Environmental trends of metals and PCDD/Fs around a cement plant after alternative fuel implementation: human health risk assessment†
Received
14th March 2017
, Accepted 11th May 2017
First published on 31st May 2017
Abstract
This study aimed at evaluating the potential impact of a cement plant after 4 years of the employment of alternative fuel. In June 2015, concentrations of PCDD/Fs and metals were determined in soils, vegetation and air in order to measure potential changes with respect to previous surveys before (July 2011) and after (June 2013) the employment of alternative fuel. Risks to human health were also assessed. In soils, metal levels were similar to those observed in June 2013 (p > 0.05). In comparison with July 2011, the increment was only statistically significant for As and Cd (p < 0.05). A notable increase in levels of PCDD/Fs was noted when current levels in soils (1.14 ng WHO-TEQ per kg) were compared with those observed in July 2011 (0.37 ng WHO-TEQ per kg) (p > 0.05) and June 2013 (0.41 ng WHO-TEQ per kg) (p < 0.05). This increase was mainly caused by the increase in PCDD/F levels at one sampling site, which showed the heterogeneity of PCDD/F levels in soils, possibly as a result of different point emissions over the years. On the other hand, temporal trends in levels of metals and PCDD/Fs in vegetation showed a clear decrease, which indicated that the particle fraction of these pollutants would potentially be removed from leaf surfaces by wash-off. In air, levels were similar to those found in previous surveys. The results of PCA showed that the change in fuel had not affected the environmental profiles of metals and PCDD/Fs around the cement plant. The exposure of the population living in the surroundings of the plant was measured and it was shown that diet was the major contributor for both metals and PCDD/Fs, with percentages of over 97%, the only exceptions being As and Pb, for which dietary intake accounted for 43% and 71% of the total exposure, respectively. Environmental non-cancer and cancer risks were within the limits considered as acceptable by international standards.
Environmental impact
The valorization of wastes as alternative fuels in cement plants implies a wide range of benefits such as reductions in CO2 emissions, brings savings of non-renewable resources, and at the same time provides a solution to an emerging problem, namely, the management of wastes. However, this practice still generates concern regarding possible health effects caused by emissions, in particular when populated areas are located nearby. This study aimed at evaluating the potential impact of a cement plant after 4 years of the employment of alternative fuels. PCDD/Fs and metals were determined in soils, vegetation and air, and the environmental changes with respect to previous studies were evaluated. Risks to human health were also assessed.
|
Introduction
The valorization of wastes as alternative fuels in cement plants has increased quickly in most European countries since the 1990s. In 2012, the average rate of substitution in the EU was 36%.1 However, wide differences exist depending on the country; for example, in Greece substitution rates are very low, on average between 6% and 7%, whereas in Germany they reach 62%.1 Currently, in Catalonia wastes (mainly sewage sludge, agricultural wastes and refuse-derived fuel (RDF)) are being used as supplementary fuels in different cement plants with an average rate of substitution of around 20%.2–4 This practice implies a wide range of benefits such as reductions in carbon dioxide (CO2) emissions and the consequent mitigation of climate change, brings savings of non-renewable resources, and at the same time provides a solution to an emerging problem in our society, namely, the management of wastes. On the other hand, as has been reported by the German GTZ and Holcim Group Support5 and subsequently summarized by Chinyama,6 important considerations have to be taken into account when co-processing: (1) co-processing must respect the waste hierarchy established by the Waste Framework Directive (2008/98EC); (2) additional emissions and negative impacts on human health must be avoided; (3) the quality of the cement must remain unaffected; (4) companies that co-process must be qualified; and finally (5) the implementation of co-processing must consider national circumstances (e.g. legislation).
