Cerium(IV) oxide nanoparticles induce sublethal changes in honeybees after chronic exposure†
Received
2nd July 2017
, Accepted 1st October 2017
First published on 4th October 2017
Abstract
The high annual production and use of cerium(IV) oxide nanoparticles (nCeO2s) may lead to their atmospheric release and substantial deposition on plants. This poses a potential threat to pollinators. We investigated the effects of nCeO2-spiked food (2–500 mg L−1) on summer and winter honeybees (Apis mellifera carnica) after chronic 9 days' oral exposure. Acetylcholinesterase (AChE) and glutathione S-transferase (GST) activities were measured in different body compartments (heads, thoraces, and haemolymph). The activity of AChE was assessed in salt-soluble (SS) (containing soluble and membrane AChE) and detergent-soluble (DS) (predominantly membrane-bound AChE) fractions. Exposure of honeybees to nCeO2-spiked food had no significant effects on survival up to 500 mg L−1 (<10%), while significant biochemical alterations were evidenced already at 2 mg L−1. In summer honeybees, a significant increase in the activities of AChE in the SS fraction and GST was found, while AChE activity in DS fractions was decreased at nearly all exposure concentrations. An exception was the 250 mg L−1 exposure, where AChE activity in DS fractions was increased. The alteration of AChE in the DS fraction could be symptomatic for the affected neuronal system, while alterations of GST activity indicate detoxification processes. An apparent difference in response to nCeO2 was evidenced between the summer and winter honeybees, which is in line with their different physiology. We ascribe most of the observed effects to particulate nCeO2 because a negligible presence of Ce ion species was found in their food. We conclude that nCeO2 release into the environment, especially atmospherically deposited material, is a potential risk to honeybees.
Environmental significance
The scope of application and the annual production of nCeO2 are increasing. The terrestrial environment is considered to be the main recipient of nCeO2 emissions, mostly via atmospheric deposition and direct application of biosolids and sewage sludge to landfills and farmlands. Thus, pollinators, such as honeybees, may come in direct contact with nCeO2 through surface exposure, inhalation, and foraging on contaminated plant resources and water droplets. This study reveals for the first time that nCeO2 induces a number of sublethal effects on honeybees. Of particular significance is the observed alteration of acetylcholinesterase, which could be symptomatic for the affected cholinergic neuronal system. Previously it has been shown that a disruption of the honeybees' neuronal cholinergic signalling results in affected orientation, learning and navigation abilities, leading to failure to return to their hives. The results offer specific insight into the potential risk of nCeO2 release into the environment to honeybees, which may be translated to other ecologically and economically important pollinators.
|
1. Introduction
Cerium(IV) oxide nanoparticles (nCeO2) have been considered as one of the key engineered nanomaterials (NMs) for the production of fuel additives, catalysts, hydrogen storage materials, fuel cells, polishing materials, pigments in glass and ceramics, gas sensors, optical devices, and ultraviolet absorbers and in biomedicine.1,2 The annual production volume is estimated to be 100–1000 t.3 The broad applicability of nCeO2 is mainly due to its insolubility and redox catalytic properties originating from the presence of oxygen vacancies on its surface and the autoregenerative cycle of the two valence states of Ce: Ce3+ and Ce4+.2,4
There are multiple possible routes of nCeO2 release to the terrestrial environment due to the atmospheric deposition of diesel automobile exhausts,5 fly ash,6 and coal fly ash,7 application of biosolids and sewage sludge to landfills and farmlands, and waste disposal.2,6,8,9 At the moment there is no information on the realistic background concentrations of nCeO2 in the environment due to the limitations in separation and analytical methodologies, so modelling predicted environmental concentrations (PECs) is used instead.1,6 Gottschalk et al. modelled 9 NMs in the Danish environment, and the PEC for nCeO2 in the environmental compartment was 24–1500 ng kg−1 in natural soil, 10–530 ng kg−1 in agricultural soil, and 94–5100 ng kg−1 in sludge-treated soil, while the PEC in the technical compartment was 44–2300 μg kg−1 in sewage treatment sludge and 240–12
000 μg kg−1 in fly ash.6 Significantly higher estimated concentrations of nCeO2 (2–30 mg kg−1 in biosolids) were found in a waste water treatment plant in San Francisco.8
nCeO2 is considered to persist in soil due to its structural properties and association with soil particles, thus possibly entering the physical and biological cycles.1,9,10 Once in the soil, nCeO2 has been shown to be accumulated and translocated to edible tissues of various crops with minimal biotransformation.11–14 Due to the high atmospheric deposition of nCeO2 it is very likely that honeybees may come in contact with nCeO2 through surface exposure,1,15 inhalation,1,15 foraging on contaminated plant resources and water droplets.1,15–17 Namely, during foraging activities the majority of environmental compartments (airborne particulate matter, vegetation, water sources, soil) are randomly sampled by honeybees within a large radius (m–km) around the hive.18 Consequently, a variety of actively/passively gathered materials are brought into the hive, and also xenobiotics (e.g. NMs), potentially contaminating the hive and affecting all members of the colony.18 Sufficiently compromised colony function can result in colony failure.19 In the past years, a decline in honeybee populations and the occurrence of sudden honeybee colony losses have been reported worldwide.19,20 Both issues are multicausal, resulting from interacting and synergising stressors including exposure to xenobiotics, deficient food resources, impaired quality of the environment, parasitic insults and pathogenic infections.19,20 According to the foregoing, several behavioural and morphological features, mobility and wide flying range make honeybees good and sensitive indicators of environmental quality18,21 and thus a species of particular interest in terrestrial nanoecotoxicology.
No data on the effects of nCeO2 on honeybees can be found currently in the literature, but some studies were done on other hexapods. For example, Tourinho and co-authors reported that 4 weeks' exposure to nCeO2-spiked Lufa 2.2 soil (10–1000 mg kg−1 dry soil) had no effects on the survival and reproduction of springtails (Folsomia candida).22 No toxicity was recorded in Chironomus riparius larvae, despite substantial Ce accumulation in this organism after exposure to 1 mg L−1 nCeO2 in experimental freshwater ecosystem studies;23,24 only differences in teratogenicity were observed between treatments.24 Alaraby et al. showed both up- and down-regulation of selected Hsp genes, internalisation of nCeO2 in the gut epithelium and its presence in haemocytes of fruit fly (Drosophila melanogaster) larvae after exposure to nCeO2 applied in culture medium (0.01–10 mM, equivalent to 1.72–1721.1 mg L−1).25 In spite of these findings, the authors observed no toxic, genotoxic, and teratogenic effects on fruit fly larvae under their experimental conditions.25 For crickets (Acheta domesticus),13 bean beetles (Epilachna varivestis) and spined soldier bugs (Podisus maculiventris)14 only trophic transfer of nCeO2 through a food chain and accumulation were recorded while no toxicity was reported.13,14
The focus of our study was to investigate the effects of nCeO2 on honeybees (Apis mellifera carnica) after chronic 9 days' dietary exposure. For this purpose, we assessed the activities of two enzymes: acetylcholinesterase (AChE) and glutathione S-transferase (GST). AChE is a serine hydrolase that hydrolyses the neurotransmitter acetylcholine.26 Two forms of AChE, membrane and soluble, were identified in honeybees.27,28 The membrane AChE form is predominantly associated with the central nervous system (brain and ganglia), where it most likely functions in synaptic signal transmission.26,29 The soluble AChE form is present in the central and peripheral nervous system as well as in non-neuronal tissues in the thorax, abdomen, and legs of honeybees.30 A number of non-neuronal roles of AChE have been reported in invertebrates, such as in fertilisation,31 embryogenesis and development,32,33 tissue regeneration,34,35 brood rearing,36 stress response,37 and xenobiotic defence.30,38,39 However, the separation between soluble and membrane AChE activities in the homogenate is a very challenging task. Previously, the detection of soluble and membrane AChE has been done using native-PAGE gels and AChE activity staining30,40 and using western blotting and specific antibodies.30 However, the only study where the activities of soluble and membrane AChE were inspected after exposure to toxicant was reported by Badiou et al., where the relative activities of soluble/membrane AChEs were measured by scanning of non-denaturing electrophoretic gels after AChE staining.40 In the work presented here, we assessed the AChE in two fractions: the salt-soluble (SS) and detergent-soluble (DS) fractions according to Das et al.41 We presume that the DS fraction is composed of predominately membrane AChE because we extracted proteins from pellets containing membranes and the soluble phase was removed prior to extraction. As clearly demonstrated by Kim et al., the SS fraction contains both soluble and membrane AChEs.30
Glutathione S-transferase (GST) activity is a frequently used stress-related enzymatic biomarker.42,43 GSTs are phase II detoxifying enzymes and can be induced by numerous chemicals because of their active role in the detoxification of exogenous as well as endogenous substances.44 Regarding the effect of metals and NMs on the GST activity, various mechanisms of action were proposed. It is expected that GST activity may be either increased or decreased due to production of lipid hydroperoxides.45–47 Despite the fact that only about half as many genes for GSTs, carboxyl/cholinesterases, and P450 monooxygenases have been found in the honeybee genome compared to other insects,48,49 the honeybees' GST detoxification system is altered upon intoxication. Monitoring of GST activity was previously used to assess environmental quality21,50 and to investigate the toxic potential of NMs in honeybees after acute51 and chronic dietary exposure.47,52
The aim of our study was to investigate if nCeO2 affects the honeybees A. m. carnica after chronic 9 days' exposure. nCeO2 was tested because of its extensive commercial use,2,53 the possibility for honeybees to be exposed to nCeO21,15–17 and the lack of data on the potential hazard for honeybees. Furthermore, nCeO2 is insoluble in physiological media, which means that observed biological effects could be ascribed to particulate matter and not predominantly ions, such as in the case of ZnO NMs.47 The particular focus in this study was on the activities of AChE in SS and DS fractions in different honeybee body compartments (head, thorax, and haemolymph). Namely, we recently evidenced that the honeybee's AChE forms respond differently to chemical exposure, e.g. specific AChE inhibitor diazinon, when they are assessed in different tissue fractions and body regions.54 Survival and GST were studied to assess the physiological state of honeybees. Finally, the adsorption of nCeO2 on the head surface was inspected to explain whether the adsorption of nCeO2 on the mouth parts and antennae could physically impact the feeding behaviour of honeybees.
