Retracted Article: Mechanistic insights into the decontamination of Th(IV) on graphene oxide-based composites by EXAFS and modeling techniques†
Received
8th October 2016
, Accepted 9th November 2016
First published on 10th November 2016
Abstract
The sorption mechanism of Th(IV) on amidoxime/graphene oxide composites was investigated by extended X-ray absorption fine structure (EXAFS) spectra and surface complexation modeling. It was found that amidoxime/graphene oxide composites possessed a variety of oxygen- and nitrogen-containing functional groups such as hydroxyl, carboxyl, carbonyl, amine and imine groups. The sorption kinetics and sorption isotherms of Th(IV) on amidoxime/graphene oxide composites can be satisfactorily fitted by the pseudo-second-order kinetic model and the Langmuir model, respectively. The maximum sorption capacity of Th(IV) on amidoxime/graphene oxide composites calculated from the Langmuir model at pH 2.0 and 293 K was 123.46 mg g−1. According to EXAFS analysis, the presence of a Th–C shell (R = 2.97 Å, CN = 4.9) indicated that inner-sphere surface complexation dominated the sorption of Th(IV) on amidoxime/graphene oxide composites at pH 2.0, whereas the occurrence of a Th–Th shell revealed that a surface precipitate dominated the sorption of Th(IV) on amidoxime/graphene oxide composites at pH 5.0. The pH-dependent sorption behaviors of Th(IV) on amidoxime/graphene oxide composites can be satisfactorily simulated by double layer modeling based on two inner-sphere surface complexes and a surface precipitate. These observations revealed that the amidoxime/graphene oxide composites can be regarded as a promising adsorbent to remove tetravalent radionuclides from aqueous solutions in environmental cleanup.
Environmental significance
This study contributes further to our understanding of the interaction mechanism between radionuclides and graphene oxide-based nanoparticles, which is significant and important for the potential application of nanoparticles in environmental remediation. These findings indicated that amidoxime/graphene oxide composites can be regarded as a highly effective adsorbent for the decontamination of radionuclides from aqueous solutions in environmental cleanup.
|
Introduction
The pollution of radionuclides from the mining, milling, and processing of materials for the nuclear power industry is a public environmental concern.1 These radionuclides could present a variety of potential threats for human health due to their radioactivity, toxicity and bioaccumulation. Therefore, the removal of radionuclides from aqueous solutions by a variety of adsorbents has been extensively investigated. Candidate adsorbents under study are, for instance, clay minerals,2–4 metal (hydr)oxides5–11 and nanoparticles.12–18 In hitherto studies, the effect of water chemistry (e.g., reaction time, pH, ionic strength and concentration) on the sorption of radionuclides at the water–solid interface had been elucidated by batch techniques. Chen et al. found that the maximum sorption capacity of Th(IV) on oxidized multi-wall carbon nanotubes at pH 2.0 and 293 K was 13.26 mg g−1.19 Such limited sorption capacities of these adsorbents prevented their wide use in practical fields, especially under low pH conditions.20
Owing to their unique excellent dispersive properties, various oxygenated functional groups and large specific surface area, graphene oxides (GOs) have been recently investigated as highly efficient adsorbents to remove various contaminants such as organic21–24 and inorganic pollutants.25–30 The high sorption capacity of GOs can be ascribed to the various oxygen-containing functional groups such as the carboxyl (–COOH) groups at the sheet edges and the hydroxyl (–OH)/epoxy groups (–O–) on the basal plane.31 In addition, GOs display excellent dispersity in aqueous solutions due to the presence of abundant hydrophilic oxygenated functional groups. The particle size of GO nanoparticles is too small to easily separate them from the liquid phase by centrifugation.32 Numerous researchers synthesized various GO-based composites such as Fe3O4/GO composites33–35 and Fe0/GO composites.15,36,37 Sun et al. found that the maximum sorption capacity of GO-supported polyaniline composites at pH 3.0 and 298 K was 1.03, 1.65, 1.68 and 1.39 mmol g−1 for U(VI), Eu(III), Sr(II) and Cs(I), respectively.38 Owing to the abundant oxygen- and nitrogen-containing functional groups (i.e., amine, imine, hydroxyl, carboxyl and carbonyl groups), amidoxime/graphene oxide composites have been extensively used to remove a variety of radionuclides such as U(VI).39–42 To the authors' knowledge, few investigations regarding the sorption mechanism of Th(IV) on amidoxime/graphene oxide composites by spectroscopic and modeling techniques are currently available in the literature.
Extended X-ray absorption fine structure (EXAFS) spectroscopy1,4,8,15,26,31,43,44 and surface complexation modeling (SCM)1,2,4–6,26,45–48 have been extensively applied for investigating the interaction mechanism of radionuclides at the water–mineral interface in recent years. Based on EXAFS spectra, Seco et al. demonstrated that Th(IV) ion sorbed onto the magnetite surface as bidentate-corner sharing arrangements to [FeO6] octahedral and [FeO4] tetrahedral.10 Xu et al. used surface complexation modeling to satisfactorily simulate the sorption of Th(IV) on GO with SOHTh4+ and SOTh(OH)2+ species.49 Therefore, a thorough investigation of the fate and transport of radionuclides at the water–solid interface by combining EXAFS and SCM is mandatory for the risk assessment of radionuclides in environmental remediation management.
The objectives of this study were (1) to characterize amidoxime/graphene oxide composites by using SEM, electrophoretic light scattering, XPS and FT-IR techniques; (2) to investigate the effect of various environmental factors (e.g., reaction time, pH, ionic strength, concentration and temperature) on the sorption of Th(IV) on amidoxime/graphene oxide composites by batch techniques; (3) to determine the sorption mechanism of Th(IV) on amidoxime/graphene oxide composites by using EXAFS spectroscopy and SCM. The highlight of this study is to distinguish surface precipitation from inner-sphere surface complexation under different pH conditions by EXAFS analysis and surface complexation modeling. These findings were crucial for the potential application of GO-based composites as a highly efficient adsorbent to remove thorium and other tetravalent actinides from aqueous solutions in environmental cleanup.
Experimental details
Synthesis of amidoxime/graphene oxide composites
First of all, graphene oxide was synthesized from flake graphite (<30 microns, Qingdao, China) by using a modified version of Hummer's method.50 Briefly, flake graphite (2.0 g) and NaNO3 (1.5 g) were added to concentrated H2SO4 (100 mL) under ultrasonication and ice-bath conditions; then KMnO4 (9.0 g) was slowly added to the suspension, and the excess MnO4− anions were eliminated by adding 6 mL of H2O2 (30 wt%) solution. The amidoxime dispersion was generated by adding iminodiacetonitrile into NH2OH solution at pH 7.0.42 Briefly, 2.78 g NH2OH·HCl was dissolved in a water/methanol solution (50/50, V/V) and the pH value was adjusted to 7.0 by adding K2CO3 solution. Then, 1.92 g iminodiacetonitrile was added into the solution under stirring at 80 °C for 8 h. The amidoxime/graphene oxide composites were obtained by mixing amidoxime solution and graphene oxide solutions. Typically, 5 mL of 10.0 g L−1 graphene oxide solution was added into 3 mL amidoxime solution under magnetic stirring. Then the mixture was freeze–dried for 4 days, immersed in deionized water and ethanol for 1 day, and then dried in a vacuum oven at 60 °C for 12 h. Th(IV) stock solution at 0.1 mol L−1 was prepared by dissolving ThCl4 (99.9% purity, Sigma-Aldrich) in 0.01 mol L−1 HCl solution. The other chemicals of analytical grade were purchased from Sinopharm Chemical Reagent Co., Ltd.