Although the use of wastes as alternative fuels in cement plants is currently a widespread practice,7–9 it still generates concern regarding the possible health effects caused by emissions, in particular when populated areas are located nearby. In addition to CO2 and nitrogen oxides (NOx), which are the major pollutants emitted by cement plants, polychlorinated dibenzo-p-dioxins and dibenzofurans (PCDD/Fs) and metals are among the most hazardous pollutants that are also potentially released. The latter chemicals are characterized by their persistence, toxicity and capacity for bioaccumulation.10 Accordingly, individuals and stakeholder organizations are often skeptical about fuel substitution owing to environmental concerns.11 Monitoring studies are a useful tool for evaluating the environmental impact of pollutant sources and assessing their potential risks.
A cement plant located between the towns of Pallejà and Sant Vicenç dels Horts and very close to the latter town, in the metropolitan area of Barcelona (Catalonia, Spain), has been operating since the 1920s. Currently, the annual production of the facility is around 200
000–1
300
000 tonnes of clinker. In 2010, a new production line (Line 6) came into operation. Line 6 replaces the old lines and is equipped with the Best Available Techniques (BATs). The main characteristics of the new facility have previously been reported by Valderrama et al.12 By the end of 2011, dried municipal sewage sludge started to be used as an alternative fuel, and the current percentage fuel substitution provides around 25% of the total thermal energy required. In brief, during the clinker production process sewage sludge is introduced into the precalciner (after being dried at low temperatures) together with the preheated milled raw materials. Afterwards, the material is discharged into the kiln, where temperatures of up to >1800 °C are attained to produce the clinker.12
In 2011, an environmental program was initiated in order to determine potential changes in the emissions of the plant. PCDD/Fs and metals were analyzed in soil, vegetation and air samples before (January and July 2011) and after (January 2012 and June 2013) the employment of alternative fuel.2 The present study aimed at evaluating the potential impact of the cement plant emissions after 4 years of the employment of alternative fuel. Environmental concentrations of PCDD/Fs and metals were determined in soil, vegetation and air, and potential environmental changes with respect to previous studies were evaluated in terms not only of levels but also of profiles. Finally, the risks to human health were assessed.
Materials and methods
Sampling
In June 2015, seven samples each of soil and vegetation and four samples of air (a total of 18 samples) were collected at the same sampling points reported in previous surveys.2 The criteria used for the selection of sampling points and the sampling methodology were described by Rovira et al.2Fig. 1 shows an illustration of the sampling points, which were located at different distances and in different directions from the cement plant. They were selected according to the results of an air dispersion model and considering the location of population nuclei. One of the sampling points was selected to represent an area of heavy traffic not influenced by the plant (no. 6: B + T), whereas another represented a background site without traffic (no. 7: B). The average temperature during the sampling period was 23.0 °C, with a maximum and minimum of 28.1 °C and 17.8 °C, respectively. No precipitation occurred during the sampling period. However, during the week prior to the sampling slight precipitation was registered (8.6 mm).
 |
| Fig. 1 Points used for sampling soil, vegetation and air. Blue: sampling points for soil, vegetation and air; green: sampling points for soil and vegetation; red: cement plant; B + T: background + traffic; B: background. | |
Air samples were collected using two high-volume active samplers (Tisch Environmental), namely, TE-1000-PUF and TE-6070-DV, for the determination of PCDD/Fs and trace elements (adsorbed on PM10), respectively. The collection of each sample took 48 hours (with volumes within the range of 580–620 m3) and 24 hours (with volumes within the range of 1500–1700 m3) for PCDD/Fs and particulate matter, respectively. For PCDD/Fs, the particulate and gas phases were collected separately with a quartz fiber filter (QFF) and polyurethane foam (PUF), respectively. The PCDD/Fs samples were kept frozen at −20 °C until analysis. In addition, a QFF was used for sampling PM10. Soil samples (approximately 500 g), which consisted of four subsamples from within an area of 25 m2, were collected from the upper 5 cm of the ground, dried at room temperature and sieved through a 2 mm mesh screen. Finally, samples of around 150 g vegetation (Piptatherum L.) were obtained by cutting the plants at 5 cm above the ground and dried at room temperature.