2 Materials and methods
2.1 Chemicals
Deionized water (dH2O) with a resistivity of 18.2 MΩ cm−1 (Milli-RX 45, MILLIPORE) was used for the preparation of solutions and dispersions.
Stabiliser-free uncoated spherical nCeO2s (batch number PROM-CeO2-20 nm-2306/5a) were supplied by NanoMILE PROM (Promethean Particles, Nottingham, UK, http://www.prometheanparticles.co.uk/) within the framework of the EU FP7 NanoMILE project as an aqueous dispersion in dH2O. The nCeO2s were synthesized using supercritical fluid synthesis followed by a post-synthesis washing step to remove any unreacted species. The particle concentration in the stock dispersion was 31 g L−1, the mean particle diameter (TEM) was 4.7 ± 1.4 nm (N = 140; JEOL JEM2100F), the Z-average size was 172.1 ± 1.705 nm, the polydispersity index (PDI) was 0.272 ± 0.009, and the zeta potential was 50.3 ± 0.719 mV (Malvern Zetasizer 5000).
Sucrose and chemicals for biochemical analyses were all of analytical grade and purchased from Sigma-Aldrich Co. (Darmstadt, Germany): Triton X-100, dibasic and monobasic potassium phosphate, sodium hydrogen carbonate (NaHCO3), 5,5′-dithiobis-2-nitrobenzoic acid (DTNB), acetylthiocholine chloride (ACh-Cl), 0,1-chloro-2,4-dinitrobenzene (CDNB), L-glutathione (reduced form, GSH), and bovine serum albumin, analytical standard (BSA). BCA Protein Assay Reagent A and BCA Protein Assay Reagent B were purchased from Pierce (Thermo Fisher Scientific, USA). Trace analytical-grade 65% nitric acid (HNO3) and 30% hydrogen peroxide (H2O2) were purchased from Carlo Erba (Milano, Italy) and used for sample digestion. Ce standard solution for inductively coupled plasma mass spectrometry (ICP-MS) was purchased from Perkin Elmer (Waltham, USA). 96-well flat base, transparent, polystyrene plates were used as test containers for enzyme assays (Sarstedt, Germany). Polypropylene + polyethylene 3 and 5 mL sterile syringes were supplied by Ecoject® (Dispomed, Germany). 1 μL glass micropipettes were from BRAND (Germany).
2.2 Characterisation of the nCeO2
All characterisation steps were performed on freshly prepared nCeO2 dispersions in a 1.5 M sucrose solution at concentrations of 0, 2, 10, 50, 250, and 500 mg L−1: measurements of the (1) pH (Thermo Scientific Orion Star A215 Benchtop pH/conductivity meter), (2) total Ce ion species concentration, (3) total Ce concentration, and (4) nCeO2 size using the dynamic light scattering (DLS) method (particle size analyzer VASCO, Cordouan technologies, France). The dispersion was mixed using a magnetic stirrer with no additional sonication.
For the analysis of the presence of free Ce ion species in the dispersions, nCeO2 stock dispersions at concentrations of 0, 2, 10, 50, 250, and 500 mg L−1 were ultracentrifuged at 250
000g for 1 h at 25 °C (Beckman Coulter L8-70 M class H preparative ultracentrifuge with a type 70.1 Ti rotor and 15 mL Beckman polyallomer bell-top quick-seal tubes). Afterwards the supernatants and the stock nCeO2 dispersions were acid digested in a Milestone Start D (Bergamo, Italy) microwave lab station equipped with an SK-10 high-pressure segmented rotor and 3 mL quartz microsampling inserts. The digestion mixture consisted of HNO3 (65%) and H2O2 (30%), 1
:
1. Digestion was conducted at 180 °C and 700 W power, with step 1 (heating) lasting 20 min, step 2 (constant temperature) lasting 15 min, and cooling for 45 min to 60 °C. The total Ce concentration in the individual samples before and after ultracentrifugation was measured using an Agilent 7500ce ICP-MS instrument (Agilent Technologies, Palo Alto, CA, USA). In parallel, we performed ultracentrifugation and ICP-MS measurements with a Ce standard at concentrations of 2, 10, 50, 250, and 500 mg L−1 prepared in 1.5 M sucrose to evaluate the adsorption of Ce onto the quick-seal tubes and the effectiveness of microwave-assisted digestion. The results are available in the ESI,† Table S1.
2.3 Collection and preparation of the honeybees
Carniolan honeybees Apis mellifera carnica, Pollman 1879 (Insecta, Hymenoptera: Apidae) used in the study originated from the hive at the Department of Biology, Biotechnical Faculty, University of Ljubljana, Ljubljana, Slovenia. They were maintained according to standard commercial techniques. Honeybees were obtained from an adequately fed, healthy, queen-right colony with a known history and physiological status and were not treated with any chemical substances at least 4 weeks prior to experiments. We used summer- and autumn-collected honeybees (hereafter summer and winter honeybees, respectively) from the same colony. The adult workers were collected in the morning of the experiment from honeycomb frames inside the hive using an aspirator. Collected honeybees were randomly allocated to test cages, and the cages were then randomly designated a treatment. Honeybees were provided ad libitum with dechlorinated water and 1.5 M sucrose solution during the collection. After the collection the cages with honeybees were transferred to an incubator (34 °C, 60 ± 5% RH), provided ad libitum with dechlorinated water only and left to starve for 2 h.
2.5 Experimental setup
The summer 9 day chronic feeding test was done in the middle of June 2015, when 150 summer honeybees were divided into 6 treatment groups of 22 to 28 honeybees and were treated with nCeO2 of the nominal concentrations 0, 2, 10, 50, 250, or 500 mg L−1 of sucrose solution. The autumn 9 day chronic feeding test was done at the end of September 2015, and the 91 winter honeybees were divided into 6 groups with 14 to 17 honeybees per group. Three groups of winter honeybees were fed the sucrose solution without nCeO2, while the other three groups of winter honeybees were treated with the sucrose solution containing nCeO2 with the nominal concentration of 250 mg L−1 sucrose solution. The choice for the nominal concentration of nCeO2 for the experiment with winter honeybees was based on the results with the summer honeybees. All test dispersions contained filter-sterilised 1.5 M sucrose (51.35% w/v) that was made by diluting analytical grade sucrose with dH2O. The 500 mg L−1 nCeO2 dispersion was prepared from the stock dispersion in 1.5 M sucrose solution and no additional sonication was applied. Successive dilutions were made from the 500 mg L−1 nCeO2 dispersion.
Each group of honeybees was placed into a separate wooden cage. Rectangular wooden cages (10 × 6 × 7 cm; length × width × height) with a steel wire mesh and sliding transparent glass were used as in Milivojević et al.47 Graduated 5 mL sterile syringes for single use with open ends were used as feeders. The honeybees in each test cage were supplied with two feeders, one containing the test solution and the other containing dechlorinated tap water, that were provided ad libitum and renewed daily. The feeder with food was refilled from prepared nCeO2 dispersions that were warmed to room temperature and well vortexed. The experiments were held in a climatic chamber (I-440 CK, Kambič d.o.o., Semič, Slovenia) in continuous darkness at a temperature of 34 ± 0.5 °C and 60 ± 5% RH, imitating hive conditions. Three identical setups containing no honeybees were used as external controls to control for the change in feeder weight due to evaporation, and the consumption was adjusted accordingly. Handling and observations were conducted in daylight. Every 24 h dead honeybees were counted and removed, and the food consumption per test group was monitored when the feeder was weighed and replaced with a new one. All the experiments were designed according to OECD test guidelines (October, 2016)55 and according to Medrzycki et al.56
At the end of exposure, the honeybees were chilled in a freezer at −20 °C until their movement slowed down and then were individually sectioned. During the section the three body segments were separated. The heads were left intact, whereas the wings and legs were carefully cut off from the thoraces. The head and thorax from each honeybee were snap-frozen in liquid nitrogen and stored at −40 °C, while the abdomens were discarded. From each treatment, a group of honeybees (5–7 individuals) were sampled for haemolymph. The haemolymph was taken from the dorsal blood vessel in the thorax region using 1 μL micropipettes. The average volume of sampled haemolymph was 4.5 ± 0.5 μL. Individual haemolymph samples were transferred into 120 μL of ice-cold potassium phosphate buffer (PPB; 50 mM, pH 7.0), stored at −40 °C, and soluble AChE activity was measured the next day.
