 |
552 |
551 |
550.5 |
551 |
14.5 min |
1 μM |
0.600 ± 0.0578** |
|
|
|
|
|
10 μM |
0.0700 ± 0.0173* |
EC |
|
|
|
|
|
0 μM |
3.00 ± 0.578 |
290 |
289 |
289.1 |
289 |
10.6 min |
1 μM |
6.00 ± 1.01# |
|
|
|
|
|
10 μM |
5.00 ± 4.33 |
Methyl-EC |
|
|
|
|
|
0 μM |
0.433 ± 0.0667 |
304 |
303 |
303.1 |
303 |
11.6 min |
1 μM |
0.833 ± 0.120* |
|
|
|
|
|
10 μM |
1.03 ± 0.0334** |
EC sulfate |
|
|
|
|
|
0 μM |
0.900 ± 0.0578 |
370 |
369 |
368.9 |
369 |
10.5 min |
1 μM |
2.00 ± 0.578 |
|
|
|
|
|
10 μM |
0.533 ± 0.0667* |
Methyl-EC sulfate |
|
|
|
|
|
0 μM |
0.0533 ± 0.0241 |
384 |
383 |
382.9 |
383 |
11.5 min |
1 μM |
0.153 ± 0.0467 |
|
|
|
|
|
10 μM |
0.0120 ± 0.00405 |
Gene expression
In order to determine the mRNA expression alteration of select xenobiotic transport and metabolizing system genes due to chronic exposure, a parallel set of Caco-2 cell experiments was conducted with cells treated with either GTE or GSE as previously described under “Cell culture and treatments.” After treatments, the cells were harvested, lysed, and RNA was isolated using RNeasy spin columns according to the manufacturer's directions (Qiagen Sciences, Germantown, MD). RNA was analyzed for its quality and quantity, reverse transcribed to cDNA, and then plated with primers for human genes COMT, ABCC2, the gene that codes for MRP2, and ABCB1, the gene that codes for P-glycoprotein (Bio-Rad Laboratories, Hercules, CA). Real-time PCR was conducted using a Bio-Rad CFX Connect™ real-time PCR system.
Data analysis
Values for the cellular accumulation of flavan-3-ols and metabolites are expressed as average pmol ± SEM. Linear regression using Excel (Microsoft, Redmond, WA) was performed to determine the slope for use in rate calculations. The apparent permeability coefficient (Papp) is used to assess the bioavailability of compounds in Caco-2 transport studies and was calculated using an equation previously described, with the main parameters being the rate of compound transport over time, the surface area of the Transwell®, and the concentration of the compound loaded in the apical chamber.16 Gene expression values are displayed as mean (n = 3) ΔΔCt fold-changes in mRNA expression relative to housekeeping genes. Analysis was performed using SAS 9.3 (SAS Institute, Cary, NC), and significant differences were evaluated by a Bonferroni post hoc pairwise test (α = 0.05).
Results
Monomeric flavan-3-ol composition of extracts and stability to experimental conditions
GTE shows a similar profile of flavan-3-ols as previously reported,14 with the major compounds being EGCG and EGC (Table 1). However, the overall concentrations of these compounds in the extract appear to have lower amounts of gallated with respect to non-gallated flavan-3-ols. The average recovery of GTE flavan-3-ols in PBS transport buffer was found to be 92.1% after 4 h. Table 1 shows the concentrations of C, EC, and PAC B2 in the GSE transport buffer (oligomers and polymers were not quantified), which were within the range of those previously reported.17 The average recovery of GSE flavan-3-ols in PBS transport buffer was 79.5% after 4 h.
Differential cellular uptake and metabolism of flavan-3-ols
In addition to unmetabolized EGCG and EC, methylated and sulfonated metabolites of these compounds were detected in Caco-2 cells following acute and chronic treatments. Fig. 1 displays representative LC-TOF-MS chromatograms of intracellular EGCG, EC, and tentative metabolite identification, which is similar to previous reports of the Caco-2 cell metabolism of flavan-3-ols.18,19 After 30 min, 1 μM EGCG pretreated monolayers showed a significantly (P < 0.05) greater accumulation of EGCG compared to the control, but no other differences in accumulation were observed (Fig. 2). While there was no alteration in the rate of EGCG uptake or methylated EGCG appearance with treatments, 10 μM EGCG pretreatment decreased the appearance rate of both sulfated (P < 0.01) and methyl sulfate derivatives (P < 0.05) compared to the control (Table 2). However, it is important to note that the resolution of EGCG metabolites was not as distinct as for EC metabolites. While the potential for some crossover in signals based on prevailing fragments between methyl and methyl sulfate metabolites exists, the selection and application of distinct m/z based on the parent ions for quantitation is believed to allow for consistent estimates of the intracellular and transported levels of these metabolites resulting in relative comparisons of experimental factors.