Characterization
The amidoxime/graphene oxide composites were characterized by using SEM, electrophoretic light scattering, XPS and FT-IR. Samples for SEM were prepared by diluting the amidoxime/graphene oxide solution on a conductive Si wafer. After coating, the sample was examined by using a field emission scanning electron microscope (FEI-JSM 6320F). The zeta potentials under different pH conditions were measured by a Malvern Zetasizer Nano ZS analyzer. The XPS spectra were measured on a thermo ESCALAB 250 electron spectrometer with a multidetection analyzer using an Al Kα X-ray source (1486.6 eV) at 10 kV and 5 mA under 10−8 Pa residual pressure. The FT-IR spectra of the samples were recorded on pressed KBr pellets (Aldrich, 99%, analytical reagent) by using a Nicolet 8700 FT-IR spectrometer at room temperature.
Batch sorption
The triplicate batch sorption of Th(IV) on amidoxime/graphene oxide composites was investigated under ambient conditions. The effect of pH on Th(IV) sorption onto 0.5 g L−1 amidoxime/graphene oxide composites was studied at T = 293 K by the batch technique, whereas the sorption isotherms were carried out at pH 2.0 and T = 293 K at different Th(IV) concentrations. The Th(IV) solution was added dropwise into the amidoxime/graphene oxide composite suspension to avoid the formation of surface precipitate such as Th(OH)4 precipitate. The blank sorption of Th(IV) without amidoxime/graphene oxide composites was also determined under the same experimental conditions. Briefly, the bulk suspensions of amidoxime/graphene oxide composites and NaCl were pre-equilibrated for 24 h, then Th(IV) solution was gradually added to the aforementioned suspension and the suspensions were reacted for 48 h. Then the suspensions were separated by centrifugation at 40
000g for 30 min. The concentrations of Th(IV) in the aqueous solutions were measured by inductively coupled plasma-mass spectroscopy (ICP-MS, Agilent Technologies). The sorption amount (Qe, mg g−1) for Th(IV) by amidoxime/graphene oxide composites at equilibrium was obtained by a mass balance of Th(IV) before and after sorption.
Column experiments
In order to demonstrate the sorption performance of amidoxime/graphene oxide composites under more complex environmental conditions, the sorption capacity of amidoxime/graphene oxide composites for actual Th(IV)-containing wastewater was determined by performing column experiments in triplicate. Th(IV)-containing wastewater was from a certain spent fuel treatment plant. The constituents included the following: 8.5 mg L−1 U(VI), 0.461 mg L−1 Th(IV), 4.64 mol L−1 HF and 7.05 mol L−1 H2SO4. Briefly, 0.5 g of amidoxime/graphene oxide composites was wetted with 10 mL of deionized water. The mixture was transferred into a 1.0 cm × 5.0 cm polycarbonate column. Then, 30 mL of deionized water was passed through the column. The breakthrough experiment was conducted by passing the aforementioned Th(IV) solution at ambient temperature at a flow rate of 1.5 ml min−1. A small amount of effluents was collected and analyzed by inductively coupled plasma-mass spectroscopy (ICP-MS, Agilent Technologies).
Preparation and analysis of XPS and EXAFS
Samples for XPS and EXAFS analysis of Th(IV)-bearing amidoxime/graphene oxide composites were prepared as follows: all experiments were conducted in a glove box (<1 ppm CO2) at room temperature. Briefly, 0.125 g amidoxime/graphene oxide composites was added into 250 mL flask bottles and then Milli-Q water (boiled then bubbled with Ar) of 0.01 mol L−1 ionic strength was added to achieve a 0.5 g L−1 suspension and pre-equilibrated for 24 h. The ThCl4 solutions were added dropwise under vigorous stirring in order to avoid the formation of Th(OH)4 precipitate, and the solution was adjusted to pH 2.0 or 5.0 by using negligible volumes of 0.01–1.0 mol L−1 HClO4 or NaOH solution. The samples were then gently agitated on a shaker (125 rpm min−1) for 2 days. The solid phase was separated from the liquid phase by centrifuging the samples at 40
000g for 30 min. The wet pastes of Th(IV)-bearing amidoxime/graphene oxide composites were mounted in Teflon sample holders with Kapton tape. Thorium LIII-edge EXAFS spectra were measured at Shanghai Synchrotron Radiation Facility (SSRF, Shanghai, China) using a double-crystal Si(111) monochromator with a 64-element solid-state Ge detector. The spectra of crystalline Th(CO3)2(s) and the sorption samples were measured in transmission and fluorescence mode, respectively.
The raw EXAFS spectra were recorded using the correction of energy and subtraction of the background. The k3-weighted EXAFS data were analyzed using Athena and Artemis interfaces to IFFEFIT 7.0 software.51,52 The fitting of paths (i.e., Th–Oax, Th–Oeq, Th–C, Th–Th shell) was derived from the crystal structures of Th(CO3)2·6H2O.53
Surface complexation modeling
Surface complexation modeling was used to simulate the pH-dependent sorption of Th(IV) on amidoxime/graphene oxide composites with the aid of Visual MINTEQ 3.0.54 The two surface acidity constants (K+, K−) are given by the following protonation and deprotonation reactions:where SOH refers to the amphoteric hydroxide functional groups of amidoxime/graphene oxide composites. The values of log
K+ and log
K− can be obtained by fitting the potentiometric data of amidoxime/graphene oxide composites in the presence of 0.01 mol L−1 NaCl solutions.
The surface complexation reactions provide a mathematical description of the charge–potential relationship near the solid surface and each requires a different number of fit parameters. Two surface complexation reactions and a surface precipitate were described by eqn (3)–(5):
| SOH + Th4+ = SOTh3+ + H+ | (3) |
| 2SOH + Th4+ + 3H2O = (SO)2Th(OH)3− + 5H+ | (4) |
| Th4+ + 4H2O = Th(OH)4(s) + 4H+ | (5) |
The adjustable chemical coordination parameters were optimized by fitting the experimental data of Th(IV) on amidoxime/graphene oxide composites under different pH conditions in the presence of 0.01 mol L−1 NaCl solution. Using these adjustable parameters, the concentration of total reactive sites (mol g−1) can be obtained by fitting the potentiometric data. The quality of the fitting results is evaluated using the standard deviation.