Three summer honeybees' heads from the control and 50 mg L−1 nCeO2 treatment were cryodesiccated (Christ 2-4 Alpha, Christ, Germany), glued onto carbon adhesive discs with silver paste (SPI), sputter coated with gold (Sputter Coater SCD 050, BAL-TEC, Germany), and investigated using field emission scanning electron microscopy and energy dispersive X-ray spectroscopy (SEM–EDX) to check the surface contamination with nCeO2 (FE-SEM; Jeol JSM-6500F EDX; EDS/WDS Oxford Instruments INCA).
2.6 Biochemical tests
2.6.1 Preparation of homogenates.
To measure the enzymatic activities, an individual body segment, head or thorax, was homogenised for 1 min in 800 μL of ice-cold filter-sterilised PPB (50 mM, pH 7.0) using an UltraTurrax T10 basic homogenizer (speed 5; IKA, Germany). The homogenates were centrifuged for 15 min at 20
817g at 4 °C (5810 R, Eppendorf, Germany). After centrifugation, the supernatants were transferred into fresh microcentrifuges and used for following the activities of AChE in the salt-soluble (SS) fraction and GST. Pellets were resuspended in 300 μL of 0.5% Triton X-100 in ice-cold PPB (50 mM, pH 7.0). The resuspended pellets were incubated on ice for 30 min and afterwards centrifuged for 15 min at 20
817g at 4 °C. These supernatants were used for following the activity of AChE in the detergent-soluble (DS) fraction. The haemolymph samples in PPB (50 mM, pH 7.0) were centrifuged for 15 min at 20
817g at 4 °C to remove the cells. Our unpublished work showed that no difference was found when 0.5% Triton X-100 in PPB (50 mM, pH 7.0) was used which means that we probably assessed only the soluble AChE activity.
2.6.2 Analysis of enzyme activities and protein content.
2.6.2.1 AChE activities in salt-soluble and detergent-soluble protein fractions.
Specific AChE activities were measured in SS and DS fractions according to Ellman's method57 with minor modifications, using a VIS microplate reader (Anthos Zenyth 3000, Great Britain). Suitable working concentrations of DTNB and ACh-Cl were prepared immediately before use. Ellman's reagent was prepared by dissolving 91 mg of DTNB in 100 mL of PPB (250 mM, pH 7.4), and 37.5 mg of NaHCO3 were added. The solution was diluted to 1 L with dH2O and stored in a dark glass bottle at 4 °C. The reaction mixture per microtiter well was composed of 50 μL of PPB (50 mM, pH 7.0), 50 μL of sample, and 100 μL of Ellman's reagent with ACh-Cl containing 0.1148 mM of DTNB and 1 mM ACh-Cl as final well concentrations, respectively. The reactions were followed spectrophotometrically at 405 nm at 25 °C for 15 min. In the case of blank reactions, the supernatant was replaced by PPB (50 mM, pH 7.0) with or without 0.5% Triton X-100. AChE activity was expressed in nmoles of hydrolysed ACh-Cl per min per mg of proteins (ε405 = 13
600 M−1 cm−1).
For the analysis of the AChE activity in the haemolymph, the reaction mixture per microtiter well was composed of 60 μL of PPB (50 mM, pH 7.0), 40 μL of supernatant, and 100 μL of Ellman's reagent with ACh-Cl as described above.
All samples were measured in triplicate except haemolymph samples, which were measured in duplicate.
2.6.2.2 Analysis of GST activity.
The GST activity was determined according to the method described by Habig et al.58 using a VIS microplate reader (Anthos Zenyth 3000, Great Britain). The reaction mixture per well was composed of 50 μL of supernatant, 50 μL of CDNB (final concentration 1 mM), and 100 μL of GSH (final concentration 1 mM). The working solution of CDNB was dissolved in absolute ethanol to 50 mM, which was afterwards diluted in PPB (100 mM, pH 6.5) to the final concentration of 4 mM and protected from direct light. The working solution of GSH was prepared in PPB (100 mM, pH 6.5) immediately before use. In the case of blank reactions, the supernatant was replaced by 50 μL of PPB (100 mM, pH 6.5). The reaction was followed spectrophotometrically at 340 nm at 25 °C for 15 min. The GST activity was expressed in nmoles of conjugated GSH per min per mg of protein (ε340 = 9600 M−1 cm−1). All samples were measured in triplicate.
2.6.2.3 Analysis of protein content.
The concentration of proteins in the supernatants was measured using a modification of the bicinchoninic acid protein assay according to the manufacturer's manual (Pierce, Rockford, IL, USA). Different concentrations of BSA were used as a standard and were processed in the same manner as the samples. In the case of blank reactions, the supernatant was replaced by PPB (50 mM, pH 7.0), with or without 0.5% Triton X-100. The absorbance was measured at 562 nm using a microplate reader (Dynex Technologies, USA) after 30 min of incubation in the dark at 37 °C. All samples were measured in triplicate. The concentration of proteins in the heads and thoraces was presented as mg of protein per mg of fresh tissue and in the haemolymph as mg of proteins per μL of haemolymph (hereafter referred to as normalised protein content).
2.7 Data analysis
The recovery of the measured total Ce concentrations of stock nCeO2 dispersions (before ultracentrifugation) is the quotient of the measured Ce concentrations and nominal Ce concentrations expressed in %. The share of the free Ce ion species in the supernatant is the quotient of the measured Ce concentration in the supernatant after ultracentrifugation and the measured Ce concentration before ultracentrifugation expressed in %. The results of the enzyme activities were expressed as specific activities obtained by normalising the enzyme activities to the quantity of proteins. The blank replicates without the protein supernatant were followed and the mean rate of the absorbance change was subtracted from the measurements containing supernatants. The protein concentration of individual samples was determined using a standard curve for proteins that was prepared by plotting the average blank-corrected measurement for each BSA standard versus its concentration. Significant differences in enzyme activities and normalised protein content between controls and treated samples were determined using the Mann–Whitney test, where p < 0.05 was considered to be significantly different. Data were calculated using Microsoft Excel (2010) and OriginPro software (OriginPro 8, OriginLab Corporation, US). The experiments were considered valid if the mortality in controls did not exceed 15% at the end of the test.55
3. Results
3.1 Characteristics of the nCeO2 exposure dispersions
All measured Ce concentrations were within <20% of the nominal concentrations; therefore we refer to nominal Ce concentrations throughout the manuscript (Table 1) (as recommended in the OECD test 202).59 To determine the share of free Ce ion species in nCeO2 dispersions, we measured the total Ce concentrations in the supernatants after ultracentrifugation of the nCeO2 stock dispersions. These values were very low, since the share of free Ce ion species was in the range of 0.086–0.82% of the measured total Ce concentrations (8.59–357.63 mg L−1) (Table 1). The only sample with a higher share of Ce ions species had a concentration of 2 mg L−1, where 7.52% of Ce was measured as free Ce. Nevertheless, this concentration was still very low (0.13 mg L−1) (Table 1). A parallel test with Ce standard prepared in 1.5 M sucrose proved that the ultracentrifugation and microwave-assisted digestion were successful and no adsorption of Ce onto the test tubes was observed (ESI,† Table S1).
Table 1 The combined results of the characterisation of the nCeO2 dispersions in 1.5 M sucrose solution. The table shows the measured total Ce concentration before and after ultracentrifugation in acid-digested samples, calculated recovery (%), calculated share of the free Ce ion species (%), hydrodynamic size (nm), polydispersity index (PDI), and pH. Hydrodynamic size (in nm) is given as the Z-average size, Z-average mean size, and size determined using the Pade–Laplace algorithm (PLA)
nCeO2 nominal conc. (mg L−1) |
nCeO2 calculated conc. (mg L−1) |
Ce nominal conc. (mg L−1) |
Ce measured conc. (mg L−1) |
Recovery (%) |
Free ion species (%) |
Hydrodynamic size |
PDI |
pH |
Size-PLA (nm) |
Z-average size (nm) |
Z-average mean size ± SD (nm) |
The background Ce concentration in 1.5 M sucrose solution.
|
nCeO2 dispersion before ultracentrifugation |
0 |
0 |
0 |
0.0009 |
— |
— |
— |
— |
— |
— |
5.57 |
2 |
2.13 |
1.63 |
1.73 |
106.13 |
— |
751 |
1078 |
1756 ± 923 |
1.106 |
5.35 |
10 |
10.55 |
8.14 |
8.59 |
105.53 |
— |
903 |
1023 |
1343 ± 513 |
0.584 |
5.29 |
50 |
53.03 |
40.70 |
43.17 |
107.47 |
— |
991 |
1214 |
1935 ± 992 |
1.051 |
5.38 |
250 |
227.93 |
203.52 |
185.55 |
91.17 |
— |
1138 |
1531 |
3212 ± 2256 |
1.973 |
5.12 |
500 |
439.30 |
407.04 |
357.63 |
87.86 |
— |
946 |
1104 |
1519 ± 632 |
0.692 |
4.98 |
After ultracentrifugation |
0 |
— |
0 |
0.0019a |
— |
— |
|
— |
— |
— |
5.79 |
2 |
— |
1.63 |
0.13 |
— |
7.52 |
|
— |
— |
— |
7.58 |
10 |
— |
8.14 |
0.07 |
— |
0.82 |
|
— |
— |
— |
7.48 |
50 |
— |
40.70 |
0.28 |
— |
0.65 |
|
— |
— |
— |
7.24 |
250 |
— |
203.52 |
0.16 |
— |
0.086 |
|
— |
— |
— |
6.86 |
500 |
— |
407.04 |
1.24 |
— |
0.35 |
|
— |
— |
— |
4.83 |
The DLS measurements showed that the largest hydrodynamic diameter of nCeO2 was found at 250 mg L−1, while the smallest hydrodynamic diameter was measured at 2 mg L−1 (Table 1). The nCeO2 dispersions had a broad size distribution according to the polydispersity index (PDI) (Table 1).