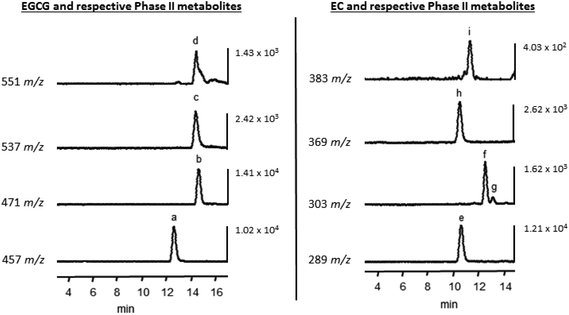 |
| Fig. 1 Representative LC-TOF MS chromatograms of intracellular EGCG, EC, and their respective metabolites with pretreatment. Tentative identification of peaks: a, EGCG; b, methyl-EGCG; c, EGCG sulfate; d, methyl-EGCG sulfate; e, EC; f, 3′-methyl-EC; g, 4′-methyl-EC; h, EC sulfate; i, methyl-EC sulfate. | |
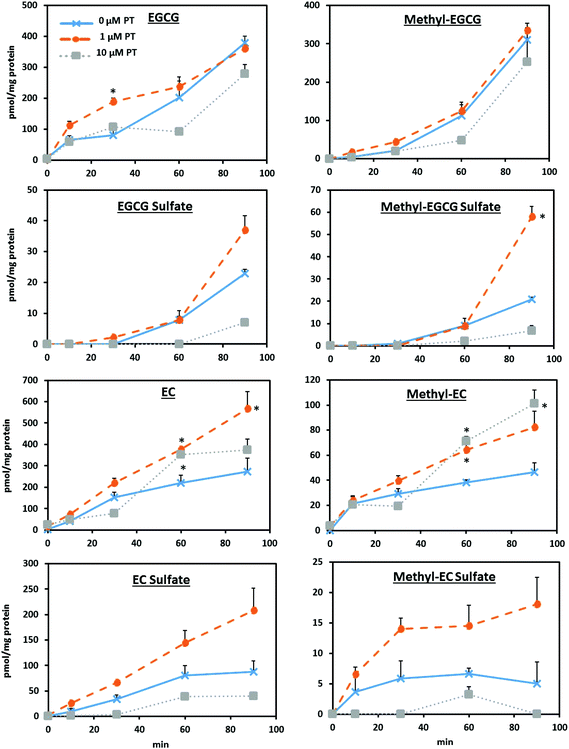 |
| Fig. 2 Effect of pretreatment on Caco-2 cell uptake and the metabolism of EGCG and EC over 90 min was assessed using monolayers differentiated in a 6-well two compartment model. Monolayers were exposed to either 0 μM (control), 1 μM, or 10 μM EGCG or EC pretreatment (PT) in growth media following the paradigm and conditions described in ESI Fig. 1.† All treatment groups were incubated with growth media containing no added flavan-3-ols 24 h before uptake and the metabolism was assessed using a 50 μM acute dose. The parent compounds, along with the methylated and sulfonated metabolites were tentatively identified by LC-TOF-MS. See Fig. 1 for representative chromatograms. *P < 0.05 compared to the control. | |
After 60 min, both 1 and 10 μM EC pretreatments resulted in significantly (P < 0.05) greater EC accumulation compared to the control, with the 1 μM pretreatment remaining significantly greater after 90 min. Similarly, both treatments displayed significantly (P < 0.05) greater levels of methylated EC derivatives after 60 min, with the 10 μM pretreatment showing approximately twice the concentration of methylated EC at 90 min (P < 0.05). The rate of the cell accumulation of EC and its metabolites over 90 min showed that while 10 μM pretreated monolayers had a significantly increased rate of EC methylation (P < 0.01), there was a decreased appearance of sulfated derivatives (P < 0.05). No significant differences were observed in the rate of appearance of methylated EC sulfate metabolites with pretreatment.
Differential alteration in the transport of flavan-3-ol-rich extracts across Caco-2 monolayers
Since major flavan-3-ol Phase II metabolites of the parent compounds were not observed to a great extent in the three compartment model, we focused on tracking the unmetabolized flavan-3-ols for flux through the cell monolayers. Fig. 3 shows that after 4 h, 10 μM GTE pretreatment resulted in a 38% greater cumulative transport of EC compared to the control (P < 0.05). GTE pretreatment resulted in significant (P < 0.05) differences in the transport of gallated flavan-3-ols at 60 min, with an approximately 60% greater cumulative transport of EGCG and a 44–61% increase in ECG transport. For monolayers pretreated with the 10 μM grape seed extract, there was a significantly (P < 0.05) greater cumulative transport of C, EC, and PAC B2 after 4 h (see Fig. 3). No significant differences were observed in the cellular accumulation of flavan-3-ols with tea or grape seed extract treatments (data not shown).