Results and discussions
Characterization
As shown by the SEM image in Fig. 1A, amidoxime/graphene oxide composites consist of multi-layered aggregates with a lateral size ranging from several sub-microns to tens of nanometers. Fig. 1B shows the change of zeta potentials of amidoxime/graphene oxide composites under different pH conditions. One can see that the positive and negative charges of amidoxime/graphene oxide composites are observed at pH <2.0 and pH >2.0, respectively. The zeta potentials of amidoxime/graphene oxide composites are much more positive (>−6 mV) than those of pristine graphene oxide (usually <−30 mV), which is due to the interaction of alkaline groups (amine) of amidoxime and acidic groups (carboxyl) of graphene oxides.43 As illustrated by XPS in Fig. 1C, the high resolution C 1s XPS spectrum can be deconvoluted into three bands at 284.4, 286.5 and 288.5 eV, corresponding to C–C, C–O/C–N and CO(O) groups, respectively.38 The results of XPS analysis indicate that amidoxime/graphene oxide composites present a variety of nitrogen- and oxygen-containing functional groups such as amine, carboxyl and hydroxyl groups. As shown by the FT-IR spectrum in Fig. 1D, amidoxime/graphene oxide composites display characteristic bands at 823 and 987 cm−1, which are assigned to C–H out-of-plane sites on the 1,2-ring and C–H in-plane sites on the 1,2,4-ring, respectively.55–59 The bands at 1289, 1600, 1720 and 3200 cm−1 can be attributed to the sp2 C
C bond, C
O stretching vibration, C(O)O and OH stretching vibration, respectively.26,60–63 It should be noted that the other peaks at 1145, 1490 and 1565 cm−1 are assigned to the stretching vibration of the N–Q–N–Q group in the quinonoid (Q) ring, the C
C group in the benzenoid ring and the N
Q
N in the quinonoid ring, respectively.64 The stretching vibration of N–Q–N–Q in the quinonoid ring shows that the amidoxime is successfully bound onto the graphene oxide surface.59 As shown in Table 1, the compositions of amidoxime/graphene oxide composites derived from XPS analysis are C (∼69%), O (27%) and N (3.22%). The results of characterization indicate that amidoxime/graphene oxide composites present a variety of nitrogen- and oxygen-containing functional groups such as amine, carboxyl and hydroxyl groups.
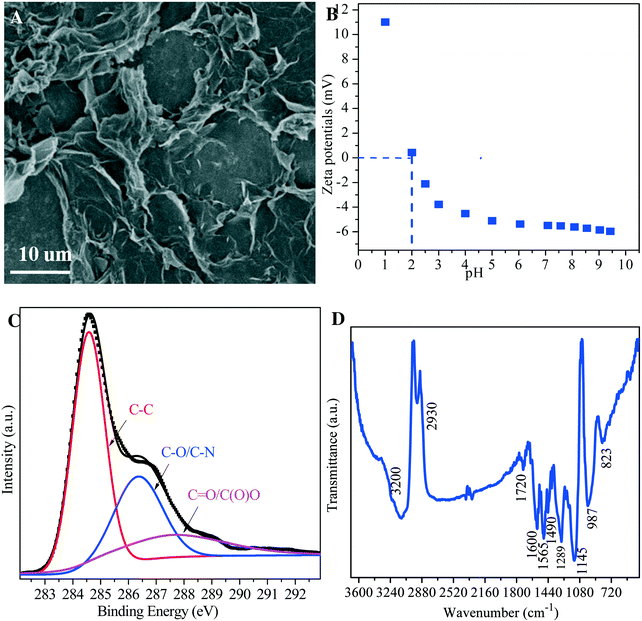 |
| Fig. 1 Characterization of amidoxime/graphene oxide composites. (A) SEM; (B) zeta-potential; (C) XPS; (D) FTIR. | |
Table 1 Selected parameters for amidoxime/graphene oxide composites
Compositiona (%) |
C (69.38 ± 0.10%d) |
N (3.22 ± 0.19%) |
O (27.37 ± 0.12%) |
Data from XPS analysis.
Data from acid–base titration.
Concentration of surface active sites, data from acid–base titration.
Standard deviation.
|
S
BET (m2 g−1) |
|
140.8 |
|
pHPZCb |
|
4.6 ± 0.32d |
|
[SOH] (mol g−1)c |
|
0.068 ± 0.26d |
|
Sorption kinetics
Fig. 2A shows the sorption kinetics of Th(IV) on amidoxime/graphene oxide composites at C0 = 5.0, 10.0 and 20.0 mg L−1. For C0 = 5.0 and 10 mg L−1, approximately 100% of Th(IV) is removed by amidoxime/graphene oxide composites at the reaction time of 1 h. The high sorption of Th(IV) on amidoxime/graphene oxide composites at C0 = 20.0 mg L−1 is also observed, which is attributed to the formation of surface Th(OH)4 precipitate. The sorption rate of Th(IV) on amidoxime/graphene oxide composites at C0 = 5.0 mg L−1 is significantly higher than that of Th(IV) sorption under high concentration conditions (C0 = 20.0 mg L−1) indicating that amidoxime/graphene oxide composites display a fast sorption rate for Th(IV) (inset in Fig. 2A). The data of sorption kinetics of Th(IV) on amidoxime/graphene oxide composites are fitted by pseudo-first-order and pseudo-second-order kinetic models. More details on the description of pseudo-first-order and pseudo-second-order kinetic models are provided in the ESI.† As shown in Fig. S1 and Table S1,† the sorption kinetics of Th(IV) on amidoxime/graphene oxide composites can be fitted by the pseudo-second-order kinetic model very well (R2 = 1) compared to the pseudo-first-order kinetic model (R2 < 0.65).