The pH of the stock nCeO2 dispersions decreased with increasing concentration, indicating a binding interaction between sucrose and nCeO2 particles (complex formation and/or adsorption of sucrose molecules onto the nCeO2 particle surface) which led to deprotonation of the former and consequently lower pH. This is also in accordance with the relatively high hydrodynamic diameter (HD) values increasing gradually from 751 nm at 2 mg L−1 up to 1138 nm at 250 mg L−1 nCeO2. However, the observed HD maximum at 250 mg L−1 is due to the optimal ratio between the sucrose and nCeO2 concentrations.
3.2 Adsorption of the nCeO2 onto the body surface
No notable surface adsorption of nCeO2 onto the head was observed at 50 mg L−1 nCeO2 (Fig. 1B–F) in comparison to control (Fig. 1A). The detailed results of the SEM–EDX analysis are available in the ESI† (annex 1 for Fig. S1†).
 |
| Fig. 1 SEM micrographs of a honeybee's head parts after 9 d exposure to control (A, whole head) or nCeO2-piked food (50 mg L−1) (B and C, base and end of antenna, respectively; D, compound eye; E and F, mouth parts). Thorough SEM–EDX analysis revealed no surface contamination with cerium (nCeO2). The detailed results of the SEM–EDX analysis are available in the ESI† (annex 1 for Fig. S1†). | |
3.3 Mortality and behaviour
The mortality of honeybees was below 10% in all treatment groups, except for the 50 mg L−1 group (summer honeybees) where 12.5% mortality was found (ESI,† Table S2). Honeybees treated with nCeO2-spiked food for 9 d did not display any signs of altered behaviour (visual observation) except in the case of summer honeybees exposed to 250 mg L−1 nCeO2. In this group the honeybees were agitated and hyperactive.
3.4 Effects on AChE and GST activities
The data for summer and winter honeybees are presented separately to show the differences in their response (Fig. 2 and 3). In summer honeybees, the AChE activity in the SS fraction was significantly increased in the heads of the 10–500 mg L−1 groups (Fig. 2A) and in the thoraces exposed to 50–500 mg L−1. A significant decrease in the AChE activity in the SS fraction was observed only in the thoraces of the 10 mg L−1group (Fig. 2B). On the other hand, the head AChE activity in the DS fraction was significantly decreased in the case of 10 and 500 mg L−1 nCeO2, while a significant increase was found only in the case of 250 mg L−1 (Fig. 2C). In the thoraces, the AChE activity in the DS fraction was significantly decreased in the 2–50 mg L−1 groups, and again a significant increase in activity was found in the 250 mg L−1 group (Fig. 2D). In the haemolymph, the AChE activity was significantly decreased in the case of the 2 and 10 mg L−1 groups (Fig. 2E). The GST activity in both heads and thoraces was significantly increased in all treatment groups compared to control (Fig. 3).
 |
| Fig. 2 Effects of the 9 day exposure to nCeO2-spiked food on the acetylcholinesterase (AChE) activities in salt-soluble (SS) and detergent-soluble (DS) fractions in the heads (A and C), thoraces (B and D), and haemolymph (E) of the summer and winter honeybees Apis mellifera carnica. Symbols on the box plot represent maximum and minimum values (whiskers: ⊥), mean values (□), and outliers (-); n represents the number of specimens in each test group (n =). Statistical differences between the control and the test groups and between controls of summer and winter honeybees with the Mann–Whitney test: p < 0.05 (*), p < 0.01 (**), p < 0.001 (***); (↑) increased and (↓) decreased AChE activity. | |
 |
| Fig. 3 Effects of the 9 day exposure to nCeO2-spiked food on the glutathione S-transferase (GST) activity responses in the heads (A) and thoraces (B) of the summer and winter honeybees Apis mellifera carnica. Symbols on the box plot represent maximum and minimum values (whiskers: ⊥), mean values (□), and outliers (-); n represents the number of specimens in each test group (n =). Statistical differences between the control and the test groups and between controls or 250 mg L−1 treatments of summer and winter honeybees with the Mann–Whitney test: p < 0.001 (***); (↑) increased and (↓) decreased GST activity. | |
In winter honeybees the exposure to 250 mg L−1 nCeO2 significantly increased the AChE activity in both fractions and the GST activity only in the thoraces (Fig. 2B and D and 3). The exposure had no significant effects on the activity of the head AChE in both fractions (Fig. 3A and C), but the GST activity was significantly inhibited (Fig. 3). The haemolymph AChE activity was unchanged in 250 mg L−1 nCeO2-treated winter honeybees compared to the control group (Fig. 2E).
When comparing enzyme activities in the control groups of summer and winter honeybees a statistical difference was observed. Overall, the control group of winter honeybees had a higher baseline activity for both fractions of AChE in the heads, AChE in the SS fraction in the thoraces, and GST in the heads, and a much lower protein content in the heads, thoraces, and haemolymph compared to the control group of summer honeybees (Fig. 2–4).
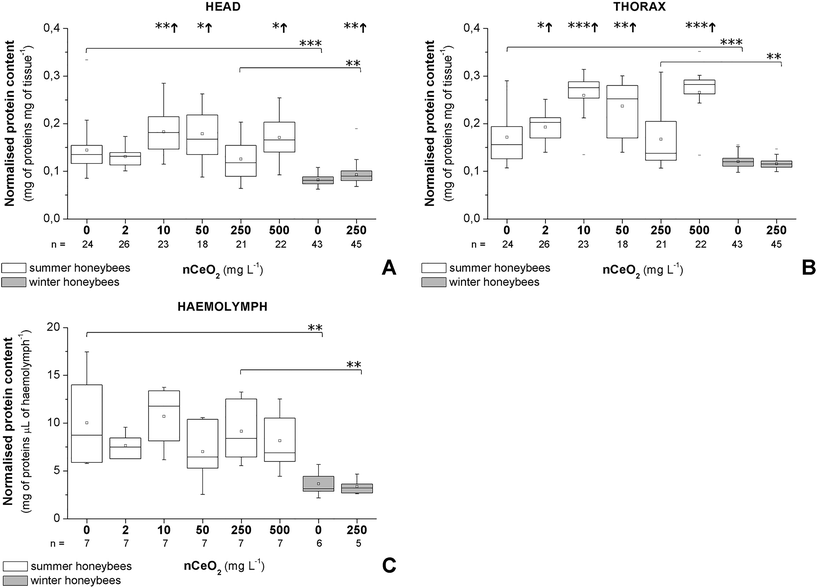 |
| Fig. 4 Effects of the 9 day exposure to nCeO2-spiked food on normalised protein content in the heads (A), thoraces (B), and haemolymph (C) of the summer and winter honeybees Apis mellifera carnica. Symbols on the box plot represent maximum and minimum values (whiskers: ⊥), mean values (□), and outliers (-); n represents the number of specimens in each test group (n =). Statistical differences between the control and the test groups and between controls or 250 mg L−1 treatments of summer and winter honeybees with the Mann–Whitney test: p < 0.05 (*), p < 0.01 (**), p < 0.001 (***); (↑) increased normalised protein content. | |
3.5 Effects on protein content
A clear difference was observed in the normalised protein contents of summer and winter honeybees in the control and in the 250 mg L−1 nCeO2 groups (Fig. 4A–C). Overall, the summer honeybees had higher baseline normalised protein content in heads, thoraces, and haemolymph (Fig. 4A–C).
The normalised protein content in the heads was increased in the 10, 50, and 500 mg L−1 groups of summer honeybees and in the 250 mg L−1 group of winter honeybees (Fig. 4A). Similarly, it was increased in the thoraces of the 2, 10, 50, and 500 mg L−1 groups of summer honeybees, but it was decreased in the 250 mg L−1 group of winter honeybees (Fig. 4B). In the haemolymph of summer and winter honeybees, the normalised protein content in the honeybees from the treated groups did not significantly differ from that of control groups (Fig. 4C).