Metal analysis
The methodology used for the pretreatment and analysis of soil, vegetation and air samples was as previously described.2,13 In brief, 0.5 g samples (soil and vegetation) were treated in hermetic Teflon bombs with 5 mL HNO3 (65% Suprapur, E. Merck, Darmstadt, Germany) for 8 h at room temperature and an additional 8 h at 80 °C. In addition, QFFs were treated with a mixture of 2 mL HNO3 (65% Suprapur, E. Merck) and 3 mL HF (37.5%, Panreac SA) in hermetic Teflon bombs for 8 h at room temperature and an additional 8 h at 80 °C. After being cooled, the extracts were filtered and made up to 25 mL with ultrapure water. They were kept frozen at −20 °C until further analysis. The concentrations of arsenic (As), cadmium (Cd), cobalt (Co), chromium (Cr), copper (Cu), mercury (Hg), manganese (Mn), nickel (Ni), lead (Pb), antimony (Sb), tin (Sn), thallium (Tl), vanadium (V), and zinc (Zn) were determined (in the three environmental monitors) by means of inductively coupled plasma mass spectrometry (ICP-MS, PerkinElmer Elan 6000). The accuracy of the methods was checked by means of laboratory reagent blanks and control samples, as well as reference materials of loamy clay (Resource Technology Corporation) for soil and air and spinach leaves (National Institute of Standards and Technology) for vegetation. The recovery percentages of the reference materials ranged between 69% and 104%, 78% and 108%, and 94% and 110% for soil, vegetation and air, respectively.
PCDD/Fs analysis
The concentrations of PCDD/Fs in soil and vegetation samples were determined by high-resolution gas chromatography coupled to high-resolution mass spectrometry (HRGC/HRMS) in accordance with US EPA method 1613. In addition, PCDD/F levels in air were also determined by HRGC/HRMS according to the German VDI method 3499 (German VDI 2003). Conventional procedures of quality assurance/quality control (QA/QC) were employed. Blank, replicate and reference samples were analyzed for every batch of samples, which showed that the method displayed good repeatability. The recovery percentages were 83–115%, 15–85% and 54–96% for soil, vegetation and air samples, respectively (the recoveries for each individual congener are listed in Table 1 of the ESI†). In the case of vegetation, for several samples the recoveries of some of the 13C-labeled dioxin standards were on the low side. According to the isotope dilution principle used in HRMS analyses, losses of “native” and “labeled” dioxins during sample preparation occur in the same ratio and therefore losses are automatically corrected for. Therefore, the results for the concentrations were considered to be valid. In this case, the lower recoveries were caused by the matrix itself; the vegetation samples contained a large amount of “waxy/oily” material, which is extremely hard to remove during sample clean-up. This forced us to employ a multi-step clean-up process, which caused the low recoveries. The first step used a multi-layer silica column. In the second clean-up stage, which used gel permeation chromatography with BioBeads SX-3 material, the extract was eluted with a mixture of cyclohexane/ethyl acetate in order to remove large interfering molecules. Finally, the extract was eluted on a basic alumina column in order to separate PCDD/Fs from other interfering components by applying different eluent solutions to the column. The detection limits ranged between 0.020 and 0.10 ng kg−1 in soil and vegetation and between 0.001 and 0.016 pg m−3 in air, according to the specific PCDD/F congener.