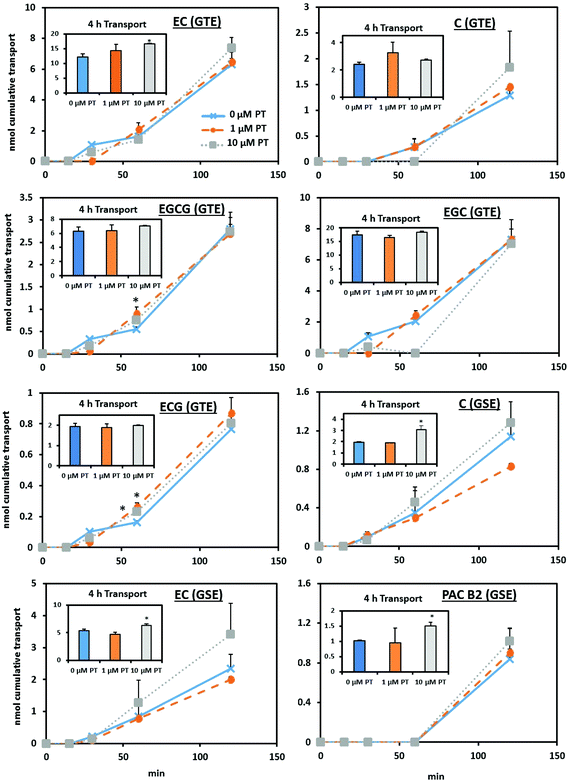 |
| Fig. 3 Chronic pretreatment with green tea extract differentially alters the apical to basolateral transport of flavan-3-ols across differentiated Caco-2 cell monolayers. Confluent Caco-2 monolayers were cultured on Transwell® inserts in pretreatment (PT) media containing either 0 μM (control), 1 μM, or 10 μM total green tea or grape seed phenolics (see Table 1 for the composition of transport media) during the differentiation process following the paradigm and conditions described in ESI Fig. 1.† All cell monolayers were incubated with growth media containing no added flavan-3-ols 24 h before transport was assessed using a 100 μM total phenolics acute dose of green tea or grape seed extract over 4 h. Native compounds were identified by LC-TOF-MS. Statistical pairwise comparison was performed comparing 0 μM (control) to treatments. *P < 0.05 compared to the control. Abbreviations: PT, pretreatment; C, catechin; EC, epicatechin; ECG, epicatechin gallate; EGC, epigallocatechin; EGCG, epigallocatechin gallate; PAC B2, proanthocyanidin B2. | |
Table 3 displays the apparent permeability coefficient (Papp) calculated from the transcellular flux of each parent flavan-3-ol compound from green tea and grape seed extracts. After 2 h, 1 μM GTE pretreated monolayers showed a greater (P < 0.01) Papp of C and EC compared to the control, though there was a trend in significance for increased EC Papp with 10 μM pretreatment (P = 0.0599). Similarly, significant (P < 0.05) differences in C transport were observed over 2 h in monolayers receiving 10 μM GSE pretreatment. Additionally, there was a trend (P = 0.0515) for increased PAC B2 Papp over 4 h with 10 μM GSE pretreatment. Interestingly, 10 μM pretreatment with both green tea and grape seed extracts significantly (P < 0.05) increased the TEER of differentiated monolayers compared to the control (Fig. 4).
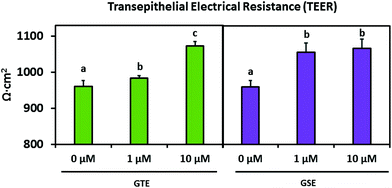 |
| Fig. 4 Pretreatment with enriched extracts increases the transepithelial electrical resistance (TEER) values. Caco-2 cells grown on Transwell® inserts (n = 3) were pretreated with either 0 (control), 1, or 10 μM total phenolics of green tea extract (GTE) or grape seed extract (GSE) over the course of the differentiation period (21–15 d). TEER values of cell monolayers were obtained immediately before transport experiments were performed. Differing letters indicate significant difference (P < 0.05) within the treatment type. | |
Table 3 Apparent permeability coefficients (Papp) of green tea (GTE) or grape seed (GSE) extract flavan-3-ols across differentiated Caco-2 cell monolayersa
Compound |
Pretreatment |
P
app ± SEM (×107 cm s−1) over 2 h |
P
app ± SEM (×107 cm s−1) over 4 h |
Values were obtained on a LC-TOF-MS system as indicated in the Materials and methods section and are presented as average (n = 3) ± SEM. Statistical pairwise comparison is between pretreatments and 0 μM. *P < 0.05; **P < 0.01; #P = 0.0588; ##P = 0.0991; †P = 0.0515.
