Transferring mixtures of chemicals from sediment to a bioassay using silicone-based passive sampling and dosing†
Received
7th June 2017
, Accepted 19th September 2017
First published on 19th September 2017
Abstract
Environmental mixtures of chemicals consist of a countless number of compounds with unknown identity and quantity. Yet, chemical regulation is mainly built around the assessment of single chemicals. Existing frameworks for assessing the toxicity of mixtures require that both the chemical composition and quantity are known. Quantitative analyses of the chemical composition of environmental mixtures are however extremely challenging and resource-demanding. Bioassays may therefore serve as a useful approach for investigating the combined toxicity of environmental mixtures of chemicals in a cost-efficient and holistic manner. In this study, an unknown environmental mixture of bioavailable semi-hydrophobic to hydrophobic chemicals was sampled from a contaminated sediment in a coastal Baltic Sea area using silicone polydimethylsiloxane (PDMS) as an equilibrium passive sampler. The chemical mixture was transferred to a PDMS-based passive dosing system, and its applicability was demonstrated using green algae Tetraselmis suecica in a cell viability assay. The proportion of dead cells increased significantly with increasing exposure level and in a dose–response manner. At an ambient concentration, the proportion of dead cells in the population was nearly doubled compared to the control; however, the difference was non-significant due to high inter-replicate variability and a low number of replicates. The validation of the test system regarding equilibrium sampling, loading efficiency into the passive dosing polymer, stability of the mixture composition, and low algal mortality in control treatments demonstrates that combining equilibrium passive sampling and passive dosing is a promising tool for investigating the toxicity of bioavailable semi-hydrophobic and hydrophobic chemicals in complex environmental mixtures.
Environmental significance
Managing the continuously increasing use of chemicals is a major challenge for society. One aspect concerns how to assess the risk posed by complex mixtures of chemicals occurring in the environment. There is only limited knowledge of what effects the constant exposure to complex mixtures has on organisms, populations and ecosystems. We present a method that combines equilibrium passive sampling and equilibrium passive dosing to transfer complex chemical mixtures from the environment to laboratory-based bioassays. We tested our method by assessing the toxicity of a mixture of chemicals from a coastal Baltic Sea sediment on the algae Tetraselmis suecica. We observed significantly increasing effects on cell viability with increasing exposure levels.
|
Introduction
Organic contaminants accumulate in the organic carbon fraction of sediment.1,2 This accumulation leads to exposure of sediment-living organisms to a complex mixture of chemicals. Chemical regulation is today mainly built around the assessment of single chemicals, as exemplified by EU's REACH regulation (Registration, Evaluation, Authorization and Restriction of Chemicals; EC 1907/2006) and EU's regulation on the use of Plant Protection Products (EC 1107/2009). Our knowledge of the potential effects of the environmental mixtures of chemicals on organisms, populations and ecosystems is scarce. Yet, there is an increasing body of evidence of mixture toxicity, demonstrating that even if each chemical is present at the no-effect concentration or below, the mixture of all chemicals present may cause toxic effects.3–6 The theoretical basis for mixture toxicity assessment focuses mainly on the concepts of concentration addition and independent action that describe the mixture effects of components having similar and dissimilar modes of action, respectively.3,7–9 However, these concepts can only be applied if the mixture composition is known. Mixture toxicity estimates based on chemicals identified by chemical analysis as part of environmental monitoring thus ignore all potentially toxic but unknown chemicals that are also present in the environmental sample. This deficit in currently used risk assessment approaches was highlighted by Tang et al.,10 who demonstrated that the toxicity predicted based on 64 chemicals observed in monitoring programs (including pharmaceuticals, pesticides and chemicals from consumer products), corresponded to less than 1% of the observed non-receptor mediated toxicity in Australian drinking water. In a study by Vermeirssen et al.,11 only a minor part of the non-receptor mediated toxicity could be explained by predictions based on concentration addition of 18 chemicals analyzed in sewage treatment plant effluents. In contrast, in the same study approximately 65% of the observed specific effect, measured as photosynthesis inhibition, could be explained by 6 herbicides analyzed in these effluents. In addition, predictions of specific toxicity such as estrogenic activity, based on chemical analyses, are typically well correlated with the effects observed in bioassays.12,13
An important toxicity pathway for organic chemicals in many organisms is their penetration into the lipid bilayer of cell membranes causing loss of cell integrity and membrane function (i.e. non-specific or baseline toxicity). In many environments, chemicals are present below the threshold level for compound-specific toxicity.14 On a global scale, non-specific toxicity is likely to determine the overall toxic effect exerted by the mixture of innumerable chemicals present in most environments.14 Non-specific toxicity is additive and directly related to the chemical activity of the sample.15
The freely dissolved concentration (which forms the basis to calculate chemical activity in any matrix) of organic contaminants can be assessed using equilibrium passive samplers.16 The chemical partitions between the sample matrix and the sampler polymer until it reaches thermodynamic equilibrium between these two phases, and the freely dissolved concentration in the sample matrix is then derived from the amount of chemical in the passive sampler at equilibrium divided by the partition coefficient between the sampler polymer and sample matrix.17 This technique is used in field studies to measure the concentrations of organic chemicals in sediment pore water and water down to pg L−1 levels.18–20 Equilibrium passive sampling has also been applied to assess the toxicity of environmental mixtures of chemicals, by adding concentrated extracts from passive samplers to bioassay systems.21,22
It is a challenge to maintain stable exposure concentrations in ecotoxicological testing of organic chemicals, since these chemicals have physicochemical properties that make them partition to surfaces or organic matter in the test vials or volatilize to air. Passive dosing can overcome these challenges, while also avoiding the usage of solvent spiking during the test.23–25 Passive dosing works on the reversed principle of passive sampling. The test chemicals are loaded into a dosing polymer, which acts as a quasi-inexhaustible source, and via equilibrium partitioning between the polymer and the exposure media a stable exposure concentration can be maintained throughout the test, even for hydrophobic chemicals. Various formats and polymers of the dosing phase have been used and a range of exposure concentrations, up to the aqueous solubility level, have been assessed.24,26–28 Moreover, passive dosing is useful for toxicity testing of mixtures of chemicals. For instance, Smith et al.29 used passive dosing to assess the increased toxicity of an artificial mixture of polycyclic aromatic hydrocarbons (PAHs) in which the individual chemicals showed limited toxicity. Still, most toxicity studies performed with passive dosing have focused on artificial mixtures of a limited number of chemicals from a few chemical classes, such as PAHs and pesticides,30,31 or fractionated solvent extracts of chemical mixtures from sediments.28
Combining equilibrium passive sampling and passive dosing enables assessment of the toxicity of complex mixtures of chemicals present in the environment, in controlled laboratory experiments. Although straightforward in theory, the transfer of mixtures of chemicals from the environment to the test organisms or cells is associated with a number of challenges, primarily regarding the dimensions of the sampling and dosing phase and the maintenance of the chemical mixture composition.32 In a recent study, sheets of polydimethylsiloxane (PDMS) were deployed along the Belgian coast and were thereafter used as a passive dosing source of environmental mixtures of chemicals in bioassays.33 No dilutions or up-concentrations of the chemical mixture were performed.