Human health risks
The human exposure, as well as the associated health risks, around the facility was assessed using the current concentrations of metals and PCDD/Fs in air and soil samples after 4 years of the partial substitution of traditional by alternative fuel (sewage sludge). The exposure of the local population was estimated by considering three different routes: soil ingestion, dermal contact and air inhalation. The numerical expressions and parameters used to evaluate exposure and the non-carcinogenic and carcinogenic risks (CRs) from ingestion, dermal contact and inhalation were as previously described.14 In addition, the exposure to metals and PCDD/Fs from dietary intake was determined from recent studies performed in the area under study15–17 and compared with the environmental exposure. The characterization of non-carcinogenic risks involves the calculation of the hazard quotient (HQ), which is defined as the ratio between the predicted exposure and the reference dose (RfD). In contrast, CRs were estimated by multiplying the predicted exposure by the respective slope factor (SF). For the estimation of dermal absorption, the dermal RfD was calculated by multiplying the respective oral RfD by the gastrointestinal absorption factor, whereas dermal SFs were obtained by dividing the respective oral factor by the same gastrointestinal absorption factor.18 For inhalation, risks were calculated on the basis of inhalation dosimetry methodology, which is based on the exposure concentration (EC).19 Toxicological parameters for risk calculations were obtained from the US Risk Assessment Information System web page (RAIS, available at: http://rais.ornl.gov/).
Statistics
Statistical analysis was carried out by means of the statistical software package SPSS 16.0. For calculations, concentrations below the detection limit (LOD) were assumed to be equal to one-half of the respective limit (ND = 1/2 LOD). The Levene test was employed to confirm the equality of variances. Either ANOVA or the Mann–Whitney U test was used, depending on whether or not the data followed a parametric distribution. The level of significance was set at a probability of lower than 0.05 (p < 0.05).
Results and discussion
Environmental levels
The results for environmental levels after 4 years of the employment of sewage sludge as an alternative fuel in the cement plant (June 2015) are presented here and compared with those obtained in previous surveys performed at the same time of year before (July 2011) and after (June 2013) the employment of an alternative fuel.2
Soil
Table 1 shows the levels of metals and PCDD/Fs in soil samples collected around the cement plant in 2015. The metals that had the lowest concentrations were Hg, of which the levels ranged from 0.02 to 0.06 mg kg−1, and Sb, of which the concentrations varied from <0.01 to 0.27 mg kg−1. The levels of Cd, Tl and Sn ranged from 0.14 to 0.37 mg kg−1, from 0.09 to 0.68 mg kg−1 and from 0.84 to 2.37 mg kg−1, respectively. Arsenic, Co and Ni displayed values of between 2.02 and 26.5 mg kg−1, 2.03 and 28.6 mg kg−1 and 5.24 and 55.9 mg kg−1, respectively. The following elements were more abundant: Cr (range: 5.90–27.3 mg kg−1), Cu (range: 18.2–53.6 mg kg−1), Pb (range: 9.24–37.5 mg kg−1) and V (range: 9.07–76.8 mg kg−1). Zinc and Mn were the most abundant metals, with values that ranged between 82.5 and 190 mg kg−1 and 307 and 678 mg kg−1, respectively. These concentrations of metals did not exceed in any case the limits established by the Catalan Agency of Residues for different soil uses.20 In general terms, no clear trend could be observed regarding the levels of metals and the distance from the facility at the three points located in Sant Vicenç dels Horts (SVdH1, SVdH2 and SVdH3). The concentrations of PCDD/Fs in soil samples ranged between 0.29 ng WHO-TEQ per kg (at sampling point no. 2) and 2.66 ng WHO-TEQ per kg (at sampling point no. 4). The concentrations of PCDD/Fs at the control sites were 1.60 and 0.49 ng WHO-TEQ per kg at sampling point no. 6 (background influenced by traffic (B + T)) and sampling point no. 7 (considered as background (B)), respectively.