|
C (GTE) |
0 μM |
4.93 ± 0.336 |
6.32 ± 0.274 |
1 μM |
7.05 ± 0.0966** |
6.48 ± 1.30 |
10 μM |
8.45 ± 3.24 |
5.86 ± 0.382 |
EC (GTE) |
0 μM |
5.23 ± 0.389 |
6.57 ± 0.652 |
1 μM |
7.15 ± 0.117** |
6.28 ± 0.798 |
10 μM |
7.64 ± 0.834# |
7.18 ± 0.105 |
EGCG (GTE) |
0 μM |
2.64 ± 0.322 |
2.47 ± 0.216 |
1 μM |
2.68 ± 0.370 |
2.51 ± 0.314 |
10 μM |
2.65 ± 0.157 |
2.72 ± 0.0715 |
ECG (GTE) |
0 μM |
9.18 ± 0.896 |
9.44 ± 0.801 |
1 μM |
10.8 ± 1.28 |
9.37 ± 1.03 |
10 μM |
9.87 ± 0.666 |
9.76 ± 0.211 |
EGC (GTE) |
0 μM |
4.80 ± 0.809 |
4.61 ± 0.243 |
1 μM |
5.04 ± 0.438 |
4.48 ± 0.117 |
10 μM |
4.97 ± 0.348 |
5.24 ± 0.163## |
C (GSE) |
0 μM |
6.54 ± 0.441 |
5.79 ± 0.285 |
1 μM |
4.75 ± 0.0912 |
5.47 ± 0.0237 |
10 μM |
7.46 ± 1.39* |
8.79 ± 1.04 |
EC (GSE) |
0 μM |
6.94 ± 0.980 |
7.90 ± 0.101 |
1 μM |
6.01 ± 0.222 |
6.89 ± 0.549 |
10 μM |
10.3 ± 3.05 |
9.47 ± 0.651 |
PAC B2 (GSE) |
0 μM |
6.02 ± 0.703 |
4.23 ± 0.0297 |
1 μM |
6.45 ± 1.79 |
3.84 ± 1.96 |
10 μM |
7.31 ± 0.896 |
6.07 ± 0.453† |
Differential changes in the mRNA expression of targeted xenobiotic transport and metabolizing systems
Fig. 5 displays alterations in the gene expression of xenobiotic metabolizing and transport systems with exposure to GTE or GSE 10 μM pretreatment plus 100 μM 4 h acute treatment compared to acute treatment only. These data indicate a significantly (P < 0.05) increased gene expression of COMT with both GTE (3.5-fold increase) and GSE (1.9-fold increase) pretreatment compared to acute treatment only. The ABCC2 mRNA expression significantly increased only with GTE exposure by 14.3-fold. The ABCB1 gene expression increased by 6.0-fold with GTE and 3.4-fold with GSE pretreatment.
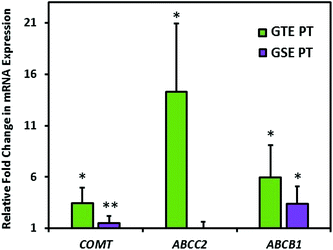 |
| Fig. 5 Pretreatment with enriched extracts increases the mRNA expression of targeted xenobiotic transport and metabolism genes. Caco-2 cells cultured on Transwell® inserts (n = 3) received a pretreatment (PT) of either 0 (control) or 10 μM total phenolics of green tea extract (GTE) or grape seed extract (GSE) during the differentiation process. After the pretreatment period, all treatments were dosed with 100 μM of the respective pretreated extract for 4 h, after which cells were harvested, lysed, and the RNA isolated for probing of the gene expression. Statistical pairwise comparison is between pretreatments compared to acute treatment only. *P < 0.05 and **P < 0.01. | |
Discussion
The Caco-2 human intestinal cell model is commonly used to investigate factors impacting the absorption and to a certain extent metabolism of dietary flavonoids. However, the typical experimental paradigms used in cell-based studies rely on acute exposures that may not mimic chronic dietary exposure to flavonoids. The extent to which the Caco-2 model can exhibit adaptive responses similar to those observed in humans and experimental animals is critical to establish in order to enhance the predictive nature of this important model. Our data suggest that Caco-2 cell human intestinal cell monolayers may, in fact, adapt differentially to the transport of gallated versus non-gallated flavan-3-ols with exposure to these flavonoids during differentiation. While non-gallated flavan-3-ols (C and EC) generally exhibited greater levels of cumulative transport at later time points with pretreatment (2–4 h; see Fig. 3), gallated flavan-3-ols (EGCG and ECG) showed differences in transport and accumulation at 30 and 60 min time points (see Fig. 2 and 3). Since gallated flavan-3-ols are less bioavailable as compared to the non-gallated ones,18 these points where alterations in transport and accumulation occurred may be a reflection of the different absorption efficiencies or perhaps transport mechanisms being affected by chronic exposure to gallated and non-gallated flavan-3-ols. Similarly, Faria et al. reported that Caco-2 cell monolayers pretreated with grape anthocyanins for 96 h exhibit approximately 50% greater transport of these compounds compared to untreated cells,20 indicating that different classes of flavonoids may induce an adaptive response.
Still, permeability coefficients of the transported flavan-3-ols (see Table 3) correspond with previous values reported in the literature18 and are indicative of their relatively low oral bioavailability (Papp < 2 × 10−5).16 Since pretreatment with both extracts significantly increased the TEER values, this suggests that pretreatment impacted tight junction proteins and that the observed increases in the flavan-3-ol transcellular flux are not mediated by paracellular transport. An increase in tight junction proteins has similarly been observed in rodents treated with the grape seed extract,21 which may be linked to this treatment resulting in a reduction of a marker of gut neutrophil infiltration in these animals.
Though there were no differences in intracellular methylated EGCG concentrations with pretreatment, there was a significant decrease in the formation rate of both sulfate and methyl sulfate metabolites with 10 μM pretreatment. These data suggest an inhibitory effect of flavan-3-ol pretreatment on these processes, as flavonoids have been reported to inhibit sulfotransferases.22 The expression of COMT was impacted by pretreatment with GTE and GSE (Fig. 5). However, the specific activity of these systems was not measured in the current study, nor was the expression of sulfotransferase enzymes. Further, the present findings appear to contrast with a report of HepG2 hepatocytes pretreated for 24–48 h with 10 μM resveratrol indicating an increased gene and protein expression of sulfotransferase enzymes.23 This suggests that the class of the phenolic compound used in treatments, length of treatment time, combined with tissue type may be critical to consider in assessing their influences on Phase II metabolizing systems. Though we observed transient increases in transport with EGCG pretreatment, an animal study with a two-week pretreatment of the 0.32% EGCG diet observed an approximately 50% reduction in EGCG plasma AUC.24 However, this study did not measure metabolites of the compound and it is possible that an enhanced metabolism could be responsible for the reduction in levels of the circulating native EGCG. In contrast, a clinical study with a two week run-in period providing 800 mg EGCG per day resulted in a ≈60% increase in plasma AUC compared to those not provided EGCG,9 indicating that there may be a differential response to pretreatment depending on the specifics of the experimental design.