The aim of this study was to develop a combined passive sampling-passive dosing method to test the toxicity of the environmental mixture of semi-hydrophobic to hydrophobic chemicals from a sediment sample collected in a contaminated bay of the Baltic Sea. The mixture toxicity of the chemicals was evaluated using a cell viability assay with the green microalgae Tetraselmis suecica (Kylin) Butcher, 1959. The test system was validated with regard to equilibrium sampling, loading efficiency into the passive dosing polymer, stability of the mixture composition, and the background mortality of the test organism.
Materials and methods
Workflow – passive sampling and loading of the environmental mixture of chemicals into the dosing polymer
General test design.
The workflow of the method to transfer environmental mixtures of chemicals from sediment to a laboratory-based bioassay is illustrated in Fig. 1: (1) the environmental mixture of chemicals was sampled from sediment by ex situ equilibrium passive sampling, using jars with a thin silicone coating on the inside of the glass walls. Silicone polymers are suitable for sampling of the freely dissolved organic chemicals which are available for partitioning and biological uptake, and they have most commonly been used for chemicals with log
Kow > 3, corresponding to those chemicals that accumulate in sediment. Still, polymer-water partition coefficients for chemicals with log
Kow down to ∼1 have been determined,34 but for those chemicals the enrichment from water to silicone is limited. The mixture of organic chemicals in the passive sampling polymer was solvent extracted with acetone:n-hexane. (2) The extract was then transferred to a passive dosing polymer cast at the bottom of each exposure vial. The exposure level of the chemical mixture was varied from the environmental level (1
:
1) to a 200-fold concentrated level (1
:
200) by using different ratios between the mass of the dosing polymer and mass of the sampling polymer, i.e. 1
:
1 to 1
:
200. To increase the exposure level, a higher sampling-to-dosing polymer mass ratio was used. After solvent evaporation, 1 mL of Milli-Q water was added and the system was allowed to equilibrate. The Milli-Q water was discarded (see below). The test medium was added and the system was allowed to equilibrate. (3) The algae T. suecica were added to the dosing vial. A 72 h cell viability test was performed to assess the response of T. suecica to the exposure of the chemical mixture at four different levels. Staining of algae was done to distinguish dead cells from live ones.35
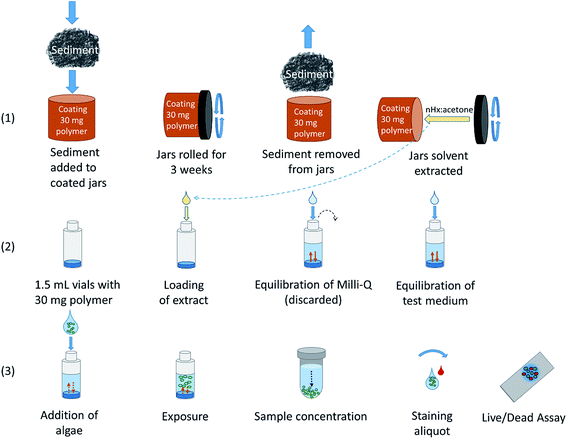 |
| Fig. 1 Workflow for the transfer of the environmental mixture of organic chemicals from sediment to the bioassay with algae at environmental levels and then to the cell viability test. (1) Equilibrium passive sampling of bioavailable organic chemicals in sediment. (2) Loading and equilibrium passive dosing. (3) Exposure of algae and quantification of cell viability. | |
Preparation of equilibrium passive sampling jars and dosing vials
Jars coated with a silicone polymer on the inner vertical walls have been used for passive sampling of PAHs in soils36 and hexachlorobenzene and PCBs in sediment.18,19,37 Coating of 180 mL amber glass jars was done as described by Reichenberg et al.,36 with slight modifications. Jars were rolling horizontally and a (Dow Corning® 1-2577) polymer–pentane solution was added to the jars. Jars were coated with four different thicknesses. The pentane was allowed to evaporate, and the polymer was cured at 110 °C overnight. Oligomers present in the coating were removed by washing the jars with ethyl acetate and methanol, 3 mL each, three times, by rolling the jars for 10 min per solvent wash. The jars were dried in two steps, first at room temperature and then finally at 110 °C for at least 1 h to ensure that the solvent had fully evaporated. The mass of the polymer in the jar was determined by weighing the jar before and after casting. The jars were stored covered with a lid until use to prevent sampling of pollutants from the laboratory air.
For passive dosing, vials of 1.5 mL (for cell viability tests) or 10 mL (for loading efficiency tests) were used and cast with 0.03 g ± 1% or 0.5 g ± 1% polymer, respectively. The ±1% precision interval minimizes the variation of the exposure concentrations that possibly originates in the test system itself. The casting of vials has previously been described by e.g. Birch et al.38 The polymer was prepared according to the instructions of the supplier and kept at 5 °C for one hour before casting. Vials were kept at 5 °C for 72 h, at room temperature for 72 h, and then at 110 °C overnight to cure. The vials were washed 3 times with 3 mL ethanol (0.3 mL for the 1.5 mL vials), with a total washing time of 48 h, and then washed with Milli-Q water 3 × 3 mL (0.3 mL for the 1.5 mL vials), for a total time of 24 h, and subsequently dried at 110 °C for one hour. Vials were capped and stored in the dark until use.
Validation of equilibrium partitioning in sampling jars
Equilibrium partitioning between sediment and the sampling polymer in the coated jars was validated after three weeks of equilibration by using jars with four different polymer thicknesses: 11 μm, 14 μm, 20 μm and 30 μm, corresponding to polymer masses of approximately 0.11 g, 0.14 g, 0.19 g and 0.29 g, respectively. Equilibrium was assessed by a linear regression of the mass of chemical in the sampling polymer vs. the mass of silicone in the jars.36 For this test, we used 6 PCB congeners with 3 to 7 chlorine atoms as model chemicals. These chemicals represent a relevant range in physicochemical properties and are commonly found in environmental samples such as sediment. The coated jars for each coating thickness were filled with wet sediment (165 ± 9 g ww; mean ± SD, n = 12) collected from the central area of Stockholm City. Sodium azide (NaN3, approx. 0.1 g) was added to each jar to prevent microbial activity. The jars were sealed with a lid covered with aluminum foil on the inside and rolled horizontally on a roller mixer (Stuart SRT9D) at 8 rpm. After three weeks of rolling at room temperature, the sediment was removed from the jars. The jars were rinsed with Milli-Q water, wiped and dried thoroughly with lint-free tissues.37 The chemicals in the polymer were extracted with 3 mL solvent by rolling the jars horizontally for 30 min.18 We used a 1
:
1 mixture of acetone:n-hexane for extracting HOCs from the polymer inside the jars, instead of n-hexane only as previously used by Jahnke et al. (where 3–7 Cl PCBs were targeted18), since we aimed to capture a wider range of chemicals. The extraction was repeated once and the extracts were combined. After evaluation of the equilibrium status, only jars with the thickest polymer layer (30 μm) were used in replicates to sample the chemical mixture from sediment and to maximize the amount of pollutants being extracted for the bioassay.