Table 1 Concentrations of metals (mg kg−1) and PCDD/Fs (ng WHO-TEQ per kg) in soils around the cement plant in June 2015
|
No. 1 |
No. 2 |
No. 3 |
No. 4 |
No. 5 |
No. 6 |
No. 7 |
SVdH1 |
SVdH2 |
Pallejà |
Molins |
SVdH3 |
B + T |
B |
As |
13.6 |
7.63 |
26.5 |
5.90 |
13.7 |
11.2 |
2.02 |
Cd |
0.34 |
0.25 |
0.22 |
0.24 |
0.22 |
0.37 |
0.14 |
Co |
10.7 |
4.94 |
28.6 |
7.17 |
7.76 |
6.55 |
2.03 |
Cr |
24.2 |
16.8 |
27.3 |
20.5 |
18.1 |
25.0 |
5.90 |
Cu |
40.0 |
27.7 |
34.8 |
53.6 |
18.2 |
51.6 |
23.3 |
Hg |
0.03 |
0.02 |
0.04 |
0.06 |
0.03 |
0.04 |
0.04 |
Mn |
495 |
577 |
307 |
678 |
555 |
637 |
476 |
Ni |
24.4 |
12.9 |
55.9 |
11.3 |
20.1 |
21.5 |
5.24 |
Pb |
30.5 |
21.7 |
16.1 |
33.5 |
37.5 |
29.5 |
9.24 |
Sb |
0.07 |
0.05 |
0.25 |
0.27 |
<0.01 |
0.03 |
0.14 |
Sn |
1.03 |
1.19 |
1.33 |
2.37 |
1.40 |
1.75 |
0.84 |
Tl |
0.58 |
0.16 |
0.68 |
0.19 |
0.19 |
0.09 |
0.13 |
V |
33.3 |
20.2 |
76.8 |
36.7 |
26.8 |
19.8 |
9.07 |
Zn |
118 |
88.0 |
96.5 |
190 |
82.5 |
108 |
131 |
PCDD/Fs |
1.03 |
0.29 |
0.99 |
2.66 |
0.73 |
1.60 |
0.49 |
Table 2 shows the mean concentrations of metals and PCDD/Fs found at the sampling points under the influence of the cement plant and the background sites with and without the influence of traffic, namely, (B + T) and (B), respectively, in the current (June 2015) and previous surveys before (July 2011) and after (June 2013) the employment of alternative fuel (Fig. 1 and 4, ESI†). In addition, the temporal trends are presented. The current concentrations of Cd and Cu were significantly higher (p < 0.05) at the sampling point considered as background affected by traffic (B + T) than those found at the sampling points near the cement plant. In fact, Cd is associated with the engine emissions of vehicles, whereas Cu is one of the metals associated with brake wear.21 For the other metals, although in general concentrations were higher at the point influenced by traffic (B + T) than those around the cement plant, the differences were not statistically significant (p > 0.05). In addition, the concentrations of As, Cd, Co, Cr, Cu, Pb, Sn, Tl and V were higher around the cement plant and at the background plus traffic site (B + T) than at the background (B) site (p < 0.05). For most metals, a decrease was noted when current levels were compared with the results found in June 2013, although the decrease was not statistically significant in any case (p > 0.05). Besides, most metals displayed increases in concentration with respect to the levels found in July 2011. However, the increase was only statistically significant for As and Cd (p < 0.05). Regarding PCDD/Fs in soils, a notable increase was noted when current levels (1.14 ng WHO-TEQ per kg) were compared with those determined in July 2011 (p > 0.05) (0.37 ng WHO-TEQ per kg) and June 2013 (p < 0.05) (0.41 ng WHO-TEQ per kg). The total rise in levels of PCDD/Fs was mainly caused by the increase at the Molins de Rei sampling site (no. 4) (2.66 ng WHO-TEQ per kg). This increase may reflect the heterogeneity of the