In contrast to EGCG pretreatment potentially decreasing metabolizing systems, there were significantly greater concentrations of methylated EC metabolites with pretreatment at 60–90 min (Fig. 2). Since 10 μM pretreatment did not significantly increase the intracellular concentrations of EC but methylated EC did increase, the increase in methylated EC levels may not simply be due to increased intracellular levels of the parent compound but may in fact reflect induction of methylation systems or EC being preferentially trafficked to these systems for more efficient conjugation, which is corroborated by our gene expression data showing an increased COMT expression. However, this was not fully assessed in these experiments and remains to be investigated. In contrast, we observed a significantly lower appearance rate of EC sulfate metabolites with 10 μM pretreatment compared to the control (Table 2), suggesting differential regulation of the individual Phase II systems with pretreatment. Again, with the exception of COMT, the specific expression and activities of the metabolizing enzymes were not assessed in the current study. As such, additional experiments are required to fully assess the extent to which changes in phenolic compound metabolism occurring in intestinal tissues with chronic exposure may shift the metabolism to other tissues such as the liver. This shift in the metabolism may then result in an altered systemic metabolite profile.
Some individual time points in Fig. 2 and 3 appear to not follow what would otherwise be expected to be a dose–response relationship in this experimental design. However, this may be a reflection of the complexity of the overall system and the fact that all flavan-3-ol metabolites contributing to the overall flux may not have been detected using the analytical methodologies applied in this study. As such, the apparent adaptation observed in these systems may not fully reflect the in vivo situation. With that in mind, the average rate of transport and apparent permeability, which consider transport and accumulation over the experimental period overall, indicate a correlation between pretreatment and the observed response.
We did not detect glucuronide metabolites of flavan-3-ols using our model, though some25 but not others19 have been able to detect these metabolites. This may be related to differences in the analytical methods used in addition to varying cell culture conditions, treatment concentrations, and length of treatment times. In addition, we did not observe significant levels of metabolites in the three compartment model, which may limit the utility of this model in instances where phenolic compounds under investigation are highly metabolized in the gut. This lack of metabolites may be related to our use of extracts rather than isolated compounds in the three compartment model, since extracts may provide additional substrates that saturate the metabolizing systems.19
Our data revealed differences in the gene expression of select xenobiotic transport and metabolizing systems with repeated pretreatments of GTE and GSE (see Fig. 5). We observed increases in the mRNA expression of the Phase II metabolizing enzyme COMT with both GTE and GSE treatments, in addition to increases in the mRNA expression of xenobiotic transport genes ABCC2 and ABCB1. The observed increases in the mRNA expression of select metabolizing and transport genes may be due to the inducible nature of these systems. These results correspond to a previous work performed with 10 μM caffeic acid treatment from 3–6 h, which increased gene expression of both P-gp and MRP2 in Caco-2 cells.26 Tissue types other than the intestine appear to respond to pretreatment with phenolic compounds, as primary hepatocytes have an altered gene expression of organic anion transporting polypeptides (OATPs) with long-term exposure to select anthocyanins.27 However, considering the observed differences in the accumulation and transport of metabolites as a function of pretreatment, a more detailed profiling of changes in the expression and activity of metabolizing systems is warranted.
On comparing our results with in vivo models, we did not observe a similar magnitude of effects in the adaptive response to our cell model as compared to animal studies previously cited.7,8 This may be due, in part, to differences in the pretreatment concentrations used in the studies and the actual concentrations employed in the animal studies. Luminal concentrations in the cited animal studies are estimated to be in the order of ≈4.3 mM concentrations of monomeric (epi)catechins,28 in contrast to the lower pretreatment concentrations used in our study (1–10 μM). Thus our experimental paradigm may be more indicative of a modest dietary consumption of flavan-3-ols, while the animal study represents what may be occurring on exposure to amounts common in dietary supplements. Though the luminal concentration of flavonoids could potentially be higher from typical servings of foods,29 the relatively low doses chosen here were based on the preliminary screening of cytotoxicity and to mimic the more modest concentrations of flavan-3-ols achieved by typical consumption in the USA.30 Another point to consider is that though we did not quantify proanthocyanidins in the grape seed extract, they appear to be an important component in the observed adaptive response in animal models that occurs with chronic exposure to C/EC monomers and polymers.8
Since the differentiation of intestinal cells is influenced by its matrix,31 it is plausible that altering that environment will affect the differentiation process. In support of this, Caco-2 cells chronically treated with flavan-3-ol-rich grape seed and other grape products over ≈30 d exhibit differences in the expression of classic markers of differentiation such as sucrase-isomaltase and aminopeptidase N.32,33 Though the present experiments did not measure these or other markers of differentiation, we did observe increases in TEER values with treatment, which may indicate altered differentiation or a response to flavan-3-ols.