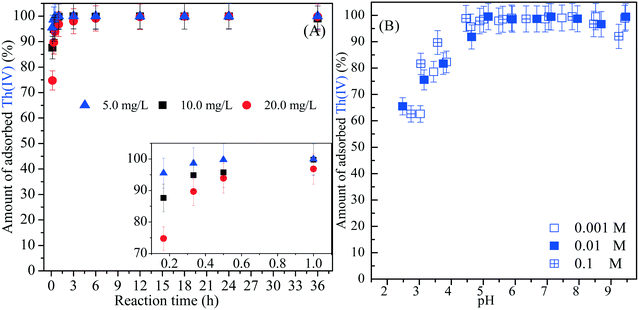 |
| Fig. 2 (A) The sorption kinetics of Th(IV) on amidoxime/graphene oxide composites at C0 = 5.0, 10.0 and 20.0 mg L−1, pH = 2.0, I = 0.01 mol L−1 NaCl, m/v = 0.5 g L−1, T = 293 K. (B) The effect of pH and ionic strength on Th(IV) sorption on amidoxime/graphene oxide composites, C0 = 10.0 mg L−1, m/v = 0.5 g L−1, T = 293 K. | |
Effect of pH and ionic strength
Fig. 2B shows the effect of pH on Th(IV) sorption onto amidoxime/graphene oxide composites at 0.01 mol L−1 NaCl solution. As shown in Fig. 2B, Th(IV) sorption on amidoxime/graphene oxide composites increases significantly with the increase in pH from 2.0 to 5.0, and then the sorption of Th(IV) remains a plateau at pH 5.0–9.0. However, Sun et al. found that the significant decrease of U(VI) sorption on polyamine/graphene oxides at pH >7.0 was due to the occurrence of U(VI)–carbonato complexes.38 Fig. S2† shows the distribution of Th(IV) species in aqueous solutions under different pH conditions calculated from corresponding equilibrium constants (Table S2†). One can see that the main Th(IV) species at pH <3.0 are the Th4+ species, and multiple Th(IV) species (e.g., ThOH3+, Th(OH)22+, Th(OH)3+ and Th(OH)4(s)) are observed at pH >3.0. As shown in Fig. 1B, a negative charge of amidoxime/graphene oxide composites was observed at pH >2.0. Therefore, the increased sorption of Th(IV) on amidoxime/graphene oxide composites at pH 2.0–4.0 can be attributed to electrostatic attraction between the negative charge of amidoxime/graphene oxide composites and the positive charge of Th(IV) (e.g., Th4+, Th(OH)3+ and Th(OH)22+ species). According to Fig. 2B and S2,† the Th(IV) sorption onto amidoxime/graphene oxide composites at pH >5.0 could be due to the formation of the surface precipitate Th(OH)4(s). Approximately 65% and 99.9% of Th(IV) are removed by amidoxime/graphene oxide composites at pH 2.5 and 5.0, respectively. As shown in Table 1, the concentration of surface active sites calculated from acid–base titration is 0.068 mol g−1. The enhanced sorption of Th(IV) on amidoxime/graphene oxide composites is attributed to the abundant oxygen- and nitrogen-containing functional groups, which can generate the strong inner-sphere complexation with Th(IV).
Sorption isotherms
Fig. 3A shows the sorption isotherms of Th(IV) on amidoxime/graphene oxide composites at different temperatures. One can clearly see that the sorption of Th(IV) on amidoxime/graphene oxide composites significantly increases with increasing temperature, indicating enhanced sorption of Th(IV) at high temperature. The sorption data of Th(IV) on amidoxime/graphene oxide composites are fitted by using the Langmuir and the Freundlich models. More details on the description of Langmuir and Freundlich models are provided in the ESI.† As shown in Table S3,† Th(IV) sorption on amidoxime/graphene oxide composites can be satisfactorily fitted by the Langmuir model with a higher correlation coefficient (R2 >0.995) compared to the Freundlich model (R2 <0.99). The maximum sorption capacity of amidoxime/graphene oxide composites calculated for the Langmuir model is 123.46 mg g−1 for Th(IV) at pH 2.0 and T = 293 K. As shown in Table 2, the maximum sorption capacity of amidoxime/graphene oxide composites is significantly higher than that of natural adsorbents such as γ-Al2O3 (14.94 mg g−1),65 TiO2 (49.89 mg g−1),66 SiO2 (0.232 mg g−1),67 and diatomite (18.91 mg g−1)68 and nanoparticles such as oxidized multi-wall carbon nanotubes (13.26 mg g−1),19 polyamine/zeolite (9.28 mg g−1),69 and graphene oxide (60.09 mg g−1).49 In addition, amidoxime/graphene oxide composites display a high sorption capacity for other radionuclides such as 398.4 mg g−1 for U(VI).42 Compared to graphene oxide, the high sorption capacity of amidoxime/graphene oxide composites for radionuclides is attributed to the abundant nitrogen- and oxygen-containing functional groups. It is demonstrated that the amidoxime/graphene oxide composites can be regarded as a promising adsorbent for the preconcentration and sorption of thorium and other tetravalent actinides from aqueous solutions at low pH in environmental pollution cleanup.
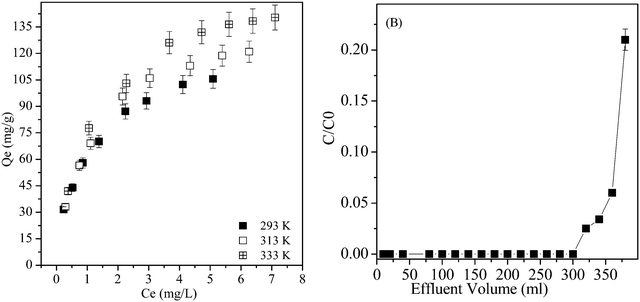 |
| Fig. 3 (A) The sorption isotherms of Th(IV) on amidoxime/graphene oxide composites, pH = 2.0, I = 0.01 mol L−1 NaCl, m/v = 0.5 g L−1. (B) Breakthrough curves for actual Th(IV)-containing wastewater on amidoxime/graphene oxide composites, flow rate = 1.5 mL min−1. | |
Table 2 The maximum sorption capacity of Th(IV) on various adsorbents
Adsorbents |
Conditions |
q
m (mg g−1) |
Ref. |
γ-Al2O3 |
pH 3.5, T = 298 K |
14.94 |
65
|
TiO2 |
pH 3.0, T = 298 K |
49.89 |
66
|
SiO2 |
pH 3.5, T = 298 K |
0.232 |
67
|
Diatomite |
pH 2.5, T = 303 K |
18.91 |
69
|
Oxidized multi-wall carbon nanotubes |
pH 2.0, T = 293 K |
13.36 |
19
|
PAN/zeolite |
pH 4.0, T = 298 K |
9.28 |
69
|
Graphene oxide |
pH 4.0, T = 293 K |
60.09 |
49
|
Amidoxime/graphene oxide composites |
pH 2.0, T = 293 K |
123.46 |
This study |
The thermodynamic parameters (e.g., ΔG0, ΔH0 and ΔS0) of Th(IV) sorption on amidoxime/graphene oxide composites are calculated in the ESI† (Fig. S3). As shown in Table S4,† the negative ΔG0 values (e.g., −27.41 kJ mol−1 at 293 K, −32.86 kJ mol−1 at 333 K) indicate that the sorption of Th(IV) on amidoxime/graphene oxide composites is a spontaneous process. The more negative ΔG0 value at 333 K indicates an energetically more favorable sorption at higher temperature. A positive value of ΔH0 (12.493 kJ mol−1) reveals that the sorption of Th(IV) on amidoxime/graphene oxide composites is an endothermic process. The positive value of ΔS0 (136.06 J mol−1 K−1) indicates the increase of the degree of freedom at the solid–liquid interface during the sorption of Th(IV) on amidoxime/graphene oxide composites. The thermodynamic parameters calculated from temperature dependent sorption isotherms suggest that the sorption of Th(IV) on amidoxime/graphene oxide composites is an endothermic and spontaneous process. The breakthrough capacity of amidoxime/graphene oxide composites for Th(IV) at pH 2.0 is given in Fig. 3B. The maximum loading prior to breakthrough for Th(IV) at pH 2.0 is ca. 300 ml, indicating that mutual separation is feasible and preconcentration is possible from dilute solutions.70 The results of breakthrough studies indicate that amidoxime/graphene oxide composites present a good potential for the enrichment of traces of thorium from large sample volumes.