4. Discussion
Chronic 9 day exposure of honeybees to nCeO2-spiked food had no noticeable effects on the survival of honeybees up to 500 mg L−1 exposure concentration, while significant alterations of biochemical parameters were evidenced. Very dynamic responses in enzymatic activities were observed in summer honeybees, i.e. increase in AChE activity in the SS fraction and GST activity and decrease in AChE activity in the DS fraction, with the exception of exposure to 250 mg L−1 nCeO2, where all activities were significantly increased. In winter honeybees, the alterations of enzyme activities were not as pronounced as in the case of summer honeybees.
In the case of other metal and metal oxide NMs, metal ion species were often identified as the predominant inducers of observed toxic effects.60 This was also the case in our recent study where honeybees were exposed for 10 d to ZnO NMs or ZnCl2-spiked food (1000 mg L−1).47 We related the observed effects primarily to Zn2+ ions originating from either ZnO NM or Zn salt. Namely, ZnO NMs are known to be unstable in aqueous dispersion, leading to dissolution and release of Zn2+ ions.53 On the contrary, nCeO2 is known for its insolubility,61,62 but Ce ions could have remained in the suspension as residues from the synthesis. Our results unequivocally show that this was not the case. The shares of free Ce ion species in the nCeO2 dispersions were overall very low (below 1.2 mg L−1). Another potential source of Ce ions could be the dissolution of nCeO2 inside the digestive tract. However, the breakdown of nCeO2 requires extreme conditions;63 therefore it is not likely that nCeO2 could dissolve inside the digestive tract of honeybees where a pH range of 5.7–6.0 is expected.64 The nCeO2 were uncoated and the dispersion was stabiliser-free, meaning there was no functional groups or other supplemental chemicals that could affect the honeybees. Consequently, the effects observed in our study can be attributed to the particulate form of nCeO2 only.
In our study the main nCeO2 exposure route that led to the observed effects on honeybees was attributed to oral intake. We found no adsorption of nCeO2 on the head surface, which means that the honeybees were not exposed via the surface. This also reveals that nCeO2 did not physically impair the feeding behaviour of honeybees by adsorption onto the antenna or mouth parts. The honeybee's gut epithelium is partly lined by a thick cuticle and in one part protected by a peritrophic envelope; therefore no direct contact between ingested material and epithelium is expected.65 Experimental evidence on various insects shows that there is a particle diameter limitation to pass the peritrophic envelope within the particle size range from 4.5 to 35 nm.65,66 Due to the size of nCeO2s in our study, they were probably not passively translocated through the peritrophic envelope of adult honeybees. In addition, because NMs aggregate and agglomerate in complex media, there is an even lower probability for passive passage through the intestinal barrier. However, a possible entrance of NMs exists when the peritrophic and epithelial barriers are compromised. In the case of a damaged peritrophic membrane only, NMs could still be potentially internalised through endocytotic pathways.67 This route of entry is well known from bacterial infections.68 The only existing study showing crossing and internalisation of nCeO2 in the insect gut epithelium and its presence in haemocytes was done on larvae of fruit flies.25 These authors reported the ability of haemocytes to phagocytose nCeO2.25 The latter results suggest that haemocytes have a role in removing the particles from haemolymph.25 Whether the passage of nCeO2 through the honeybee's gut epithelium occurs is a matter of further investigation. However, even if nCeO2 had not passed through the gut epithelium barrier, a number of effects on the organism are possible. For example, nCeO2 may cause inflammation due to its high oxidizing capacity,1 damage the gut epithelium and interfere with the process of digestion and nutrient uptake, leading to nutrient deprivation.16,23,24
In our study, we evidenced a complex response of AChE and GST activities in different honeybee body compartments. We observed an elevation of GST activities in the heads and thoraces of summer honeybees after the 9 d exposure to nCeO2 in all exposure groups and in thoraces of winter honeybees. One of the possible explanations for the increase in GST activity is a response to increased oxidative damage induced by reactive oxygen and nitrogen species generation, although there are opposing data regarding the potential of nCeO2 to induce oxidative stress.2,4In vivo studies on different organisms indicated that nCeO2 induced oxidative stress (reviewed by Malev et al.).69 On the other hand, the antioxidant action of nCeO2 was shown in in vivo25,70 as well as in vitro studies.71,72 Unexpectedly, we observed an inhibition of GST in the heads of winter honeybees exposed to 250 mg L−1, but we cannot explain the mechanism of this inhibition. We also observed a complex increase or decrease of protein contents in the head and thoraces of nCeO2-treated summer honeybees, which were concentration dependent but are difficult to interpret. However, it was previously suggested that alterations in the amount of proteins could be a result of different energy-demanding compensatory mechanisms induced by NMs.73
The particular focus in this study was on the activities of AChE in SS and DS fractions in different honeybee body compartments (head, thorax, and haemolymph). It has been previously shown that the DS fraction is composed of predominately membrane AChE, while the SS fraction contains both soluble and membrane AChEs.30 The exposure of summer honeybees to nCeO2 resulted in dual alterations (decrease and increase) of AChE activity in the DS fraction: at 10, 50, and 500 mg L−1 in the head and at 2, 10, and 50 mg L−1 in the thorax, AChE activity in the DS fraction was decreased, and at 250 mg L−1, the AChE activity in the DS fraction was increased in both body regions. The AChE activity in the DS fraction of winter honeybees exposed to 250 mg L−1 was not changed in the heads, but it was significantly increased in the thoraces. Decrease of membrane AChE is most commonly explained as a direct action of a chemical substance on the active site,74 but other mechanisms are also possible such as modifications of the lipid membrane leading to conformational changes of membrane AChE.75 However, as there are no data in the literature describing a direct interaction of nCeO2 with the AChE, the observed alteration of membrane AChE, either increased or decreased activity, is probably a consequence of indirect nCeO2 effects resulting from a complex systemic response. For example, stress signal transduction in the gut epithelium could mediate the general stress response as is well known from microbial infection studies.68 Interestingly, at 250 mg L−1 nCeO2 where the AChE activities in both fractions were the highest, the summer honeybees were hyperactive and were evidently agitated during the second half of the feeding test. This indicates that significantly increased membrane AChE could also be interpreted as an affected neuronal system evidenced as altered behaviour. Similarly, in another study, the hyperactivity (tumbling and trembling) was accompanied by increased activity of the total AChE when honeybees were exposed to neonicotinoid.76 We are not able to explain why the highest effect on enzymes was observed at 250 mg L−1, but our results show that this test dispersion significantly differed from others in terms of hydrodynamic diameter.
The most evident alterations in AChE activities in the SS fraction were noticed in summer honeybees. Since the SS fraction consist of both the soluble and the membrane AChEs30 we cannot ascribe this response to either of them alone. Considering the relevantly higher in vitro catalytic activity of membrane AChE as compared to soluble AChE,30 the AChE activity in SS fractions could be ascribed predominantly to membrane AChE. If this were the case, alterations of AChE activity in both fractions, SS and DS, would be similar after the exposure to nCeO2, both either diminished or elevated. However, we found that a significantly different pattern of response was observed between the SS and DS fractions, which may imply that a soluble AChE activity in the SS fraction was increased upon exposure to a stressor. Namely, this has been previously suggested,30,36,38,54,77 but it needs to be further investigated. We also assessed the AChE activity in the honeybee haemolymph, which has not been reported previously. The physiological role of haemolymph AChE is still not completely clear, but a possible role could be in anti-inflammatory actions.78 Our results however showed no clear dose–response of haemolymph AChE in honeybees exposed to nCeO2.
We observed that nCeO2 induced different alterations of biochemical parameters in summer and winter honeybees. The activities of AChE and GST in untreated honeybees were higher in winter honeybees in comparison to the summer honeybees. Differences in AChE activities in various developmental stages of honeybees were also observed by others.79,80 Shapira et al. ascribed lower brain AChE activity in foragers as compared to in-hive honeybees to facilitated learning capabilities.79 In general, the difference in AChE and GST activities between summer and winter honeybees could be attributed to their different physiology. In a study by Badiou-Beneteau et al., all honeybee biochemical biomarkers (GST, alkaline phosphatase, metallothioneins, and membrane AChE) were subjected to seasonal variations.21 Harris and Woodring observed significant seasonal differences in levels of all three biogenic amines, octopamine, dopamine and serotonin, in the brains of worker honeybees.81 Crailsheim also reported seasonal variation of protein content in honeybees, which was dependent on several factors, such as age, current assignment in the hive, and colony nutrition, among others.82,83 The seasonal variation in protein content was evident also from our study as we measured lower protein content in the head, thorax and haemolymph of winter honeybees compared to the summer honeybees. The lower protein content in the heads could be explained by the hypertrophied hypopharyngeal glands of overwintering worker honeybees,84 whereas the lower protein content in the thorax and haemolymph is probably a reflection of reduced metabolisms during winter, when the turnover of proteins is decreased.82,83 It was previously shown that the rates of protein levels in the haemolymph are in correlation with the rates of protein synthesis.85
In this study, chronic (9 day) exposure to up to 500 mg L−1 nCeO2 had no significant effect on the survival of A. m. carnica. However, nCeO2 induced a number of sublethal effects evidenced at the biochemical level, which suggests that nCeO2 does pose a potential threat to the neuronal system of honeybees and induces other physiological responses as well. As recently reviewed by Malev et al., the sublethal effects of nCeO2 on other terrestrial organisms, such as nematodes (Caenorhabditis elegans), isopods (Porcellio scaber), and earthworms (Eisenia fetida), have also been reported.69 However, the exact comparison of honeybee toxicity data with that of other terrestrial organisms is not possible due to different exposure concentrations and ways of exposure. In comparison to other NMs, nCeO2 induced sublethal effects to honeybees at lower exposure concentrations as in the case of TiO2 NM and carbon black NM tested in a similar setup.52
5. Conclusions
This study reveals for the first time that nCeO2 induces a number of sublethal effects on honeybees A. m. carnica after chronic 9 day exposure. Among enzyme activities tested, the most significant alterations were found in the head and thorax AChE in the DS fraction, which most probably denotes an affected neuronal system. AChE activity in the SS fraction was significantly increased, but it remains to be investigated whether this indicates an alteration of soluble AChE and what the actual physiological meaning is. Increased GST activities most probably demonstrate detoxification due to oxidative damage. These results show that AChE and GST activities are altered in a different manner when evaluated in different body compartments and when comparing winter and summer honeybees. We ascribe most of the observed effects to particulate nCeO2 because a negligible presence of Ce ion species was found in their food. We conclude that nCeO2 released into the environment, especially atmospherically deposited material, is a potential risk to honeybees.