A limitation of this model is that it does not consider other tissues involved in the absorption and transport of flavonoids, such as the liver and kidney. In addition, it only focuses on adaptation occurring in the upper gastrointestinal tract, which does not incorporate changes in gut microbiota in the lower bowel that may occur with a flavonoid-rich diet. Ultimately, it is important to consider the absorption and metabolism of these compounds in the context of a diet, where there are likely multiple interactions between food components and individual flavonoids that can alter the metabolism of other compounds.18,34 Still, these results suggest that intestinal adaptation to chronic exposure to both isolated flavan-3-ols and extracts rich in flavan-3-ols can alter the flavan-3-ol transport and potentially intestinal barrier function.
Conclusion
Our data suggest pretreatment with isolated flavan-3-ols and extracts rich in these compounds are both able to elicit an adaptive response in the Caco-2 cell model that is directionally reflective of in vivo adaptations previously documented. Since this model is widely used to predict the bioavailability of phytochemicals, the experimental paradigm of chronic exposure could possibly allow for a more accurate assessment of the absorption and metabolism of these compounds from exposure due to broad dietary patterns. In addition to the increased transport of green tea and grape seed flavan-3-ols observed with pretreatment, differences in EGCG and EC metabolite profiles were also observed. These data are comparable to previous pre-clinical and clinical studies demonstrating adaptation with chronic exposure to these compounds. Though not directly assessed in the present experiments, the increased flux of flavan-3-ols observed after pretreatment suggests more efficient cellular trafficking of the compounds. More complex models incorporating aspects of Phase II metabolism other than intestinal metabolism may assist in elucidating alterations occurring at other tissue sites. In addition, future studies can further explore this question by determining further molecular mechanisms involved in this adaptive response. Taken together, these results demonstrate that the Caco-2 cell model may be used to model alterations in intestinal transport and metabolism that occur with chronic dietary exposure to select flavan-3-ols.
Acknowledgements
BWR is supported by the National Science Foundation (NSF) Graduate Research Fellowship Program under Grant No. DGE-1333468.
References
- A. Tresserra-Rimbau, E. B. Rimm, A. Medina-Remón, M. A. Martínez-González, R. de la Torre, D. Corella, J. Salas-Salvadó, E. Gómez-Gracia, J. Lapetra, F. Arós, M. Fiol, E. Ros, L. Serra-Majem, X. Pintó, G. T. Saez, J. Basora, J. V. Sorlí, J. A. Martínez, E. Vinyoles, V. Ruiz-Gutiérrez, R. Estruch, R. M. Lamuela-Raventós and PREDIMED Study Investigators, Inverse association between habitual polyphenol intake and incidence of cardiovascular events in the PREDIMED study, Nutr., Metab. Cardiovasc. Dis., 2014, 24, 639–647 CrossRef CAS PubMed.
- R. Zamora-Ros, N. G. Forouhi, S. J. Sharp, C. A. González, B. Buijsse, M. Guevara, Y. T. van der Schouw, P. Amiano, H. Boeing, L. Bredsdorff, F. Clavel-Chapelon, G. Fagherazzi, E. J. Feskens, P. W. Franks, S. Grioni, V. Katzke, T. J. Key, K.-T. Khaw, T. Kühn, G. Masala, A. Mattiello, E. Molina-Montes, P. M. Nilsson, K. Overvad, F. Perquier, J. R. Quirós, I. Romieu, C. Sacerdote, A. Scalbert, M. Schulze, N. Slimani, A. M. W. Spijkerman, A. Tjonneland, M. J. Tormo, R. Tumino, D. L. van der A, C. Langenberg, E. Riboli and N. J. Wareham, The association between dietary flavonoid and lignan intakes and incident type 2 diabetes in European populations: the EPIC-InterAct study, Diabetes Care, 2013, 36, 3961–3970 CrossRef CAS PubMed.
- R. Zamora-Ros, V. Fedirko, A. Trichopoulou, C. A. González, C. Bamia, E. Trepo, U. Nöthlings, T. Duarte-Salles, M. Serafini, L. Bredsdorff, K. Overvad, A. Tjønneland, J. Halkjaer, G. Fagherazzi, F. Perquier, M.-C. Boutron-Ruault, V. Katzke, A. Lukanova, A. Floegel, H. Boeing, P. Lagiou, D. Trichopoulos, C. Saieva, C. Agnoli, A. Mattiello, R. Tumino, C. Sacerdote, H. B. Bueno-de-Mesquita, P. H. M. Peeters, E. Weiderpass, D. Engeset, G. Skeie, M. V. Argüelles, E. Molina-Montes, M. Dorronsoro, M. J. Tormo, E. Ardanaz, U. Ericson, E. Sonestedt, M. Sund, R. Landberg, K.-T. Khaw, N. J. Wareham, F. L. Crowe, E. Riboli and M. Jenab, Dietary flavonoid, lignan and antioxidant capacity and risk of hepatocellular carcinoma in the European prospective investigation into cancer and nutrition study, Int. J. Cancer, 2013, 133, 2429–2443 CrossRef CAS PubMed.