XPS and EXAFS analysis
The sorption mechanism of Th(IV) on amidoxime/graphene oxide composites is demonstrated by XPS and EXAFS analysis. Fig. 4 shows the XPS spectra of Th(IV) on amidoxime/graphene oxide composites after sorption and desorption (noted as AMI/GO_Th and AMI/GO_Th-HCl, respectively). The occurrence of Th 4f peaks after Th(IV) sorption, while the relative intensities of the N 1s and O 1s peaks are decreased (Fig. 4A), indicate that the high effective sorption of Th(IV) on amidoxime/graphene oxide composites is attributed to these oxygen- and nitrogen-containing functional groups. After desorption, the reduced degree of relative intensity of the N 1s peak is lower than that of the O 1s peak, which reveals that the nitrogen-containing functional groups present a strong chemical affinity to Th(IV) compared to the oxygen-containing functional groups. The evidence is further supported by the high resolution of the N 1s and O 1s peaks before and after desorption. As shown in Fig. 4B and C, the relative intensities of the N 1s and O 1s peaks of amidoxime/graphene oxide composites after sorption are significantly decreased. In addition, the positions of the N 1s and O 1s peaks are shifted to the lower binding energy. It is interesting that the change of N 1s peaks after desorption is significantly less than that of O 1s, suggesting that Th(IV) is not easily desorbed from nitrogen-containing functional groups. These findings are consistent with previous studies.38Fig. 4D shows the high resolution of Th 4f peaks before and after desorption. The relative intensities of Th 4f7/2 (at ∼335 eV) and Th 4f5/2 (at ∼342 eV) after sorption are significantly higher than those of Th(IV) desorption, indicating that more Th(IV) are sorbed on the surface of amidoxime/graphene oxide composites.
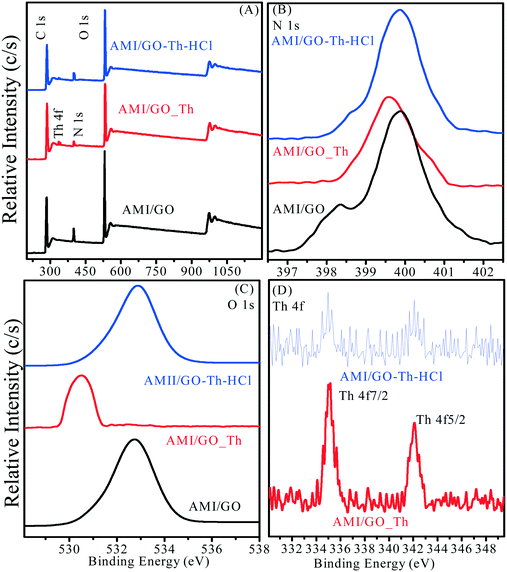 |
| Fig. 4 The XPS spectra of amidoxime/graphene oxide composites before and after Th(IV) sorption and desorption: (A) total scans; (B) N 1s; (C) O 1s and (D) Th 4f. | |
Fig. 5 shows the k2-weighted Th LIII-edge EXAFS spectra and the corresponding Fourier transforms (FT, uncorrected phase shift) of the reference (Th(CO3)2 solid) and sorption samples at pH 2.0 and 5.0. As shown in Fig. 5A, the EXAFS spectra of all sorption samples show a distinct cyclic evolution, whereas a poor signal-to-noise ratio is observed for all samples at κ > 6 Å−1 due to the low Th4+ concentration. The fitted results (dashed lines) and the corresponding parameters are shown in Fig. 5B and Table 3, respectively. As summarized in Table 3, large values of the Debye–Waller factor are observed at high energy shells (such as Th–C and Th–Th shells), which is consistent with a broader peak in the Fourier transform (Fig. 5B). As shown in Fig. 5B, the FT peaks at ∼1.9 and 2.3 Å (no corrected phase) can be satisfactorily fitted by approximately 9 (Th–O1) and 3.5 (Th–O2) oxygen atoms with a bond distance of 2.49 and 3.63 Å, respectively.13 The occurrence of the Th–O2 shell could be attributed to the effect of CO32−. It can be seen that the shorter Th–O distance is observed with increasing pH, indicating that the interaction of Th(IV) with amidoxime/graphene oxide composites shifts from monodentate coordination to bidentate coordination with increasing pH.10 As shown in Table 3, the bond distances of the Th–O2 shell for Th(IV)_pH 2.0 and Th(IV)_pH 5.0 (R ∼2.55 Å) are significantly lower than that of the Th–O2 shell for Th(CO3)2 (R = 3.63 Å). These results indicate that the high effective sorption of Th(IV) on amidoxime/graphene oxide composites is attributed to both oxygen and nitrogen-containing functional groups. For the samples of Th(IV)_pH 2.0 and Th(IV)_pH 5.0, FT peaks at ∼2.7 Å are consistent with the Th–C distance of ∼3.0 Å (Table 3), indicating the formation of inner-sphere surface complexes of Th(IV) and amidoxime/graphene oxide composites at pH 2.0 and 5.0.10 Interestingly, the occurrence of a Th–Th shell for Th(IV)_pH 5.0 reveals the formation of a surface precipitate under high pH conditions.53 The results from the EXAFS spectra show that the primary inner-sphere surface complexation dominates the sorption of Th(IV) on amidoxime/graphene oxide composites over a wide range of pH from 2.0 to 5.0, whereas a surface precipitate is partially observed at pH 5.0.
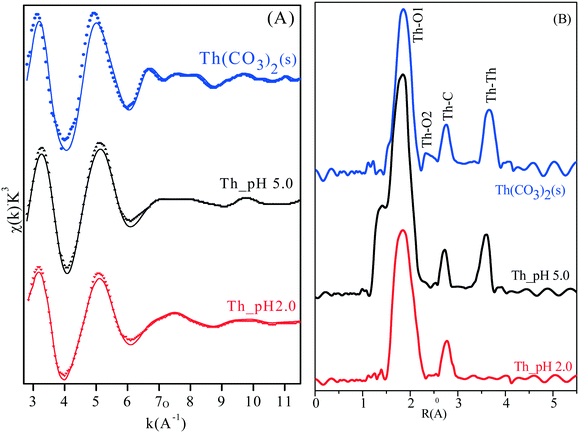 |
| Fig. 5 Th(IV) LIII-edge EXAFS spectra (A) and corresponding Fourier transforms (B) of Th4+ and sorption samples, C0 = 10.0 mg L−1, I = 0.01 mol L−1 NaCl, m/v = 0.5 g L−1, T = 293 K. | |
Table 3 EXAFS structural parameter for the Th(IV) carbonate and Th sorbed on amidoxime/graphene oxide composites at pH 2.0 and 5.0
Sample |
Shell |
R (Å)a |
N
|
σ
2 (Å2)c |
Error in distance of ±0.02 Å.