Chemical analysis
The chemicals in the silicone coating of the sampling jars were extracted for testing of either the extraction efficiency or for validating the equilibrium partitioning after three weeks of sampling. To evaluate the equilibrium between the sampling polymer and sediment, the polymer in the jars was extracted twice and PCBs were analyzed. A mixture of 7 stable isotope-labeled PCBs (3–7 Cl, Text ESI S1†) as internal surrogate standards was added to the jars in the first extraction step. The extracts were cleaned on an open triple silica gel column, 1 cm in diameter with 3 cm silica gel per layer, from top to bottom: SiO2/H2O (10% Milli-Q water w/w), SiO2/KOH (36% KOH w/w) and SiO2/H2SO4 (40% H2SO4 w/w).39 The volume of the extract was reduced and the recovery standard (PCB 53) was added.
The efficiency of the extraction method was evaluated by analyzing both PAHs and PCBs. For this the polymer in the jars was extracted three times with 3 mL of acetone:n-hexane (1
:
1) each. The two first extracts were combined, while the third extract was analyzed separately to determine the amount of PAHs and PCBs remaining in the polymer after two extractions. Labeled internal surrogate standards (15 PAHs and 7 PCBs, see the Chemicals and materials section, Text ESI S1†) were added to the combined extract (containing the first and second extracts) and separately to the third extract. Clean up was done with dimethylformamide and a SiO2/H2O (10% Milli-Q water w/w) column as described by Mandalakis et al.40 following the modifications described by Mustajärvi et al.41 to include both PAHs and PCBs in the analysis. The extracts were reduced in volume prior to instrumental analysis and the recovery standards (PCB 53 and acenaphthylene) were added. All samples were analyzed by gas chromatography (GC, with a DB-5 column 30 m, 25 mm inner Ø and 0.25 mm film thickness) coupled with mass spectrometry (MS, Thermo Scientific™ ISQ™), operating in electron impact mode (EI+, 70 eV) and in single ion monitoring acquisition mode.
Loading chemicals into the passive dosing polymer.
To validate the loading efficiency of the chemicals into the dosing polymer and to ensure stable exposure levels, the polymer in the dosing vials was spiked with 1 mL of a n-hexane solution containing the test chemicals (anthracene, pyrene, benzo(a)pyrene, PCB 28 and PCB 52, log
Kow 4.6–6.3) (Table ESI S1†), and the solvent was left to evaporate. Each chemical was loaded at two different levels into the polymer, and 9 replicates were prepared per level. Two 10 mL batches of Milli-Q water were equilibrated successively with the silicone polymer by horizontal shaking (170 rpm, 18 h). The first batch of water equilibrated with the dosing silicone polymer was discarded as elevated concentrations (above equilibrium partitioning) have been observed in the first batch of water in a previous study, where chemicals were loaded from a methanol–water solution.25 This was done as a safety precaution and might not have been necessary as a different loading method was used in this study than in Ribbenstedt et al.25 Analytes in the second batch of equilibrated water were extracted with 5 mL n-hexane after addition of a mixture of labeled internal surrogate standards containing D-anthracene, D-pyrene, D-benzo(a)pyrene, 13C-PCB 28 and 13C-PCB 52. The dosing polymer was extracted twice with 5 mL n-hexane for 24 h, and the extracts were combined. A mixture of internal surrogate standards, containing the same labeled PAH and PCB congeners as used for the water extraction, was thereafter added to an aliquot of the extract at expected levels for each chemical. The mass of the polymer extracts and aliquots were gravimetrically monitored to enable determination of the total mass of analytes in the extract. Quantification was done by GC-MS as described above.
The environmental mixture of chemicals was extracted from the equilibrium passive sampler as described above and loaded into the passive dosing polymer. For the loading, the n-hexane/acetone extract was reduced to 0.3 mL and added to the dosing vial, after which the solvent was allowed to evaporate. This loading method was chosen in order to avoid precipitation of the hydrophobic analytes extracted from the passive sampling polymer, which had been observed in pre-experiments using methanol or methanol–water solutions for loading into the passive dosing polymer. Loading methods based on solvent evaporation have previously been used for passive dosing with halogenated and non-halogenated aromatic chemicals.42,43 Also, recently Gilbert et al.44 loaded silicone polymers with PCBs using isooctane, which was thereafter allowed to evaporate. To confirm that all loading solvent had evaporated, the weight of dosing vials (n = 9) containing 0.03 g of polymer was determined before loading and after evaporation of the solvent.
Stability of dosing conditions.
To monitor potential shifts in the mixture composition during prolonged usage of the passive dosing vials, we loaded a mixture containing 9 chemicals with a range of log
Kow of 2.4–7.3 (Table ESI S2†) into the polymer (0.03 g PDMS) cast in vials. The mixture was dissolved in 0.3 mL n-hexane, added to the dosing vials, and the evaporation of the solvent was confirmed by weighing as described above. 1 mL Milli-Q water was equilibrated and discarded (see above). The mass of the chemicals in the polymer was monitored at three different time points; before the exposure test, when the vials had been used once, and when the vials had been used twice in a 72 h exposure test with algae T. suecica. The chemicals were extracted twice with 1 mL n-hexane for 24 h, and the extracts were combined and analyzed as described above.
Bioassay
Test organism.
The effects of exposure to the chemical mixture transferred from the field sediment were investigated using green algae T. suecica. This alga is recommended as standard test species in marine and brackish bioassays45 and has been used for investigating the effects of binary chemical mixtures4 in multi generation exposure tests with the pesticide diuron46 and in growth inhibition tests with PAHs and PCBs.47 The algae were cultivated continuously in f/2 medium48 using artificial sea water (Instant Ocean™, Aquarium Systems, 14‰), constant light with an intensity of 25 μE cm−2 s−1 and horizontal rotation (125 rpm).
72 h exposure using passive dosing and the subsequent cell viability test.