Conflicts of interest
There are no conflicts to declare.
Acknowledgements
This research was funded under the EU FP7 project NanoMile (Engineered nanomaterial mechanisms of interactions with living systems and the environment: a universal framework for safe nanotechnology, grant agreement no. 320451). The work of PhD student Monika Kos was supported by the Slovenian Research Agency (ARRS) within the framework of young researchers. Dr. Gregor Marolt acknowledges support from the ARRS (Grant No. P1-0153). We thank Dr. Slavko Kralj from the Jožef Štefan Institute (Ljubljana, Slovenia) for the characterization of nCeO2 dispersions in sucrose solution with DLS. We thank Dr. Matej Hočevar from the Institute of Metals and Technology (Ljubljana, Slovenia) for the help with SEM–EDX analysis. We thank Saša Kos, MSc in Engineering Geology student, for the photo of the honeybee in the graphical abstract. The authors report no conflicts of interest. The authors alone are responsible for the content and writing of the paper. Mention of trade names of commercial products and companies does not constitute endorsement or recommendation for use.
References
- F. R. Cassee, E. C. van Balen, C. Singh, D. Green, H. Muijser, J. Weinstein and K. Dreher, Exposure, health and ecological effects review of engineered nanoscale cerium and cerium oxide associated with its use as a fuel additive, Crit. Rev. Toxicol., 2011, 41(3), 213–229 CrossRef PubMed.
- J. T. Dahle and Y. Arai, Environmental geochemistry of cerium: applications and toxicology of cerium oxide nanoparticles, Int. J. Environ. Res. Public Health, 2015, 12(2), 1253–1278 CrossRef PubMed.
- F. Piccinno, F. Gottschalk, S. Seeger and B. Nowack, Industrial production quantities and uses of ten engineered nanomaterials in Europe and the world, J. Nanopart. Res., 2012, 14(9), 1109, DOI:10.1007/s11051-012-1109-9.
- D. Andreescu, G. Bulbul, R. E. Özel, A. Hayat, N. Sardesai and S. Andreescu, Applications and implications of nanoceria reactivity: measurement tools and environmental impact, Environ. Sci.: Nano, 2014, 1(5), 445–458 RSC.
- B. Park, K. Donaldson, R. Duffin, L. Tran, F. Kelly, I. Mudway, J.-P. Morin, R. Guest, P. Jenkinson, Z. Samaras, M. Giannouli, H. Kouridis and P. Martin, Hazard and risk assessment of a nanoparticulate cerium oxide-based diesel fuel additive - a case study, Inhalation Toxicol., 2008, 20(6), 547–566 CrossRef CAS PubMed.
- F. Gottschalk, C. Lassen, J. Kjoelholt, F. Christensen and B. Nowack, Modeling flows and concentrations of nine engineered nanomaterials in the Danish environment, Int. J. Environ. Res. Public Health, 2015, 12(5), 5581–5602 CrossRef CAS PubMed.
- W. Franus, M. M. Wiatros-Motyka and M. Wdowin, Coal fly ash as a resource for rare earth elements, Environ. Sci. Pollut. Res., 2015, 22(12), 9464–9474 CrossRef CAS PubMed.
- A. A. Keller and A. Lazareva, Predicted releases of engineered nanomaterials: from global to regional to local, Environ. Sci. Technol. Lett., 2014, 1(1), 65–70 CrossRef CAS.
- Y. Arai and J. Dahle, Redox-ligand complexation controlled chemical fate of ceria nanoparticles in an agricultural soil, J. Agric. Food Chem., 2017 DOI:10.1021/acs.jafc.7b01277.
- X. Pang, D. Li and A. Peng, Application of rare-earth elements in the agriculture of China and its environmental behavior in soil, J. Soils Sediments, 2001, 1(2), 124, DOI:10.1065/espr2001.05.065.
- M. L. López-Moreno, G. De la Rosa, J. Á. HernÁndez-Viezcas, H. Castillo-Michel, C. E. Botez, J. R. Peralta-Videa and J. L. Gardea-Torresdey, Evidence of the differential biotransformation and genotoxicity of ZnO and CeO2 nanoparticles on soybean (Glycine max) plants, Environ. Sci. Technol., 2010, 44, 7315–7320 CrossRef PubMed.
- J. A. Hernandez-Viezcas, H. Castillo-Michel, J. C. Andrews, M. Cotte, C. Rico, J. R. Peralta-Videa, Y. Ge, J. H. Priester, P. A. Holden and J. L. Gardea-Torresdey, In situ synchrotron X-ray fluorescence mapping and speciation of CeO2 and ZnO nanoparticles in soil cultivated soybean (Glycine max), ACS Nano, 2013, 7(2), 1415–1423 CrossRef CAS PubMed.
- J. Hawthorne, R. De la Torre Roche, B. Xing, L. A. Newman, X. Ma, S. Majumdar, J. Gardea-Torresdey and J. C. White, Particle-size dependent accumulation and trophic transfer of cerium oxide through a terrestrial food chain, Environ. Sci. Technol., 2014, 48(22), 13102–13109 CrossRef CAS PubMed.
- S. Majumdar, J. Trujillo-Reyes, J. A. Hernandez-Viezcas, J. C. White, J. R. Peralta-Videa and J. L. Gardea-Torresdey, Cerium biomagnification in a terrestrial food chain: influence of particle size and growth stage, Environ. Sci. Technol., 2016, 50(13), 6782–6792 CrossRef CAS PubMed.
-
HEI - Health Effects Institute, Evaluation of Human Health Risk from Cerium Added to Diesel Fuel, Communication 9, Health Effects Institute, Boston MA, 2001, https://www.healtheffects.org/system/files/Cerium.pdf (Accessed June 16th 2017) Search PubMed.
- V. Mommaerts, K. Jodko, L. C. Thomassen, J. A. Martens, M. Kirsch-Volders and G. Smagghe, Assessment of side-effects by Ludox TMA silica nanoparticles following a dietary exposure on the bumblebee Bombus terrestris, Nanotoxicology, 2012, 6(5), 554–561 CrossRef CAS PubMed.
- O. Samson-Robert, G. Labrie, M. Chagnon and V. Fournier, Neonicotinoid-contaminated puddles of water represent a risk of intoxication for honey bees, PLoS One, 2014, 9(12), e108443, DOI:10.1371/journal.pone.0108443.
-
J. Devillers, in Honey bees: estimating the environmental impact of chemicals, ed. J. Devillers and M. H. Pham-Delègue, Taylor and Francis, London, 2003, ch. 1, pp. 1–12 Search PubMed.
- A. Barron, Death of the bee hive: understanding the failure of an insect society, Curr. Opin. Insect Sci., 2015, 10, 45–50 CrossRef.
- T. Farooqui, A potential link among biogenic amines-based pesticides, learning and memory, and colony collapse disorder: a unique hypothesis, Neurochem. Int., 2013, 62, 122–136 CrossRef CAS PubMed.
- A. Badiou-Beneteau, A. Benneveau, F. Geret, H. Delatte, N. Becker, J. L. Brunet, B. Reynaud, B. Reynaud and L. P. Belzunces, Honeybee biomarkers as promising tools to monitor environmental quality, Environ. Int., 2013, 60, 31–41 CrossRef CAS PubMed.
- P. S. Tourinho, P. L. Waalewijn-Kool, I. Zantkuijl, K. Jurkschat, C. Svendsen, A. M. Soares, S. Loureiro and C. A. M. van Gestel, CeO2 nanoparticles induce no changes in phenanthrene toxicity to the soil organisms Porcellionides pruinosus and Folsomia candida, Ecotoxicol. Environ. Saf., 2015, 113, 201–206 CrossRef CAS PubMed.