Errors in coordination numbers ±20%.
Debye–Waller factor.
R ± standard deviation.
|
Th(CO3)2(s) |
Th–O1 |
2.45 ± 0.05d |
8.9 ± 0.04 |
0.005 |
Th–O2 |
2.63 ± 0.04 |
3.4 ± 0.05 |
0.006 |
Th–C |
2.96 ± 0.11 |
4.8 ± 0.08 |
0.0077 |
Th–Th |
3.96 ± 0.17 |
12.0 ± 0.13 |
0.0084 |
Th_pH 2.0 |
Th–O1 |
2.41 ± 0.03 |
7.8 ± 0.05 |
0.0055 |
Th–O2 |
2.56 ± 0.03 |
3.2 ± 0.02 |
0.0065 |
Th–C |
2.98 ± 0.61 |
4.9 ± 0.21 |
0.0083 |
Th_pH 5.0 |
Th–O1 |
2.38 ± 0.02 |
3.6 ± 0.04 |
0.0034 |
Th–O2 |
2.53 ± 0.04 |
2.8 ± 0.05 |
0.0058 |
Th–C |
2.97 ± 0.67 |
4.9 ± 0.24 |
0.0074 |
Th–Th |
4.01 ± 0.19 |
12.4 ± 0.22 |
0.0088 |
Surface complexation modeling
In an initial attempt to model the sorption edges by using simple sorption sites (SOTh3+ species), the fitted results underestimate the sorption edge under high pH conditions. Therefore, we tentatively postulate that the second surface complex ((SO)2Th(OH)3− species) and Th(IV) surface precipitate (i.e., Th(OH)4(s)) should be taken into account. Fig. 6A and B show the surface complexation modeling of Th(IV) on amidoxime/graphene oxide composites at I = 0.001 and 0.1 mol L−1 NaCl, respectively. The optimized parameters based on a goodness-of-fit criterion are summarized in Table 4. As shown in Table 4, the values of log
K+ and log
K− for amidoxime/graphene oxide composites (3.64, −5.35) are obtained by fitting their potentiometric acid–base titration data in 0.01 mol L−1 NaCl solution. It is seen from Fig. 6A and B that diffuse layer modeling (DLM) can satisfactorily describe the sorption of Th(IV) on amidoxime/graphene oxide composites with increasing pH under atmospheric conditions by assuming the existence of two inner-sphere surface complexes (i.e., SOTh3+ and (SO)2Th(OH)3−) and a surface precipitate (Th(OH)4(s)). It was also found that the main sorption species of Th(IV) on amidoxime/graphene oxide composites are SOTh3+, (SO)2Th(OH)3− and Th(OH)4(s) species at pH <4.5, 4.5–6.0 and >6.0, respectively. The fitting results of surface complexation modeling indicate that the sorption mechanism of Th(IV) on amidoxime/graphene oxide composites was inner-sphere surface complexation at pH <6.0, whereas the surface precipitate (i.e., Th(OH)4(s)) dominates the Th(IV) sorption on amidoxime/graphene oxide composites at pH >6.0.
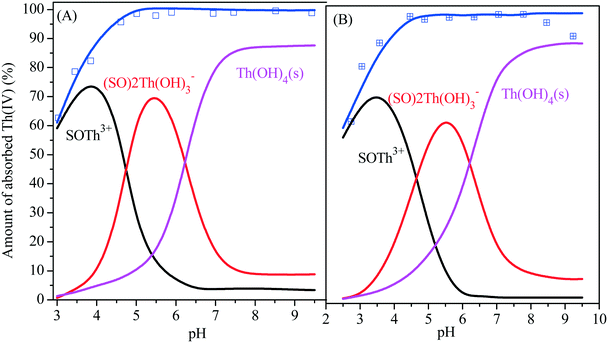 |
| Fig. 6 Surface complexation modeling of Th(IV) sorption on amidoxime/graphene oxide composites at 0.001 (A) and 0.1 mol L−1 NaCl (B), C0 = 10.0 mg L−1, I = 0.01 mol L−1 NaCl, m/v = 0.5 g L−1, T = 293 K. | |
Table 4 The optimized parameters of surface complexation modeling of Th(IV) sorption on amidoxime/graphene oxide composites
Equations |
log Ka |
Equilibration constants.
Standard deviation.
Data from ref. 51.
|
Protonation/preprotonation |
SOH + H+ = SOH2+ |
3.64 ± 1.53b |
SOH = SO− + H+ |
−5.35 ± 2.21 |
Surface complexation/precipitation reactions |
SOH + Th4+ = SOTh3+ + H+ |
3.53 ± 2.77 |
2SOH + Th4+ + 3H2O = (SO)2Th(OH)3− + 5H+ |
−2.05 ± 3.66 |
Th4+ + 4H2O = Th(OH)4(s) + 4H+ |
47c |
Conclusions
As-prepared amidoxime/graphene oxide composites present a variety of nitrogen- and oxygen-containing functional groups. The batch sorption experiments indicate that the sorption kinetics and isotherms of Th(IV) on amidoxime/graphene oxide composites can be satisfactorily simulated by a pseudo-second-order kinetic model and a Langmuir model, respectively. The maximum sorption capacity of Th(IV) on amidoxime/graphene oxide composites calculated from the Langmuir model is 123.46 mg g−1 at pH 2.0 and 293 K. According to EXAFS analysis, the presence of a Th–C shell indicates that the inner-sphere surface complexation dominates the sorption of Th(IV) on amidoxime/graphene oxide composites at pH 2.0, whereas the occurrence of a Th–Th shell reveals that a surface precipitate dominates the sorption of Th(IV) on amidoxime/graphene oxide composites at pH 5.0. The pH-dependent sorption behaviors of Th(IV) on amidoxime/graphene oxide composites can be satisfactorily simulated by double layer modeling using two inner-sphere surface complexes (i.e., SOTh3+ and (SO)2Th(OH)3− species) and a surface precipitate. These observations indicate that the amidoxime/graphene oxide composites can be regarded as suitable materials for the preconcentration and immobilization of thorium and other tetravalent actinides in environmental cleanup and nuclear waste management, especially at low pH. Because of the rapid development of high-tech industries, GO-based nanoparticles can be mass-produced at lower prices nowadays, which is crucial for the design of cost-effective materials suitable for the encapsulation and disposal of radionuclides in environmental remediation.