The toxicity of the chemical mixture was tested at four concentration levels; 1
:
1, 1
:
10, 1
:
50 and 1
:
200 (dosing polymer mass: sampling polymer mass), thus ranging from an environmental level exposure of the mixture to a 200-fold higher exposure. Vials loaded with corresponding levels of polymer extracts from coated blank jars served as controls. Each concentration and corresponding control was tested in triplicate, resulting in a total of 24 experimental units. Prior to exposure, 1 mL of Milli-Q water was equilibrated with the dosing polymer by horizontal shaking for 18 h at 170 rpm and discarded (Fig. 1(2)). Thereafter, 1 mL test medium was pre-equilibrated with the passive dosing silicone polymer. The exposure was started by replacing 0.3 mL of the equilibrated test medium with an equal volume of algal culture. The starting cell concentration was approx. 6 × 104 cells mL−1, which is within the concentration range recommended by the OECD guideline for growth inhibition tests.49 Replacing part of the pre-equilibrated solution and adding a sorptive phase of the algae decreases the initial exposure concentration by at least 30%, but, due to the continuous shaking (150 rpm) of the vials, the equilibrium concentration is expected to be restored within a few hours. The algae were exposed at room temperature, constant light of 70 μE m−2 s−1 and orbital shaking for 72 h, which is a standard test duration in growth inhibition tests49 and a suitable time considering how long it takes to reach equilibrium for hydrophobic organic contaminants between water and algae (<25 h).50 Nutrients were supplied with the f/2 medium at the start of the test.
The toxicity was evaluated using cell viability analysis (Fig. 1(3)). The test medium with the exposed algae (1 mL) was transferred to Eppendorf tubes, concentrated by centrifugation (3300g, 5 min), and the supernatant was discarded. The pellet with the overlaying 0.1 mL was vortexed; a single 25 μL aliquot was stained with 10 μL of the working solution of TO-PRO-1 iodide. To prepare the working solution, the stock was diluted 1
:
10 with the test medium to the concentration of 64.5 μg mL−1.35 After incubation in darkness for 20 minutes, the fraction of dead cells was determined by counting at least 200 cells in each of at least three fields of vision, using a fluorescence microscope (Leica, Leitz DMRB). Cells with any indication of a stained nucleus were assigned as dead.35
The stability and repeatability of the test conditions were validated by evaluating the variance in control incubations among the four independent experiments with ANOVA. Furthermore, a general linear model (GLM) with a normal error structure and log-link as implemented in STATISTICA 8.0 (StatSoft, 1984–2007) was used to evaluate the effect of the concentration (n = 3 for each exposure level) as a continuous variable (0 to 200) on cell viability. Controls were found statistically indistinguishable and therefore pooled in this model (n = 12). To evaluate the effect of exposure at ambient contaminant concentrations (1
:
1 treatment), an unpaired t-test with the exposed and the control algae was used. When the observed between-group difference was found to be below the significance level at α = 0.05, a power test was conducted to estimate the sample size needed to detect a significant difference given the variance and the mean difference observed. All bioassay data were Box–Cox transformed and the model residuals were evaluated using q–q plots, Table ESI S4.†
Results & discussion
Passive equilibrium sampling and extraction of the sampling polymer
Equilibrium partitioning between the sampling polymer and sediment after three weeks was tested by using jars with four different coating thicknesses. Equilibrium was established for PCBs with log
Kow up to 7.3 (PCB153), whereas the most hydrophobic PCB180 (log
Kow 7.7) needs longer sampling time to fully achieve equilibrium (Fig. ESI S1†). In order to increase the mass of chemicals sampled per jar for toxicological assessment, we aimed for thicker coatings in this study than those used in previous studies with coated jars that targeted chemical analysis only. Jahnke et al.18 used PDMS coating thicknesses between 2 and 8 μm and a sampling time of two weeks to establish equilibrium for PCBs in sediment, and Mäenpää et al.51 established equilibrium for PCBs in sediment after two weeks of sampling, using PDMS coating thicknesses up to 15 μm. Reichenberg et al.36 used 3–12 μm thick PDMS coatings and a sampling time of five days to establish equilibrium for PAHs in soil.
The extraction efficiency of the passive sampling polymer was evaluated by extracting the polymer three times. The first two extractions captured 93–100% and 91–100% of the individual PAHs and PCBs, respectively. As only minor fractions remained in the third extract, extracting the polymer twice was considered sufficient for the hydrophobic organic chemicals (HOCs) absorbed in the silicone. See Table ESI S6† for the concentrations of PAHs and PCBs in pore water.
Loading efficiency of chemicals into the passive dosing polymer
The method used to load the dosing polymer was evaluated by spiking polymer-cast vials with three PAHs and two PCBs (log
Kow 4.6–6.3). The chemical was extracted from the polymer, and its mass was quantified and compared to the nominal mass loaded into the vials. For anthracene, PCB 28 and PCB 52 the average mass of each individual chemical was 80–100% (range 60–150%) of the nominal mass and for pyrene and benzo(a)pyrene the average mass was 130–140% (range 90–260%) of the nominal mass (Fig. 2). It is possible that the high numbers observed for pyrene and benzo(a)pyrene (i.e., apparently a higher amount was quantified than what had been loaded into the silicone) is due to analytical challenges. Furthermore, the low reproducibility for loading some of the chemicals into the silicone compared to previous studies (e.g. Birch et al.38 and Smith et al.24), may be due to the loading method used in this study (solvent evaporation), which differs from the most common approach implying dissolving the chemicals in methanol and then subsequently adding small amounts of water to push the (hydrophobic) chemicals into the silicone38 or allowing chemicals partition into the silicone from a methanol solution.24,52
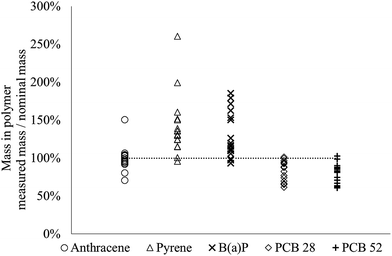 |
| Fig. 2 Measured versus nominal mass of five chemicals added to the polymer (n = 18). The dashed line indicates 100%. | |
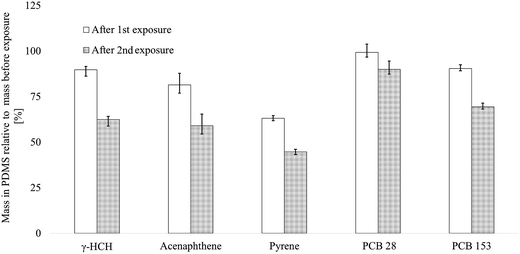 |
| Fig. 3 Mass of chemicals (γ-HCH, acenaphthene, pyrene, PCB 28 and PCB 153) in PDMS (0.03 g) used twice in 72 h passive dosing exposure, relative to the mass of chemicals in unused PDMS (0.03 g) set at 100%. Error bars represent min–max (n = 3). | |
As loading into the passive dosing polymer in this study involved evaporation of the spiking solvent, there is a risk of loss of chemicals due to volatilization. However, anthracene, which has the highest vapor pressure of the tested chemicals and thus should be the chemical most susceptible to losses via volatilization, had the best agreement between the measured and nominal mass (100%) (Fig. 2). These results indicate that volatilization losses were negligible for the tested chemicals.