- L. Hooper, C. Kay, A. Abdelhamid, P. A. Kroon, J. S. Cohn, E. B. Rimm and A. Cassidy, Effects of chocolate, cocoa, and flavan-3-ols on cardiovascular health: a systematic review and meta-analysis of randomized trials, Am. J. Clin. Nutr., 2012, 95, 740–751 CrossRef CAS PubMed.
- R. Sansone, A. Rodriguez-Mateos, J. Heuel, D. Falk, D. Schuler, R. Wagstaff, G. G. C. Kuhnle, J. P. E. Spencer, H. Schroeter, M. W. Merx, M. Kelm, C. Heiss and F. Consortium, European Union 7th Framework Program, Cocoa flavanol intake improves endothelial function and Framingham Risk Score in healthy men and women: A randomised, controlled, double-masked trial: the Flaviola Health Study, Br. J. Nutr., 2015, 114, 1246–1255 CrossRef CAS PubMed.
- A. P. Neilson and M. G. Ferruzzi, Influence of formulation and processing on absorption and metabolism of flavan-3-ols from tea and cocoa, Annu. Rev. Food Sci. Technol., 2011, 2, 125–151 CrossRef CAS PubMed.
- M. G. Ferruzzi, J. K. Lobo, E. M. Janle, B. Cooper, J. E. Simon, Q.-L. Wu, C. Welch, L. Ho, C. Weaver and G. M. Pasinetti, Bioavailability of gallic acid and catechins from grape seed polyphenol extract is improved by repeated dosing in rats: implications for treatment in Alzheimer's disease, J. Alzheimer's Dis., 2009, 18, 113–124 CAS.
- J. Wang, M. G. Ferruzzi, L. Ho, J. Blount, E. M. Janle, B. Gong, Y. Pan, G. A. N. Gowda, D. Raftery, I. Arrieta-Cruz, V. Sharma, B. Cooper, J. Lobo, J. E. Simon, C. Zhang, A. Cheng, X. Qian, K. Ono, D. B. Teplow, C. Pavlides, R. A. Dixon and G. M. Pasinetti, Brain-targeted proanthocyanidin metabolites for Alzheimer's disease treatment, J. Neurosci., 2012, 32, 5144–5150 CrossRef CAS PubMed.
- H.-H. S. Chow, Y. Cai, I. A. Hakim, J. A. Crowell, F. Shahi, C. A. Brooks, R. T. Dorr, Y. Hara and D. S. Alberts, Pharmacokinetics and safety of green tea polyphenols after multiple-dose administration of epigallocatechin gallate and polyphenon E in healthy individuals, Clin. Cancer Res., 2003, 9, 3312–3319 CAS.
- E. Croom, Metabolism of xenobiotics of human environments, Prog. Mol. Biol. Transl. Sci., 2012, 112, 31–88 CAS.
- F. A. Tomás-Barberán, R. García-Villalba, A. González-Sarrías, M. V. Selma and J. C. Espín, Ellagic acid metabolism by human gut microbiota: Consistent observation of three urolithin phenotypes in intervention trials, independent of food source, age, and health status, J. Agric. Food Chem., 2014, 62, 6535–6538 CrossRef PubMed.
- Y. Sambuy, I. De Angelis, G. Ranaldi, M. L. Scarino, A. Stammati and F. Zucco, The Caco-2 cell line as a model of the intestinal barrier: influence of cell and culture-related factors on Caco-2 cell functional characteristics, Cell Biol. Toxicol., 2005, 21, 1–26 CrossRef CAS PubMed.
-
A. L. Waterhouse, Determination of total phenolics, in Current Protocols in Food Analytical Chemistry, John Wiley & Sons, Inc., 2001 Search PubMed.
- A. P. Neilson, B. J. Song, T. N. Sapper, J. A. Bomser and M. G. Ferruzzi, Tea catechin auto-oxidation dimers are accumulated and retained by Caco-2 human intestinal cells, Nutr. Res., 2010, 30, 327–340 CrossRef CAS PubMed.
- B. J. Song, C. Manganais and M. G. Ferruzzi, Thermal degradation of green tea flavan-3-ols and formation of hetero- and homocatechin dimers in model dairy beverages, Food Chem., 2015, 173, 305–312 CrossRef CAS PubMed.
- I. Hubatsch, E. G. E. Ragnarsson and P. Artursson, Determination of drug permeability and prediction of drug absorption in Caco-2 monolayers, Nat. Protoc., 2007, 2, 2111–2119 CrossRef CAS PubMed.
- T. S. Villani, W. Reichert, M. G. Ferruzzi, G. M. Pasinetti, J. E. Simon and Q. Wu, Chemical investigation of commercial grape seed derived products to assess quality and detect adulteration, Food Chem., 2015, 170, 271–280 CrossRef CAS PubMed.
- L. Zhang, Y. Zheng, M. S. S. Chow and Z. Zuo, Investigation of intestinal absorption and disposition of green tea catechins by Caco-2 monolayer model, Int. J. Pharm., 2004, 287, 1–12 CrossRef CAS PubMed.