Acknowledgements
Financial support from the Natural Science Foundation of China (21477133; 41273134, 91326202; 21225730), the Fundamental Research Funds for the Central Universities, the Jiangsu Provincial Key Laboratory of Radiation Medicine and Protection and the Priority Academic Program Development of Jiangsu Higher Education Institutions is acknowledged.
Notes and references
- Y. B. Sun, J. X. Li and X. K. Wang, Geochim. Cosmochim. Acta, 2014, 140, 621–643 CrossRef CAS.
- A. Kowal-Fouchard, R. Drot, E. Simoni and J. J. Ehrhardt, Environ. Sci. Technol., 2004, 38, 1399–1407 CrossRef CAS PubMed.
- Y. Tachi and K. Yotsuji, Geochim. Cosmochim. Acta, 2014, 132, 75–93 CrossRef CAS.
- Y. B. Sun, R. Zhang, C. C. Ding, X. X. Wang, W. C. Cheng, C. L. Chen and X. K. Wang, Geochim. Cosmochim. Acta, 2016, 180, 51–65 CrossRef CAS.
- T. D. Waite, J. A. Davis, T. E. Payne, G. A. Waychunas and N. Xu, Geochim. Cosmochim. Acta, 1994, 58, 5465–5478 CrossRef CAS.
- D. M. Sherman, C. L. Peacock and C. G. Hubbard, Geochim. Cosmochim. Acta, 2008, 72, 298–310 CrossRef CAS.
- S. L. Estes, Y. Arai, U. Becker, S. Fernando, K. Yuan, R. C. Ewing, J. M. Zhang, T. Shibata and B. A. Powell, Geochim. Cosmochim. Acta, 2013, 122, 430–447 CrossRef CAS.
- Y. B. Sun, C. L. Chen, X. L. Tan, D. D. Shao, J. X. Li, G. X. Zhao, S. B. Yang, Q. Wang and X. K. Wang, Dalton Trans., 2012, 41, 13388–13394 RSC.
- C. C. Ding, W. C. Cheng, Y. B. Sun and X. K. Wang, J. Hazard. Mater., 2015, 295, 127–137 CrossRef CAS PubMed.
- F. Seco, C. Hennig, J. de Pablo, M. Rovira, I. Rojo, V. Marti, J. Gimenez, L. Duro, M. Grive and J. Bruno, Environ. Sci. Technol., 2009, 43, 2825–2830 CrossRef CAS PubMed.
- C. K. D. Hsi and D. Langmuir, Geochim. Cosmochim. Acta, 1985, 49, 1931–1941 CrossRef CAS.
- Y. B. Sun, S. T. Yang, G. D. Sheng, Z. Q. Guo, X. L. Tan, J. Z. Xu and X. K. Wang, Sep. Purif. Technol., 2011, 83, 196–203 CrossRef.
- C. Hennig, S. Weiss, D. Banerjee, E. Brendler, V. Honkimaki, G. Cuello, A. Ikeda-Ohno, A. C. Scheinost and H. Zanker, Geochim. Cosmochim. Acta, 2013, 103, 197–212 CrossRef CAS.
- L. Y. Yuan, Z. Q. Bai, R. Zhao, Y. L. Liu, Z. J. Li, S. Q. Chu, L. R. Zheng, J. Zhang, Y. L. Zhao, Z. F. Chai and W. Q. Shi, ACS Appl. Mater. Interfaces, 2014, 6, 4786–4796 CAS.
- C. C. Ding, W. C. Cheng, Y. B. Sun and X. K. Wang, Geochim. Cosmochim. Acta, 2015, 165, 86–107 CrossRef CAS.
- X. X. Wang, S. B. Yang, W. Q. Shi, J. X. Li, T. Hayat and X. K. Wang, Environ. Sci. Technol., 2015, 49, 11721–11728 CrossRef CAS PubMed.
- Z. J. Li, L. Wang, L. Y. Yuan, C. L. Xiao, L. Mei, L. R. Zheng, J. Zhang, J. H. Yang, Y. L. Zhao, Z. T. Zhu, Z. F. Chai and W. Q. Shi, J. Hazard. Mater., 2015, 290, 26–33 CrossRef CAS PubMed.
- Z. Q. Bai, L. Y. Yuan, L. Zhu, Z. R. Liu, S. Q. Chu, L. R. Zheng, J. Zhang, Z. F. Chai and W. Q. Shi, J. Mater. Chem. A, 2015, 3, 525–534 CAS.
- C. L. Chen, X. L. Li, D. L. Zhao, X. L. Tan and X. K. Wang, Colloids Surf., A, 2007, 302, 449–454 CrossRef CAS.
- S. B. Yang, C. C. Ding, W. C. Cheng, Z. X. Jin and Y. B. Sun, J. Mol. Liq., 2015, 204, 170–175 CrossRef CAS.
- J. Wang, B. L. Chen and B. S. Xing, Environ. Sci. Technol., 2016, 50, 3798–3808 CrossRef CAS PubMed.
- X. X. Chen and B. L. Chen, Environ. Sci. Technol., 2015, 49, 6181–6189 CrossRef CAS PubMed.
- Y. Shen and B. L. Chen, Environ. Sci. Technol., 2015, 49, 7364–7372 CrossRef CAS PubMed.
- Y. Shen, Q. L. Fang and B. L. Chen, Environ. Sci. Technol., 2015, 49, 67–84 CrossRef CAS PubMed.
- A. Y. Romanchuk, A. S. Slesarev, S. N. Kalmykov, D. V. Kosynkin and J. M. Tour, Phys. Chem. Chem. Phys., 2013, 15, 2321–2327 RSC.
- Y. B. Sun, Q. Wang, C. L. Chen, X. L. Tan and X. K. Wang, Environ. Sci. Technol., 2012, 46, 6020–6027 CrossRef CAS PubMed.
- C. C. Ding, W. C. Cheng, Y. B. Sun and X. K. Wang, Dalton Trans., 2014, 43, 3888–3896 RSC.
- Y. Xie, E. M. Helvenston, L. C. Shilller-Nickles and B. A. Powell, Environ. Sci. Technol., 2016, 50, 1821–1827 CrossRef CAS PubMed.
- C. D. Williams and P. Carbone, Environ. Sci. Technol., 2016, 50, 3875–3881 CrossRef CAS PubMed.
- G. Zhao, J. Li, X. Ren, C. Chen and X. Wang, Environ. Sci. Technol., 2011, 45, 10454–10462 CrossRef CAS PubMed.
- Y. B. Sun, S. B. Yang, Y. Chen, C. C. Ding, W. C. Cheng and X. K. Wang, Environ. Sci. Technol., 2015, 49, 4255–4262 CrossRef CAS PubMed.
- Y. B. Sun, S. B. Yang, C. C. Ding, Z. X. Jin and W. C. Cheng, RSC Adv., 2015, 5, 24886–24892 RSC.
- Z. X. Jin, X. X. Wang, Y. B. Sun, Y. J. Ai and X. K. Wang, Environ. Sci. Technol., 2015, 49, 9168–9175 CrossRef CAS PubMed.