Loading methods that include addition of a non-polar solvent such as n-hexane, dichloromethane, ethyl acetate or acetone to the dosing polymer cause swelling of the polymer, which might change its partitioning properties.53 Recently Stibany et al.54 used a loading approach where silicone o-rings were submerged in dodecylbenzene. The swelling was >14% as determined by the change in weight and no effects on the partitioning properties of the polymer were observed. A similar loading method was used by Bandow et al.,28 who argued that swelling of the polymer helps to ensure an even distribution of the chemical in the polymer during loading. In this study, the weight of the vials was controlled before loading and after evaporation of the loading solvent. As no significant difference in weight was observed, complete evaporation of the loading solvent (n-hexane) was confirmed. Rusina et al.53 tested the swelling of silicone when immersed in several non-polar solvents, including n-hexane, and stated that after evaporation of the solvent the original size of the polymer was regained.
Determination of Kpdms–w
The PDMS–water partition coefficient (Kpdms–w) was determined for the five chemicals used to determine the loading efficiency (Table 1). The relative standard deviation of the Kpdms–w was generally low (3–12%), which supports the assumption that reproducible exposure levels in the passive dosing vials can be achieved. The measured Kpdms–w values were compared with partition coefficients from the literature, determined for the same polymer and for several other types of silicone polymers, covering various suppliers (literature polymer partition coefficient denoted as Kp–w; Table 1). There was a close agreement between our measured Kpdms–w and Kp–w from the literature for anthracene and pyrene. For the more hydrophobic chemicals benzo(a)pyrene, PCB 28 and PCB 52, our Kpdms–w values were lower than Kp–w by a factor of 4–5 (Table 1), but since the uncertainties of our Kpdms–w increased with hydrophobicity, no significant differences between our measured Kpdms–w and Kp–w from the literature were observed. Smedes et al.55 showed that partition coefficients vary by up to 0.6 log units for the same polymer from different suppliers. Hence, partition coefficients are polymer-specific as the content of different additives and fillers (e.g. diatomaceous earth44) may vary between suppliers and thus cause differences in partitioning properties. In an extensive review by DiFilippo et al.,56 it was concluded that improper measuring techniques were a main source of error for the Kp–w and as opposed to Smedes et al., no significant effect associated with the supplier of the polymers was found. The importance of the methodology was also acknowledged by Poerschmann et al.,57 who demonstrated that sampling time and sampling mode (static or dynamic) affected the partition coefficients.
Table 1 Polymer-water partition coefficients for PDMS (Kpdms–w) other types of silicone polymers (Kp–w) for anthracene, pyrene, benzo(a)pyrene, PCB 28 and PCB 52. Data from the literature are presented as an average with the standard deviation of the reported values. See Table ESI S7 for information on the literature data used
|
This study |
Literature |
Ref. |
log Kpdms–w (±SD) |
log Kp–w (±SD) |
Anthracene |
3.9 ± 0.14 |
4.0 ± 0.32 |
24, 34, 55 and 57–62
|
Pyrene |
4.2 ± 0.12 |
4.4 ± 0.31 |
24, 34, 55 and 57–64
|
Benzo(a)pyrene |
4.7 ± 0.22 |
5.4 ± 0.43 |
24, 34, 55, 57–60, 62, 64 and 65
|
PCB 28 |
4.6 ± 0.46 |
5.2 ± 0.30 |
55, 58 and 66–71
|
PCB 52 |
5.0 ± 0.58 |
5.6 ± 0.22 |
55, 58, 65, 66 and 68–72
|
Stability of dosing conditions during repeated usage of the dosing system
To assess the mixture composition in the exposure medium during repeated use of the dosing vials, PDMS-cast dosing vials were spiked with 9 chemicals, and the amount of chemical in the silicone was monitored after using the dosing vials twice consecutively for two 72 h bioassays (3). The concentrations of individual chemicals in the exposure medium decreased by 1–37% after the first 72 h test and by 10–55% after the second 72 h test, relative to the initial mass in the silicone (before use but after equilibration of 1 mL Milli-Q water, Fig. 1(2)), respectively. The concentrations of the PCBs decreased the least, with 10% (0.2 μg, PCB 28) up to 31% (0.7 μg, PCB 153) after the second exposure, which indicates that the chemical composition is rather stable for more hydrophobic chemicals (log
Kow 5.9–7.3) after multiple usage. The concentrations of γ-HCH and the PAHs decreased by 41–55% (0.3–1.6 μg). Surprisingly, pyrene decreased the most, by 55% between before the exposure and after the second exposure, which indicates that hydrophobicity alone cannot explain the buffer capacity of the silicone polymer.
At the start of the exposure, 4-monochlorophenol (log
Kow 2.4) was below the detection limit, while 2,6-dichlorophenol and 3,5-dichlorophenol (Kow 2.8–3.6) were detected at <10% of the loaded mass. After the first 72 h of exposure, none of the phenolic chemicals could be detected, including triclosan (log
Kow 4.76). This observation could be explained by a fairly low sorptive capacity of the PDMS for some of these chemicals due to their relatively polar properties, which also cause them to partition more readily into the water phase compared to γ-HCH, PCBs and PAHs. To compensate for the losses of phenolic chemicals, a larger ratio of the mass of PDMS versus water volume might be needed, as was also observed by Ribbenstedt et al.25 These results support the applicability domain of the silicone-based system for semi-hydrophobic to hydrophobic chemicals and the possibility to retain a mixture composition within ∼50% for such chemicals.
Effects of the exposure on cell viability
As illustrated by the low mortality in the controls (0–1.6%, min–max), the passive dosing system did not exert measurable stress to the test algae. Moreover, the test system provided stable test conditions, as evidenced by the negligible between-run variability in the controls and no significant difference in the control mortality between the experimental runs (ANOVA; p > 0.4); Table ESI S3.† In the algae exposed to 1 to 200-fold concentrated chemicals (approximate exposure levels of e.g. PAH15 and PCB7 are 0.25–50 μg L−1 and 0.22–44 ng L−1, respectively), the proportion of dead cells increased significantly and in a dose–response manner (Fig. 4 and Table ESI S4†). This illustrates that the test system provided adequate means for testing the exposure effects of mixtures of chemicals. In the 1
:
1 treatment, the fraction of dead cells nearly doubled compared to that in the controls. However, due to the small group size and a relative high variability in the 1
:
1 treatment and controls, this effect was non-significant (unpaired t-test; p > 0.178; Table ESI S5†). To detect a significant increase over the background mortality at such low numbers of dead cells in the population, at least 10 replicates would be necessary as estimated by the power test (power > 80%, α = 0.05). This, however, would also imply higher experimental costs. Still, this method might be more cost-efficient and provide more realistic information on effects of complex environmental mixtures of chemicals compared to other approaches, where single chemicals are tested, and the data are combined by modeling.