- B. Sanchez-Bridge, A. Lévèques, H. Li, E. Bertschy, A. Patin and L. Actis-Goretta, Modulation of (-)-epicatechin metabolism by coadministration with other polyphenols in Caco-2 cell model, Drug Metab. Dispos., 2015, 43, 9–16 CrossRef PubMed.
- A. Faria, D. Pestana, J. Azevedo, F. Martel, V. de Freitas, I. Azevedo, N. Mateus and C. Calhau, Absorption of anthocyanins through intestinal epithelial cells - Putative involvement of GLUT2, Mol. Nutr. Food Res., 2009, 53, 1430–1437 CAS.
- K. M. Goodrich, G. Fundaro, L. E. Griffin, A. Grant, M. W. Hulver, M. A. Ponder and A. P. Neilson, Chronic administration of dietary grape seed extract increases colonic expression of gut tight junction protein occludin and reduces fecal calprotectin: a secondary analysis of healthy Wistar Furth rats, Nutr. Res., 2012, 32, 787–794 CrossRef CAS PubMed.
- M. O. James and S. Ambadapadi, Interactions of cytosolic sulfotransferases with xenobiotics, Drug Metab. Rev., 2013, 45, 401–414 CrossRef CAS PubMed.
- A. Lançon, N. Hanet, B. Jannin, D. Delmas, J.-M. Heydel, G. Lizard, M.-C. Chagnon, Y. Artur and N. Latruffe, Resveratrol in human hepatoma HepG2 cells: metabolism and inducibility of detoxifying enzymes, Drug Metab. Dispos., 2007, 35, 699–703 CrossRef PubMed.
- K. D. James, S. C. Forester and J. D. Lambert, Dietary pretreatment with green tea polyphenol, (-)-epigallocatechin-3-gallate reduces the bioavailability and hepatotoxicity of subsequent oral bolus doses of (-)-epigallocatechin-3-gallate, Food Chem. Toxicol., 2015, 76, 103–108 CrossRef CAS PubMed.
- A. Rodriguez-Mateos, N. Toro-Funes, T. Cifuentes-Gomez, M. Cortese-Krott, C. Heiss and J. P. E. Spencer, Uptake and metabolism of (-)-epicatechin in endothelial cells, Arch. Biochem. Biophys., 2014, 559, 17–23 CrossRef CAS PubMed.
- Y.-J. Hong, S.-Y. Yang, M.-H. Nam, Y.-C. Koo and K.-W. Lee, Caffeic acid inhibits the uptake of 2-amino-1-methyl-6-phenylimidazo[4,5-b]pyridine (PhIP) by inducing the efflux transporters expression in Caco-2 cells, Biol. Pharm. Bull., 2015, 38, 201–207 CAS.
- J. Riha, S. Brenner, A. Srovnalova, L. Klameth, Z. Dvorak, W. Jäger and T. Thalhammer, Effects of anthocyans on the expression of organic anion transporting polypeptides (SLCOs/OATPs) in primary human hepatocytes, Food Funct., 2015, 6, 772–779 CAS.
- T. T. Kararli, Comparison of the gastrointestinal anatomy, physiology, and biochemistry of humans and commonly used laboratory animals, Biopharm. Drug Dispos., 1995, 16, 351–380 CrossRef CAS PubMed.
- A. Scalbert and G. Williamson, Dietary intake and bioavailability of polyphenols, J. Nutr., 2000, 130, 2073S–2085S CAS.
- S. A. Kim, L. V. Moore, D. Galuska, A. P. Wright, D. Harris, L. M. Grummer-Strawn, C. L. Merlo, A. J. Nihiser and D. G. Rhodes, Vital signs: Fruit and vegetable intake among children - United States, 2003-2010, Rhodes and Division of Nutrition, Physical Activity, and Obesity, National Center for Chronic Disease Prevention and Health Promotion, CDC, MMWR Morb. Mortal. Wkly. Rep., 2014, 63, 671–676 Search PubMed.
- T. Reya and H. Clevers, Wnt signalling in stem cells and cancer, Nature, 2005, 434, 843–850 CrossRef CAS PubMed.
- C. Laurent, P. Besançon, C. Auger, J.-M. Rouanet and B. Caporiccio, Grape seed extract affects proliferation and differentiation of human intestinal Caco-2 cells, J. Agric. Food Chem., 2004, 52, 3301–3308 CrossRef CAS PubMed.
- C. Laurent, P. Besançon and B. Caporiccio, Ethanol and polyphenolic free wine matrix stimulate the differentiation of human intestinal Caco-2 cells., J. Agric. Food Chem., 2005, 53, 5541–5548 CrossRef CAS PubMed.
- B. T. Zhu, J.-Y. Shim, M. Nagai and H.-W. Bai, Molecular modelling study of the mechanism of high-potency inhibition of human catechol-O-methyltransferase by (-)-epigallocatechin-3-O-gallate, Xenobiotica, 2008, 38, 130–146 CrossRef CAS PubMed.
Footnotes |
† Electronic supplementary information (ESI) available. See DOI: 10.1039/c6fo01289b |
‡ Current address: Plants for Human Health Institute, Department of Food, Bioprocessing and Nutrition Sciences, North Carolina State University, 600 Laureate Way, Kannapolis, NC 28081, USA. |
|
This journal is © The Royal Society of Chemistry 2017 |