levels of PCDD/Fs in the area, which was probably caused by different point emission sources over the years. Notwithstanding, mean concentrations at the background site affected by traffic (B + T) were still higher (2.52 ng WHO-TEQ per kg) than those in the area directly affected by the plant (1.14 ng WHO-TEQ per kg), which highlights the importance of traffic as an important contributor to environmental PCDD/Fs. Finally, PCDD/F levels around the plant were notably higher than the mean values found at the background (B) site (0.45 ng WHO-TEQ per kg; p < 0.05). In any case, PCDD/F levels in soils were well below the most restrictive level established in Europe, which is set at 5 ng TEQ per kg for agricultural soils in Germany.22
Table 2 Mean levels of metals (mg kg−1) and PCDD/Fs (ng WHO-TEQ per kg) in soil samples collected in the current survey (June 2015) performed 4 years after the employment of alternative fuels and those obtained in previous surveys before (July 2011) and after (June 2013) the change in fuels. Samples were collected in the area under the potential influence of the facility, as well as in two background areas (with traffic (B + T) and no traffic impact (B))
|
Traditional fuel |
Alternative fuel |
Background |
% variation |
July 2011 |
June 2013 |
June 2015 (current levels) |
B + T |
B |
July 2011–June 2015 |
June 2013–June 2015 |
Concentrations in background areas (B + T and B) were calculated as mean values of the three studies. a,bIndicate statistical differences between 2015 levels and background areas (B + T and B) (p < 0.05). *Indicate statistical differences between years of collection (p < 0.05). |
As |
4.86 ± 7.61 |
10.7 ± 11.1 |
13.5 ± 8.08a |
10.9 ± 1.67a |
1.68 ± 0.30b |
64* |
−11 |
Cd |
0.10 ± 0.06 |
0.34 ± 0.44 |
0.25 ± 0.05a |
0.61 ± 0.35b |
0.06 ± 0.06c |
62* |
0 |
Co |
7.63 ± 4.02 |
11.6 ± 5.63 |
11.8 ± 9.60a |
8.02 ± 1.6a |
3.03 ± 0.88b |
35 |
−15 |
Cr |
16.8 ± 9.40 |
21.2 ± 9.43 |
21.4 ± 4.34a |
47.9 ± 38.4a |
6.90 ± 0.96b |
21 |
−5 |
Cu |
25.6 ± 18.8 |
42.6 ± 23.0 |
34.9 ± 13.3a |
56.9 ± 29.3b |
10.1 ± 11.4c |
27 |
−5 |
Hg |
<0.10 |
<0.10 |
0.04 ± 0.01 |
<0.10 |
<0.10 |
— |
— |
Mn |
415 ± 140 |
656 ± 80.4 |
522 ± 137 |
722 ± 231 |
449 ± 96.1 |
20 |
3 |
Ni |
11.1 ± 14.8 |
14.5 ± 18.0 |
24.9 ± 18.1 |
26.1 ± 6.34 |
6.71 ± 1.28 |
56 |
−12 |
Pb |
14.6 ± 7.65 |
25.1 ± 10.8 |
27.9 ± 8.77a |
62.8 ± 47.6a |
5.06 ± 3.62b |
47 |
−6 |
Sb |
<0.10 |
<0.10 |
0.16 ± 0.12 |
0.14 ± 0.17 |
0.08 ± 0.05 |
69 |
−24 |
Sn |
0.82 ± 0.44 |
1.11 ± 0.60 |
1.46 ± 0.53a |
1.89 ± 1.69a |
0.35 ± 0.43b |
44 |
−5 |
Tl |
0.28 ± 0.21 |
0.45 ± 0.42 |
0.36 ± 0.25a |
0.15 ± 0.05a |
0.09 ± 0.03b |
21 |
−14 |
V |
46.7 ± 24.2 |
63.9 ± 20.7 |
38.8 ± 22.2a |
30.0 ± 9.14a |
11.0 ± 2.00b |
−21 |
−12 |
Zn |
84.2 ± 14.9 |
111 ± 0.29 |
115 ± 44.0 |
205 ± 133 |
55.4 ± 65.6 |
27 |
−6 |
PCDD/Fs |
0.37 ± 0.33 |
0.41 ± 0.29 |
1.14 ± 0.90 |
2.52 ± 2.25 |
0.45 ± 0.21 |
67 |
64* |
Vegetation