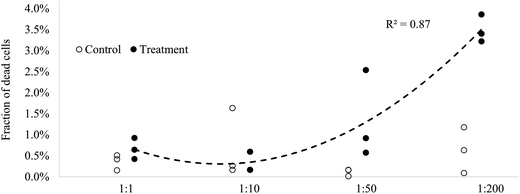 |
| Fig. 4 Fraction of dead algal cells in the exposure treatments and the controls (n = 3). Trend line (dashed) fitted to the exposure treatments. NB: two replicates overlap in the 1 : 10 treatment and in the 1 : 50 control. Due to the fact that samples from treatments 1 : 10, 1 : 50 and 1 : 200 were lost upon the first exposure, the experiment was repeated re-using the same dosing vials. As it was found that the concentration of chemicals in the exposure vials declined after the first use (Fig. 3), the actual exposure levels in all treatments but 1 : 1 are likely overestimated (by 55% at the most). Thus, the fraction of dead cells in the treatments 1 : 10, 1 : 50 and 1 : 200 might be underestimated relative to the exposure levels reported in this study. | |
A number of studies have investigated responses to environmental mixtures of chemicals, by using either passive dosing methods or solvent spiking. As the methodologies differ regarding the test organisms and experimental setup, the results are not directly comparable. Nevertheless, the relatively low proportion of dead cells (0.7%) at the ambient exposure level agrees with the growth-related responses reported in the literature. For instance, Claessens et al.33 did not observe any adverse effects on growth inhibition, using the marine diatom Phaeodactylum tricornutum (Bohlin) exposed by passive dosing to an environmental chemical mixture from seawater from the Belgian coast. Furthermore, Everaert et al.73 used the same methodology as Claessens et al.33 to investigate the toxicity of an environmental mixture of HOCs sampled in the same area and concluded that chemical exposure at ambient levels explained only 1.1% of the variability in the growth dynamic of P. tricornutum that was largely controlled by environmental factors, such as nutrient availability, temperature and light. In another study from the open Atlantic Ocean, the toxicity of chemical mixtures sampled from ocean water on marine cyanobacteria was investigated by solvent spiking. No effect was observed at ambient water concentrations of the mixture, but a negative effect was observed when the extract was concentrated to 20–40 fold the ambient concentrations.74 Several studies have investigated toxicity of chemical mixtures extracted from sediment in passive dosing systems. However, comparisons are difficult as those studies focus on the toxicity of specific chemical fractions (as in Bandow et al.28), use solvent extraction to extract the chemicals from the sediment (while we used passive sampling which only captures the bioavailable fraction), or they recreate the toxicity of spiked sediment by the use of passive dosing (as in Perron et al.75). Bandow et al. solvent-extracted chemicals from sediment and fractionated the extract into 18 groups. While some fractions were non-toxic, others resulted in >50% growth inhibition of the green algae Scenedesmus vacuolatus. The toxicity of chemical mixtures has also been investigated by using solvent extracts from passive samplers that had equilibrated with sediment. For instance, in Li et al.21 the non-specific cytotoxicity of chemical mixtures in sediment was more accurately assessed by chemicals attained by passive sampling compared to exhaustive extraction. Bräunig et al.76 retrieved chemicals from sediments by passive sampling or solvent extraction. Sediment toxicity was attributed to the sorption capacity of sediments, as strongly sorbed chemicals may be unavailable for partitioning and therefore not available to sediment dwelling organisms.
Implications
Ecosystems and humans are constantly exposed to complex mixtures of innumerable chemicals. The strategy of combining passive sampling and passive dosing opens up ways for addressing the risk of the bioavailable fraction of many unknown semi-hydrophobic to hydrophobic chemicals present in the environment, as well as the joint effects of the mixture. This method can be used for testing a wide range of exposure scenarios, at different exposure levels. Thus, the passive dosing system was demonstrated to be applicable for both short-term (this study) and chronic bioassays.25 To improve the sensitivity of the 72 h bioassay with algal Live/Dead assay and to assess the detectability of toxic action at low exposure concentrations, a higher number of replicates should be used. The method is cost-effective and offers a more realistic complement to chemical analyses of artificial mixtures. Expanding the applicability domain of the method and thereby including more polar chemicals in the mixture would increase its usefulness also in water. To capture a wider range of chemicals, polymers with different properties could be used in parallel, which needs further research. Furthermore, the sensitivity of the bioassay may be improved by using endpoints targeting different modes of toxic action.
Conflicts of interest
The authors declare no competing financial interest.
Acknowledgements
This research was funded by the Swedish research council (FORMAS Grant no. #2012–1211). We thank Karin Ek, Department of Environmental Science and Analytical Chemistry, Stockholm University, for laboratory assistance with the algal culture.
References
- A. Jönsson, Ö. Gustafsson, J. Axelman and H. Sundberg, Environ. Sci. Technol., 2003, 37, 245–255 CrossRef.
- L. Nizzetto, M. Macleod, K. Borgå, A. Cabrerizo, J. Dachs, A. D. Guardo, D. Ghirardello, K. M. Hansen, A. Jarvis, A. Lindroth, B. Ludwig, D. Monteith, J. A. Perlinger, M. Scheringer, L. Schwendenmann, K. T. Semple, L. Y. Wick, G. Zhang and K. C. Jones, Environ. Sci. Technol., 2010, 44, 6526–6531 CrossRef CAS PubMed.
-
A. Kortenkamp, T. Backhaus and M. Faust, State of the Art Review of Mixture Toxicity. Report to the Commission of the European Union, 2009 Search PubMed.
- V. Dupraz, N. Coquillé, D. Ménard, R. Sussarellu, L. Haugarreau and S. Stachowski-Haberkorn, Chemosphere, 2016, 151, 241–252 CrossRef CAS PubMed.
- C. Bizarro, M. Eide, D. J. Hitchcock, A. Goksøyr and M. Ortiz-Zarragoitia, Aquat. Toxicol., 2016, 177, 395–404 CrossRef CAS PubMed.
- E. Silva, N. Rajapakse and A. Kortenkamp, Environ. Sci. Technol., 2002, 36, 1751–1756 CrossRef CAS PubMed.
- T. Backhaus and M. Faust, Environ. Sci. Technol., 2012, 46, 2564–2573 CrossRef CAS PubMed.