- A. Bour, F. Mouchet, S. Cadarsi, J. Silvestre, L. Verneuil, D. Baqué, E. Chauvet, J.-M. Bonzom, C. Pagnout, H. Clivot, I. Fourquaux, M. Tella, M. Auffan, L. Gauthier and E. Pinell, Toxicity of CeO2 nanoparticles on a freshwater experimental trophic chain: A study in environmentally relevant conditions through the use of mesocosms, Nanotoxicology, 2016, 10(2), 245–255 CAS.
- A. Bour, F. Mouchet, S. Cadarsi, J. Silvestre, E. Chauvet, J. M. Bonzom, C. Pagnout, H. Clivot, L. Gauthier and E. Pinelli, Impact of CeO2 nanoparticles on the functions of freshwater ecosystems: a microcosm study, Environ. Sci.: Nano, 2016, 3(4), 830–838 RSC.
- M. Alaraby, A. Hernández, B. Annangi, E. Demir, J. Bach, L. Rubio, A. Creus and R. Marcos, Antioxidant and antigenotoxic properties of CeO2 NPs and cerium sulphate: Studies with Drosophila melanogaster as a promising in vivo model, Nanotoxicology, 2015, 9(6), 749–759 CrossRef CAS PubMed.
- Y. H. Kim and S. H. Lee, Which acetylcholinesterase functions as the main catalytic enzyme in the Class Insecta?, Insect Biochem. Mol. Biol., 2013, 43, 47–53 CrossRef CAS PubMed.
- L. P. Belzunces, J. Lenoir-Rousseaux and M. Bounias, Properties of acetylcholinesterase from Apis mellifera heads, Insect Biochem., 1988, 18, 811–819 CrossRef CAS.
- L. P. Belzunces, J. P. Toutant and M. Bounias, Acetylcholinesterase from Apis mellifera head, evidence for amphiphilic and hydrophilic forms characterized by Triton X-114 phase separation, Biochem. J., 1988, 255, 463–470 CrossRef CAS PubMed.
-
S. H. Thany, H. Tricoire-Leignel and B. Lapied, in Insect Nicotinic Acetylcholine Receptors, ed. S. H. Thany, Springer, New York, 2010, ch. 1, pp. 1–10 Search PubMed.
- Y. H. Kim, D. J. Cha, J. W. Jung, H. W. Kwon and S. H. Lee, Molecular and kinetic properties of two acetylcholinesterases from the Western honey bee, Apis mellifera, PLoS One, 2012, 7, e48838, DOI:10.1371/journal.pone.0048838.
- L. Cariello, G. Romano and L. Nelson, Acetylcholinesterase in sea urchin spermatozoa, Gamete Res., 1986, 14, 323–332 CrossRef CAS.
- C. Angelini, B. Baccetti, P. Piomboni, S. Trombino, M. G. Aluigi, S. Stringara, L. Gallus and C. Falugi, Acetylcholine synthesis and possible functions during sea urchin development, Eur. J. Histochem., 2004, 48(3), 235 CAS.
- Y. Lu, Y. Park, X. Gao, X. Zhang, J. Yao, Y. P. Pang, H. Jiang and K. Y. Zhu, Cholinergic and non-cholinergic functions of two acetylcholinesterase genes revealed by gene-silencing in Tribolium castaneum, Sci. Rep., 2012, 2, 288, DOI:10.1038/srep00288.
- P. M. Lenique and J. P. Feral, A mechanism of action of neurotransmitters on the regeneration of the planarian worm Dugesia tigrina, Role of acetylcholine as a negative feed-back, Acta Zool., 1976, 57, 1–5 CrossRef.
- S. M. Fossati, S. Candiani, M. T. Nödl, L. Maragliano, M. Pennuto, P. Domingues, F. Benfenati, M. Pestarino and L. Zullo, Identification and expression of acetylcholinesterase in Octopus vulgaris arm development and regeneration: a conserved role for AChE?, Mol. Neurobiol., 2015, 52(1), 45–56 CrossRef CAS PubMed.
- Y. H. Kim, J. H. Kim, K. Kim and S. H. Lee, Expression of acetylcholinesterase 1 is associated with brood rearing status in the honey bee, Apis mellifera, Sci. Rep., 2017, 7(39864), 1–8 Search PubMed.
- K. K. Lehtonen, D. Schiedek, A. Köhler, T. Lang, P. J. Vuorinen, L. Förlin, J. Baršienė, J. Pempkowiak and J. Gercken, The BEEP project in the Baltic Sea: overview of results and outline for a regional biological effects monitoring strategy, Mar. Pollut. Bull., 2006, 53(8), 523–537 CrossRef CAS PubMed.
- J. S. Kang, D. W. Lee, Y. H. Koh and S. H. Lee, A soluble acetylcholinesterase provides chemical defense against xenobiotics in the pinewood nematode, PLoS One, 2011, 6, e19063, DOI:10.1371/journal.pone.0019063.
- Y. H. Kim, D. H. Kwon, H. M. Ahn, Y. H. Koh and S. H. Lee, Induction of soluble AChE expression via alternative splicing by chemical stress in Drosophila melanogaster, Insect Biochem. Mol. Biol., 2014, 48, 75–82 CrossRef CAS PubMed.
- A. Badiou, M. Meled and L. P. Belzunces, Honeybee Apis mellifera acetylcholinesterase-A biomarker to detect deltamethrin exposure, Ecotoxicol. Environ. Saf., 2008, 69, 246–253 CrossRef CAS PubMed.
- A. Das, M. Dikshit and C. Nath, Profile of acetylcholinesterase in brain areas of male and female rats of adult and old age, Life Sci., 2001, 68(13), 1545–1555 CrossRef CAS PubMed.
- R. Van der Oost, J. Beyer and N. P. E. Vermeulen, Fish bioaccumulation and biomarkers in environmental risk assessment: a review, Environ. Toxicol. Pharmacol., 2003, 13(2), 57–149 CrossRef CAS PubMed.
- Q. Diao, K. Yuan, P. Liang and X. Gao, Tissue distribution and properties of glutathione S-transferases in Apis ceranacerana Fabricius and Apis mellifera ligustica Spinola, J. Apic. Res., 2006, 45, 145–152 CrossRef CAS.
-
B. Halliwell and J. M. C. Gutteridge, Free Radicals in Biology and Medicine, Oxford University Press, New York, 2007 Search PubMed.
- I. Cunha, E. Mangas-Ramirez and L. Guilhermino, Effects of copper and cadmium on cholinesterase and glutathione S-transferase activities of two marine gastropods (Monodonta lineata and Nucella lapillus), Comp. Biochem. Physiol., Part C: Toxicol. Pharmacol., 2007, 145(4), 648–657 CrossRef CAS PubMed.
- A. Jemec, T. Tišler, D. Drobne, K. Sepčić, P. Jamnik and M. Roš, Biochemical biomarkers in chronically metal-stressed daphnids, Comp. Biochem. Physiol., Part C: Toxicol. Pharmacol., 2008, 147(1), 61–68 CrossRef PubMed.
- T. Milivojević, G. Glavan, J. Božič, K. Sepčić, T. Mesarič and D. Drobne, Neurotoxic potential of ingested ZnO nanomaterials on bees, Chemosphere, 2015, 120, 547–554 CrossRef PubMed.
- C. Claudianos, H. Ranson, R. M. Johnson, S. Biswas, M. A. Schuler, M. R. Berenbaum, R. Feyereisen and J. G. Oakeshott, A deficit of detoxification enzymes: pesticide sensitivity and environmental response in the honeybee, Insect Mol. Biol., 2006, 15(5), 615–636 CrossRef CAS PubMed.
- M. R. Berenbaum and R. M. Johnson, Xenobiotic detoxification pathways in honey bees, Curr. Opin. Insect Sci., 2015, 10, 51–58, DOI:10.1016/j.cois.2015.03.005.
- S. M. Carvalho, L. P. Belzunces, G. A. Carvalho, J. L. Brunet and A. Badiou-Beneteau, Enzymatic biomarkers as tools to assess environmental quality: A case study of exposure of the honey bee Apis mellifera to insecticides, Environ. Toxicol. Chem., 2013, 32(9), 2117–2124 CrossRef CAS PubMed.
- G. Glavan, T. Milivojević, J. Božič, K. Sepčić and D. Drobne, Feeding preference and sub-chronic effects of ZnO nanomaterials in honey bees (Apis mellifera carnica), Arch. Environ. Contam. Toxicol., 2017, 72, 471–480 CrossRef CAS PubMed.
- A. Jemec, T. Milivojević, D. Drobne, K. Sepčić, J. Božič and G. Glavan, No chronic effects on biochemical biomarkers, feeding and survival of carnolian honeybees (Apis mellifera carnica) after exposure to nanosized carbon black and titanium dioxide, Acta Biol. Slov., 2016, 59(1), 45–55 Search PubMed.
- W. K. Boyes, R. Chen, C. Chen and R. A. Yokel, The neurotoxic potential of engineered nanomaterials, Neurotoxicology, 2012, 33(4), 902–910 CrossRef PubMed.