The metal and PCDD/F levels measured in vegetation samples collected around the cement plant are summarized in Table 3. Table 4 shows the concentrations found in the previous surveys, as well as the temporal trends (Fig. 2 and 5, ESI†). Levels of Hg were detected in six out of the seven samples, with a range of <0.01 to 0.02 mg kg−1. These concentrations were considerably lower than the detection limits achieved in the previous studies (<0.10 mg kg−1). Similarly, Sb was detected at sampling points no. 4 and no. 5, with a concentration of 0.02 mg kg−1 in both cases. Likewise, Tl was only detected at sampling point no. 2, with a concentration of 0.02 mg kg−1. Levels of Cd (range: <0.01–0.03 mg kg−1) and As (range: 0.04–0.19 mg kg−1) were also low. Concentrations of Sn ranged between 0.79 mg kg−1 at the background sampling point affected by traffic (B + T; no. 6) and 1.55 mg kg−1 at sampling point no. 4, whereas Co levels ranged between 0.02 mg kg−1 at sampling point no. 4 and 0.15 mg kg−1 at sampling point no. 5. Vanadium, Cr and Ni also exhibited similar concentrations at all the sampling sites, which ranged from 0.06 to 0.17 mg kg−1, 0.35 to 0.81 mg kg−1 and 0.31 to 0.83 mg kg−1, respectively. Higher levels were found for Cu (range: 2.24–8.84 mg kg−1), Mn (range: 14.9–58.6 mg kg−1) and Zn (range: 12.7–37.2 mg kg−1). No clear trend could be found between the levels of metals and the distance from the cement plant (SVdH1, SVdH2 and SVdH3). Statistically higher levels of Cr, Pb, V and Zn were found at the background site influenced by traffic (B + T) when compared with those found around the cement plant and at the background site (p < 0.05). In addition, concentrations of Cr were higher at the background site (B) than in the area influenced by the cement plant (p < 0.05). These elements are tracers of different sources: V is associated with fuel oil combustion, Pb and Zn with traffic, and Cr with pigment production.17,23 General decreases were noted when current levels of metals were compared with those determined before and after the employment of alternative fuel in 2011 and 2013, respectively, most of which were significant (p < 0.05). This fact could be associated with the precipitation registered during the week prior to the sampling, because: (1) metals deposited on the vegetation may have been washed off and (2) vegetation may have grown, which would have had the effect of diluting the levels present in the vegetation samples. The current mean PCDD/Fs concentration in vegetation was 0.13 ng WHO-TEQ per kg, with maximum and minimum concentrations of 0.18 and 0.08 ng WHO-TEQ per kg, respectively. Temporal trends showed a clear decrease in 2015, in contrast with the increase observed in soils, which confirmed the possibility that rain may have washed PCDD/Fs off the surface of the vegetation samples, as well as diluting them. Although PCDD/Fs are hydrophobic substances, particulate and associated particle-bound PCDD/Fs would adhere to leaf surfaces and would potentially be removed by wash-off.24 The decrease in current PCDD/F levels in vegetation was significant in comparison