- T. Backhaus, R. Altenburger, Å. Arrhenius, H. Blanck, M. Faust, A. Finizio, P. Gramatica, M. Grote, M. Junghans, W. Meyer, M. Pavan, T. Porsbring, M. Scholze, R. Todeschini, M. Vighi, H. Walter and L. Horst Grimme, Cont. Shelf Res., 2003, 23, 1757–1769 CrossRef.
- H. Walter, F. Consolaro, P. Gramatica, M. Scholze and R. Altenburger, Ecotoxicology, 2002, 11, 299–310 CrossRef CAS PubMed.
- J. Y. M. Tang, S. McCarty, E. Glenn, P. A. Neale, M. S. J. Warne and B. I. Escher, Water Res., 2013, 47, 3300–3314 CrossRef CAS PubMed.
- E. L. M. Vermeirssen, J. Hollender, N. Bramaz, J. van der Voet and B. I. Escher, Environ. Toxicol. Chem., 2010, 29, 2575–2582 CrossRef CAS PubMed.
- B. V. Rutishauser, M. Pesonen, B. I. Escher, G. E. Ackermann, H. R. Aerni, M. J. F. Suter and R. I. Eggen, Environ. Toxicol. Chem., 2004, 23, 857–864 CrossRef CAS PubMed.
- F. D. Leusch, C. De Jager, Y. Levi, R. Lim, L. Puijker, F. Sacher, L. A. Tremblay, V. S. Wilson and H. F. Chapman, Environ. Sci. Technol., 2010, 44, 3853–3860 CrossRef CAS PubMed.
- B. I. Escher, R. I. Eggen, U. Schreiber, Z. Schreiber, E. Vye, B. Wisner and R. P. Schwarzenbach, Environ. Sci. Technol., 2002, 36, 1971–1979 CrossRef CAS PubMed.
- D. Mackay, J. A. Arnot, F. Wania and R. E. Bailey, Integr. Environ. Assess. Manage., 2011, 7, 248–255 CrossRef CAS PubMed.
- P. Mayer, J. Tolls, J. L. Hermens and D. Mackay, Environ. Sci. Technol., 2003, 37, 184A–191A CrossRef PubMed.
- C. L. Arthur and J. Pawliszyn, Anal. Chem., 1990, 62, 2145–2148 CrossRef CAS.
- A. Jahnke, P. Mayer and M. S. McLachlan, Environ. Sci. Technol., 2012, 46, 10114–10122 CrossRef CAS PubMed.
- K. Mäenpää, M. T. Leppänen, F. Reichenberg, K. Figueiredo and P. Mayer, Environ. Sci. Technol., 2011, 45, 1041–1047 CrossRef PubMed.
- G. Cornelissen, K. Wiberg, D. Broman, H. P. H. Arp, Y. Persson, K. Sundqvist and P. Jonsson, Environ. Sci. Technol., 2008, 42, 8733–8739 CrossRef CAS PubMed.
- J. Y. Li, J. Y. Tang, L. Jin and B. I. Escher, Environ. Toxicol. Chem., 2013, 32, 2888–2896 CrossRef CAS PubMed.
- M. Shaw, A. Negri, K. Fabricius and J. F. Mueller, Aquat. Toxicol., 2009, 95, 108–116 CrossRef CAS PubMed.
- P. Mayer, J. Wernsing, J. Tolls, P. G. J. de Maagd and D. T. Sijm, Environ. Sci. Technol., 1999, 33, 2284–2290 CrossRef CAS.
- K. E. Smith, N. Dom, R. Blust and P. Mayer, Aquat. Toxicol., 2010, 98, 15–24 CrossRef CAS PubMed.
- A. Ribbenstedt, L. Mustajärvi, M. Breitholtz, E. Gorokhova, P. Mayer and A. Sobek, Environ. Toxicol. Chem., 2017, 35, 1254–1260 CrossRef PubMed.
- K. E. Smith, G. J. Oostingh and P. Mayer, Chem. Res. Toxicol., 2009, 23, 55–65 CrossRef PubMed.
- A. Gerofke, P. Komp and M. S. McLachlan, Water Res., 2004, 38, 3411–3419 CrossRef CAS PubMed.
- N. Bandow, R. Altenburger, U. Lübcke-von Varel, A. Paschke, G. Streck and W. Brack, Environ. Sci. Technol., 2009, 43, 3891–3896 CrossRef CAS PubMed.
- K. E. Smith, S. N. Schmidt, N. Dom, R. Blust, M. Holmstrup and P. Mayer, Environ. Sci. Technol., 2013, 47, 2026–2033 CrossRef CAS PubMed.
- N. I. Kramer, F. J. M. Busser, M. T. T. Oosterwijk, K. Schirmer, B. I. Escher and J. L. M. Hermens, Chem. Res. Toxicol., 2010, 23, 1806–1814 CrossRef CAS PubMed.
- E. Rojo-Nieto, K. E. Smith, J. Perales and P. Mayer, Aquat. Toxicol., 2012, 120, 27–34 CrossRef PubMed.
- A. Jahnke, P. Mayer, S. Schäfer, G. Witt, N. Haase and B. I. Escher, Environ. Sci. Technol., 2016, 50, 5424–5431 CrossRef CAS PubMed.
- M. Claessens, E. Monteyne, K. Wille, L. Vanhaecke, P. Roose and C. R. Janssen, Mar. Pollut. Bull., 2015, 93, 9–19 CrossRef CAS PubMed.
- J.-H. Kwon, T. Wuethrich, P. Mayer and B. I. Escher, Anal. Chem., 2007, 79, 6816–6822 CrossRef CAS PubMed.
- E. Gorokhova, L. Mattsson and A. M. Sundstrom, J. Microbiol. Methods, 2012, 89, 216–221 CrossRef CAS PubMed.
- F. Reichenberg, F. Smedes, J. A. Jonsson and P. Mayer, Chem. Cent. J., 2008, 2, 8 CrossRef PubMed.
- K. Mäenpää, M. T. Leppänen, K. Figueiredo, P. Mayer, D. Gilbert, A. Jahnke, C. Gil-Allué, J. Akkanen, I. Nybom and S. Herve, Environ. Toxicol. Chem., 2015, 34, 2463–2474 CrossRef PubMed.
- H. Birch, V. Gouliarmou, H.-C. Holten Lützhøft, P. S. Mikkelsen and P. Mayer, Anal. Chem., 2010, 82, 1142–1146 CrossRef CAS PubMed.
- Y. Zebühr, C. Näf, C. Bandh, D. Broman, R. Ishaq and H. Pettersen, Chemosphere, 1993, 27, 1211–1219 CrossRef.
- M. Mandalakis, Y. Zebühr and Ö. Gustafsson, J. Chromatogr. A, 2004, 1041, 111–117 CrossRef CAS PubMed.