-
G. Glavan, M. Kos, J. Božič, D. Drobne and A. Jemec, Differential effects of organophosphate diazinon on membrane and soluble acetylcholinesterase in honeybee head and thorax, in The 7th European Conference of Apidology, ed. D. S. Dezmirean, Academic Pres, Cluj-Napoca, 2016, p. 141 Search PubMed.
-
OECD, Proposal for a new guideline for the testing of chemicals. Honey bee Apis mellifera L. chronic oral toxicity test, OECD Publishing, Paris, 2016 Search PubMed.
- P. Medrzycki, H. Giffardi, P. Aupinel, L. P. Belzunces, M.-P. Chauzat, C. Claßen, M. E. Colin, T. Dupont, V. Girolami, R. Johnson, Y. Leconte, J. Lückmann, M. Marzaro, J. Pistorius, C. Porrini, A. Schur, F. Sgolastra, N. Simon Delso, J. J. F. Van Der Steen, K. Wallner, C. Alaux, D. G. Biron, N. Blot, G. Bogo, J. L. Brunet, F. Delbac, M. Diogon, H. El Alaoui, B. Provost, S. Tosi and C. Vidau, Standard methods for toxicology research in Apis mellifera, J. Apic. Res., 2013, 52(4), 1–60 CrossRef.
- G. L. Ellman, K. D. Courtney, V. Andres and R. M. Featherstone, A new and rapid colorimetric determination of acetylcholinesterase activity, Biochem. Pharmacol., 1961, 7(2), 88–95 CrossRef CAS PubMed.
- W. H. Habig, M. J. Pabst and W. B. Jakoby, Glutathione S-transferase, the first enzymatic step in mercapturic acid formation, J. Biol. Chem., 1974, 249, 7130–7139 CAS.
-
OECD, Test No. 202: Daphnia sp. acute immobilisation test, OECD Publishing, Paris, 2004, DOI: http://dx.doi.org/10.1787/9789264069947-en Search PubMed.
- O. M. Bondarenko, M. Heinlaan, M. Sihtmäe, A. Ivask, I. Kurvet, E. Joonas, A. Jemec, M. Mannerström, T. Heinonen, R. Rekulapelly, S. Singh, J. Zou, I. Pyykkö, D. Drobne and A. Kahru, Multilaboratory
evaluation of 15 bioassays for (eco)toxicity screening and hazard ranking of engineered nanomaterials: FP7 project NANOVALID, Nanotoxicology, 2016, 10(9), 1229–1242 CrossRef CAS PubMed.
- T. J. Brunner, P. Wick, P. Manser, P. Spohn, R. N. Grass, L. K. Limbach, A. Bruinink and W. J. Stark, In vitro cytotoxicity of oxide nanoparticles: comparison to asbestos, silica, and the effect of particle solubility, Environ. Sci. Technol., 2006, 40(14), 4374–4381 CrossRef CAS PubMed.
- B. Collin, M. Auffan, A. C. Johnson, I. Kaur, A. A. Keller, A. Lazareva, J. R. Lead, X. Ma, R. C. Merrifield, C. Svendsen, J. C. White and J. M. Unrine, Environmental release, fate and ecotoxicological effects of manufactured ceria nanomaterials, Environ. Sci.: Nano, 2014, 1(6), 533–548 RSC.
- K. V. Vimalnath, M. K. Das, M. Venkatesh and N. Ramamoorthy, Prospects and problems in the production of 143Pr for radionuclide therapy applications, Radiochim. Acta, 2005, 93(7), 419–426 CrossRef CAS.
- W. R. Terra and C. Ferreira, Insect digestive enzymes: properties, compartmentalization and function, Comp. Biochem. Physiol., 1994, 109(1), 1–62 CrossRef.
-
R. F. Chapman, The insects. Structure and function, Cambridge University Press, United Kingdom, 1998 Search PubMed.
-
V. H. Resh and R. T. Carde, Encyclopedia of Insects, Elsevier Science, USA, 2003 Search PubMed.
- B. B. Manshian, S. Munck, P. Agostinis, U. Himmelreich and S. J. Soenen, High content analysis at single cell level identifies different cellular responses dependent on nanomaterial concentrations, Sci. Rep., 2015, 5, 13890, DOI:10.1038/srep13890.
- N. Buchon, N. A. Broderick and B. Lemaitre, Gut homeostasis in a microbial world: insights from Drosophila melanogaster, Nat. Rev. Microbiol., 2013, 11, 615–626 CrossRef CAS PubMed.
- O. Malev, P. Trebše, M. Piecha, S. Novak, B. Budič, M. D. Dramićanin and D. Drobne, Effects of CeO2 nanoparticles on terrestrial isopod Porcellio scaber: comparison of CeO2 biological potential with other nanoparticles, Arch. Environ. Contam. Toxicol., 2017, 72(2), 303–311 CrossRef CAS PubMed.
- S. M. Hirst, A. Karakoti, S. Singh, W. Self, R. Tyler, S. Seal and C. M. Reilly, Bio-distribution and in vivo antioxidant effects of cerium oxide nanoparticles in mice, Environ. Toxicol., 2013, 28(2), 107–118 CrossRef CAS PubMed.
- S. Das, J. M. Dowding, K. E. Klump, J. F. McGinnis, W. Self and S. Seal, Cerium oxide nanoparticles: applications and prospects in nanomedicine, Nanomedicine, 2013, 8(9), 1483–1508 CrossRef CAS PubMed.
- C. Xu and X. Qu, Cerium oxide nanoparticle: a remarkably versatile rare earth nanomaterial for biological applications, NPG Asia Mater., 2014, 6(3), e90, DOI:10.1038/am.2013.88.
- A. Jemec, D. Drobne, T. Tišler, P. Trebše, M. Roš and K. Sepčić, The applicability of acetylcholinesterase and glutathione S-transferase in Daphnia magna toxicity test, Comp. Biochem. Physiol., Part C: Toxicol. Pharmacol., 2007, 144, 303–309 CrossRef PubMed.
- T. R. Fukuto, Mechanism of action of organophosphorus and carbamate insecticides, Environ. Health Perspect., 1990, 87, 245–254 CrossRef CAS PubMed.
- S. Kumar, Biphasic effect of aluminium on cholinergic enzyme of rat brain, Neurosci. Lett., 1998, 248, 121–123 CrossRef CAS PubMed.
- M. Boily, B. Sarrasin, C. DeBlois, P. Aras and M. Chagnon, Acetylcholinesterase in honeybees (Apis mellifera) exposed to neonicotinoids, atrazine and glyphosate: laboratory and field experiments, Environ. Sci. Pollut. Res., 2013, 20(8), 5603–5614 CrossRef CAS PubMed.
- S. H. Lee, Y. H. Kim, D. H. Kwon, D. J. Cha and J. H. Kim, Mutation and duplication of arthropod acetylcholinesterase: implications for pesticide resistance and tolerance, Pestic. Biochem. Physiol., 2015, 120, 118–124 CrossRef CAS PubMed.
- R. Rajendran, E. Borghi, M. Falleni, F. Perdoni, D. Tosi, D. F. Lappin, L. O'Donnell, D. Greetham, G. Ramage and C. Nile, Acetylcholine protects against Candida albicans infection by inhibiting biofilm formation and promoting hemocyte function in a Galleria mellonella infection model, Eukaryotic Cell, 2015, 14(8), 834–844 CrossRef CAS PubMed.
- M. Shapira, C. K. Thompson, H. Soreq and G. E. Robinson, Changes in neuronal acetylcholinesterase gene expression and division of labor in honey bee colonies, J. Mol. Neurosci., 2001, 17(1), 1, DOI:10.1385/JMN:17:1:1.
- A. Polyzou, J. F. Debras and L. P. Belzunces, Changes in acetylcholinesterase during pupal development of Apis mellifera queen, Arch. Insect Biochem. Physiol., 1997, 36(2), 69–84 CrossRef CAS.
- J. W. Harris and J. Woodring, Effects of stress, age, season, and source colony on levels of octopamine, dopamine and serotonin in the honey bee (Apis mellifera L.) brain, J. Insect Physiol., 1992, 38(1), 29–35 CrossRef CAS.
- K. Crailsheim, Dependence of protein metabolism on age and season in the honeybee (Apis mellifica carnica Pollm.), J. Insect Physiol., 1986, 32(7), 629–634 CrossRef CAS.
- K. Crailsheim, The protein balance of the honey bee worker, Apidologie, 1990, 21(5), 417–429 CrossRef CAS.
- E. V. M. Brouwers, Activation of the hypopharyngeal glands of honeybees in winter, J. Apic. Res., 1983, 22, 137–141 CrossRef CAS.
-
N. N. Sinizki and I. W. Lewtschenko, Der Gehalt an Eiweißszlig und freien Aminosäuren in der Hämolymphe der Arbeitsindividuen der Honigbiene, 23rd International Beekeeping Congress Moscow, Apimondia Publishing House, Bucharest, 1971, pp. 361–364 Search PubMed.
Footnote |
† Electronic supplementary information (ESI) available. See DOI: 10.1039/c7en00596b |
|
This journal is © The Royal Society of Chemistry 2017 |