- J. Li, S. Zhang, C. Chen, G. Zhao, X. Yang, J. Li and X. Wang, ACS Appl. Mater. Interfaces, 2012, 4, 4991–5000 CAS.
- V. Chandra, J. Park, Y. Chun, J. W. Lee, I.-C. Hwang and K. S. Kim, ACS Nano, 2010, 4, 3979–3986 CrossRef CAS PubMed.
- Y. B. Sun, C. C. Ding, W. C. Cheng and X. K. Wang, J. Hazard. Mater., 2014, 280, 399–408 CrossRef CAS PubMed.
- M. Xing, L. Xu and J. Wang, J. Hazard. Mater., 2016, 301, 286–296 CrossRef CAS PubMed.
- Y. B. Sun, D. D. Shao, C. L. Chen, S. B. Yang and X. K. Wang, Environ. Sci. Technol., 2013, 47, 9904–9910 CrossRef CAS PubMed.
- Y. Wang, Z. S. Wang, R. Ang, J. J. Yang, N. Liu, J. L. Liao, Y. Y. Yang and J. Tang, RSC Adv., 2015, 5, 89309–89318 RSC.
- H. Chen, D. D. Shao, J. X. Li and X. K. Wang, Chem. Eng. J., 2014, 254, 623–634 CrossRef CAS.
- D. D. Shao, J. X. Li and X. K. Wang, Sci. China: Chem., 2014, 57, 1449–1458 CrossRef CAS.
- F. H. Wang, H. P. Li, Q. Liu, Z. S. Li, R. M. Li, H. S. Zhang, L. H. Liu, G. A. Emelchenko and J. Wang, Sci. Rep., 2016, 6, 19367 CrossRef CAS PubMed.
- Y. B. Sun, Z.-Y. Wu, X. X. Wang, C. C. Ding, W. C. Cheng, S.-H. Yu and X. K. Wang, Environ. Sci. Technol., 2016, 50, 4459–4467 CrossRef CAS PubMed.
- C. C. Ding, W. C. Cheng, X. X. Wang, Z.-Y. Wu, Y. B. Sun, X. K. Wang and S.-H. Yu, J. Hazard. Mater., 2016, 313, 253–261 CrossRef CAS PubMed.
- J. Y. Huang, Z. W. Wu, L. W. Chen and Y. B. Sun, J. Mol. Liq., 2015, 209, 753–758 CrossRef CAS.
- M. O. Barnett, P. M. Jardine and S. C. Brooks, Environ. Sci. Technol., 2002, 36, 937–942 CrossRef CAS PubMed.
- W. Um, R. J. Serne and K. M. Krupka, Environ. Sci. Technol., 2007, 41, 3587–3592 CrossRef CAS PubMed.
- J. G. Catalano and G. E. Brown, Geochim. Cosmochim. Acta, 2005, 69, 2995–3005 CrossRef CAS.
- H. Xu, G. Li, J. Li, C. Chen and X. Ren, J. Mol. Liq., 2016, 213, 58–68 CrossRef CAS.
- W. S. Hummers and R. E. Offeman, J. Am. Chem. Soc., 1958, 80, 1339 CrossRef CAS.
- M. Newville, J. Synchrotron Radiat., 2001, 8, 96–100 CrossRef CAS PubMed.
- B. Ravel and M. Newville, J. Synchrotron Radiat., 2005, 12, 537–541 CrossRef CAS PubMed.
- N. A. Yamnova, D. Y. Pushcharovskii and A. V. Voloshin, Soviet Physics - Doklady, 1990, 35, 12–14 Search PubMed.
-
J. P. Gustafsson, http://www.lwr.kth.se/English/OurSOrware/vminteq/index.htm, 2009.
- Y. B. Sun, S. T. Yang, G. D. Sheng, Z. Q. Guo and X. K. Wang, J. Environ. Radioact., 2012, 105, 40–47 CrossRef CAS PubMed.
- S. Kumar, R. Kumar, V. K. Jindal and L. M. Bharadwaj, Mater. Lett., 2008, 62, 731–734 CrossRef CAS.
- A. Kuznetsova, I. Popova, J. T. Yates, M. J. Bronikowski, C. B. Huffman, J. Liu, R. E. Smalley, H. H. Hwu and J. G. G. Chen, J. Am. Chem. Soc., 2001, 123, 10699–10704 CrossRef CAS PubMed.
- Z. Li, F. Chen, L. Yuan, Y. Liu, Y. Zhao, Z. Chai and W. Shi, Chem. Eng. J., 2012, 210, 539–546 CrossRef CAS.
- N. A. Kumar, H.-J. Choi, Y. R. Shin, D. W. Chang, L. Dai and J.-B. Baek, ACS Nano, 2012, 6, 1715–1723 CrossRef CAS PubMed.
- M. T. Martinez, M. A. Callejas, A. M. Benito, M. Cochet, T. Seeger, A. Anson, J. Schreiber, C. Gordon, C. Marhic, O. Chauvet, J. L. G. Fierro and W. K. Maser, Carbon, 2003, 41, 2247–2256 CrossRef CAS.
- V. Neck and J. I. Kim, Radiochim. Acta, 2001, 89, 1–16 CrossRef CAS.
- V. Neck, R. Muller, M. Bouby, M. Altmaier, J. Rothe, M. A. Denecke and J. I. Kim, Radiochim. Acta, 2002, 90, 485–494 CrossRef CAS.
- A. Schierz and H. Zaenker, Environ. Pollut., 2009, 157, 1088–1094 CrossRef CAS PubMed.
- J. S. Tang, X. B. Jing, B. C. Wang and F. S. Wang, Synth. Met., 1988, 24, 231–238 CrossRef CAS.
- C. L. Chen and X. K. Wang, Appl. Geochem., 2007, 22, 436–445 CrossRef CAS.
- X. L. Tan, X. K. Wang, M. Fang and C. L. Chen, Colloids Surf., A, 2007, 296, 109–116 CrossRef CAS.
- C. L. Chen and X. K. Wang, Appl. Radiat. Isot., 2007, 65, 155–163 CrossRef CAS PubMed.
- G. D. Sheng, J. Hu and X. K. Wang, Appl. Radiat. Isot., 2008, 66, 1313–1320 CrossRef CAS PubMed.
- A. K. Kaygun and S. Akyil, J. Hazard. Mater., 2007, 147, 357–362 CrossRef CAS PubMed.
- N. Demirel, M. Merdivan, N. Pirinccioglu and C. Hamamci, Anal. Chim. Acta, 2003, 485, 213–219 CrossRef CAS.
Footnote |
† Electronic supplementary information (ESI) available: The fitting of sorption kinetics and isotherms, distribution of Th(IV) speciation. See DOI: 10.1039/c6en00470a |
|
This journal is © The Royal Society of Chemistry 2017 |
Click here to see how this site uses Cookies. View our privacy policy here.