- L. Mustajärvi, E. Eek, G. Cornelissen, A.-K. Eriksson-Wilkund, E. Undeman and A. Sobek, Environ. Pollut., 2017, 231, 854–862 CrossRef PubMed.
- R. S. Brown, P. Akhtar, J. Åkerman, L. Hampel, I. S. Kozin, L. A. Villerius and H. J. Klamer, Environ. Sci. Technol., 2001, 35, 4097–4102 CrossRef CAS PubMed.
- Y. Kiparissis, P. Akhtar, P. V. Hodson and R. S. Brown, Environ. Sci. Technol., 2003, 37, 2262–2266 CrossRef CAS PubMed.
- D. Gilbert, G. Witt, F. Smedes and P. Mayer, Anal. Chem., 2016, 88, 5818–5826 CrossRef CAS PubMed.
-
P. G. Wells, K. Lee and C. Blaise, Microscale testing in aquatic toxicology: advances, techniques, and practice, CRC Press, 1997 Search PubMed.
- S. Stachowski-Haberkorn, M. Jérôme, J. Rouxel, C. Khelifi, M. Rincé and T. Burgeot, Aquat. Toxicol., 2013, 140, 380–388 CrossRef PubMed.
- A. Macken, M. Giltrap, B. Foley, E. McGovern, B. McHugh and M. Davoren, Environ. Pollut., 2008, 153, 627–637 CrossRef CAS PubMed.
- R. R. L. Guillard and J. H. Ryther, Can. J. Microbiol., 1962, 8, 229–239 CrossRef CAS PubMed.
-
OECD, Guideline for testing of chemicals. No. 201, freshwater algae and cyanobacteria, growth inhibition test, Organisation for Economic Co-operation and Development, Paris, 2011 Search PubMed.
- A. Gerofke, P. Kömp and M. S. McLachlan, Environ. Toxicol. Chem., 2005, 24, 2908–2917 CrossRef CAS PubMed.
- K. Mäenpää, M. T. Leppänen, F. Reichenberg, K. Figueiredo and P. Mayer, Environ. Sci. Technol., 2011, 45, 1041–1047 CrossRef PubMed.
- K. E. Smith, M. B. Heringa, M. Uytewaal and P. Mayer, Mutat. Res., Genet. Toxicol. Environ. Mutagen., 2013, 750, 12–18 CrossRef CAS PubMed.
- T. P. Rusina, F. Smedes, J. Klanova, K. Booij and I. Holoubek, Chemosphere, 2007, 68, 1344–1351 CrossRef CAS PubMed.
- F. Stibany, S. N. Schmidt, A. Schäffer and P. Mayer, Chemosphere, 2017, 167, 551–558 CrossRef CAS PubMed.
- F. Smedes, R. W. Geertsma, T. v. d. Zande and K. Booij, Environ. Sci. Technol., 2009, 43, 7047–7054 CrossRef CAS PubMed.
- E. L. DiFilippo and R. P. Eganhouse, Environ. Sci. Technol., 2010, 44, 6917–6925 CrossRef CAS PubMed.
- J. Poerschmann, T. Górecki and F.-D. Kopinke, Environ. Sci. Technol., 2000, 34, 3824–3830 CrossRef CAS.
- A. Paschke and P. Popp, J. Chromatogr. A, 2003, 999, 35–42 CrossRef CAS PubMed.
- B. Shurmer and J. Pawliszyn, Anal. Chem., 2000, 72, 3660–3664 CrossRef CAS PubMed.
- M. T. Jonker and B. Muijs, Chemosphere, 2010, 80, 223–227 CrossRef CAS PubMed.
- G. Ouyang, J. Cai, X. Zhang, H. Li and J. Pawliszyn, J. Sep. Sci., 2008, 31, 1167–1172 CrossRef CAS PubMed.
- G. Witt, G. A. Liehr, D. Borck and P. Mayer, Chemosphere, 2009, 74, 522–529 CrossRef CAS PubMed.
- J. J. H. Haftka, J. R. Parsons, H. A. J. Govers and J. J. Ortega-Calvo, Environ. Toxicol. Chem., 2008, 27, 1526–1532 CrossRef CAS PubMed.
- T. L. ter Laak, M. Durjava, J. Struijs and J. L. Hermens, Environ. Sci. Technol., 2005, 39, 3736–3742 CrossRef CAS PubMed.
- K. A. Maruya, E. Y. Zeng, D. Tsukada and S. M. Bay, Environ. Toxicol. Chem., 2009, 28, 733–740 CrossRef CAS PubMed.
- T. L. ter Laak, F. J. Busser and J. L. Hermens, Anal. Chem., 2008, 80, 3859–3866 CrossRef CAS PubMed.
- Z.-Y. Yang, E. Y. Zeng, H. Xia, J.-Z. Wang, B.-X. Mai and K. A. Maruya, J. Chromatogr. A, 2006, 1116, 240–247 CrossRef CAS PubMed.
- E. Baltussen, P. Sandra, F. David, H.-G. Janssen and C. Cramers, Anal. Chem., 1999, 71, 5213–5216 CrossRef CAS.
- M. K. Durjava, T. L. Ter Laak, J. L. Hermens and J. Struijs, Chemosphere, 2007, 67, 990–997 CrossRef CAS PubMed.
- M.-K. Hsieh, C.-T. Fu and S.-c. Wu, Environ. Sci. Technol., 2011, 45, 7785–7791 CrossRef CAS PubMed.
- R. P. Eganhouse, J. Chromatogr. A, 2016, 1438, 226–235 CrossRef CAS PubMed.
- A. R. Schneider, A. Paolicchi and J. E. Baker, Int. J. Environ. Anal. Chem., 2006, 86, 789–803 CrossRef CAS.
- G. Everaert, F. De Laender, M. Claessens, J. Baert, E. Monteyne, P. Roose, P. L. M. Goethals and C. R. Janssen, Mar. Pollut. Bull., 2016, 102, 58–64 CrossRef CAS PubMed.
- P. Echeveste, J. Dachs, N. Berrojalbiz and S. Agustí, Chemosphere, 2010, 81, 161–168 CrossRef CAS PubMed.
- M. M. Perron, R. M. Burgess, K. T. Ho, M. C. Pelletier, C. L. Friedman, M. G. Cantwell and J. P. Shine, Environ. Toxicol. Chem., 2009, 28, 749–758 CAS.
- J. Bräunig, J. Y. M. Tang, M. S. J. Warne and B. I. Escher, Chemosphere, 2016, 156, 181–190 CrossRef PubMed.
Footnote |
† Electronic supplementary information (ESI) available. See DOI: 10.1039/c7em00228a |
|
This journal is © The Royal Society of Chemistry 2017 |