with the 2013 levels (p < 0.05). PCDD/F levels recorded near the plant were statistically similar to those found at the background site affected by traffic (B + T) and the background (B) site (p > 0.05). The PCDD/F levels determined here in vegetation samples, all of which were collected in summer, were lower than those found in the same area in the winter months.2 Seasonality in PCDD/F levels is associated with seasonal variations in the dispersion of PCDD/Fs in ambient air, as well as in their sources (such as home heating, fireplaces, etc.).25
Table 3 Concentrations of metals (mg kg−1) and PCDD/Fs (ng WHO-TEQ per kg) in vegetation around the cement plant in June 2015
|
No. 1 |
No. 2 |
No. 3 |
No. 4 |
No. 5 |
No. 6 |
No. 7 |
SVdH1 |
SVdH2 |
Pallejà |
Molins |
SVdH3 |
B + T |
B |
As |
0.05 |
0.07 |
0.19 |
0.04 |
0.07 |
0.17 |
0.07 |
Cd |
<0.01 |
0.01 |
0.02 |
0.03 |
0.02 |
<0.01 |
<0.01 |
Co |
0.05 |
0.04 |
0.04 |
0.02 |
0.15 |
0.06 |
0.02 |
Cr |
0.72 |
0.57 |
0.81 |
0.35 |
0.58 |
0.39 |
0.79 |
Cu |
3.61 |
4.43 |
5.09 |
3.88 |
8.84 |
8.49 |
2.24 |
Hg |
0.02 |
0.02 |
0.02 |
<0.01 |
0.01 |
0.02 |
0.02 |
Mn |
26.5 |
23.5 |
35.1 |
14.9 |
58.6 |
43.8 |
45.6 |
Ni |
0.57 |
0.65 |
0.71 |
0.31 |
0.83 |
0.66 |
0.62 |
Pb |
0.21 |
0.28 |
0.43 |
0.31 |
0.39 |
0.31 |
0.08 |
Sb |
<0.01 |
<0.01 |
<0.01 |
0.02 |
0.02 |
<0.01 |
<0.01 |
Sn |
1.10 |
1.13 |
1.31 |
1.55 |
1.01 |
0.79 |
1.05 |
Tl |
<0.01 |
0.02 |
<0.01 |
<0.01 |
<0.01 |
<0.01 |
<0.01 |
V |
0.11 |
0.11 |
0.17 |
0.06 |
0.14 |
0.07 |
0.06 |
Zn |
15.0 |
18.9 |
18.5 |
13.7 |
37.2 |
32.5 |
12.7 |
PCDD/Fs |
0.09 |
0.16 |
0.08 |
0.18 |
0.14 |
0.11 |
0.08 |
Table 4 Levels of metals (mg kg−1) and PCDD/Fs (ng WHO-TEQ per kg) in vegetation samples collected in the current survey (June 2015) performed 4 years after the employment of alternative fuels and those obtained in previous surveys before (July 2011) and after (June 2013) the change in fuels. Samples were collected in the area under the potential influence of the facility, as well as in two background areas (with traffic (B + T) and no traffic impact (B))
|
Traditional fuel |
Alternative fuel |
Background |
% variation |
July 2011 |
June 2013 |
June 2015 (current levels) |
B + T |
B |
July 2011–June 2015 |
June 2013–June 2015 |
Concentrations in background areas (B + T and B) were calculated as mean values of the three studies. a,b Indicate statistical differences between 2015 levels and background areas (B + T and B) (p < 0.05). *Indicates statistical differences between years of collection (p < 0.05). |
As |
0.16 ± 0.16 |
0.16 ± 0.14 |
0.08 ± 0.06 |
0.17 ± 0.01 |
0.06 ± 0.01 |
−92 |
−93 |
Cd |
0.02 ± 0.02 |
0.09 ± 0.07 |
0.02 ± 0.01 |
0.15 ± 0.13 |
0.02 ± 0.02 |
−23 |
−444* |
Co |
0.07 ± 0.05 |
0.08 ± 0.01 |
0.06 ± 0.05 |
0.09 ± 0.03 |
0.02 ± 0.02 |
−26 |
−31 |
Cr |
1.56 ± 0.21 |
1.85 ± 0.61 |
0.61 ± 0.18a |
1.80 ± 1.24b |
1.21 ± 0.44b |
−157* |
−205* |
Cu |
5.28 ± 0.99 |
9.33 ± 3.56 |
5.17 ± 2.13 |
8.50 ± 1.58 |
4.65 ± 2.86 |
−2 |
−80 |
Hg |
<0.10 |
<0.10 |
<0.10 |
<0.10 |
<0.10 |
— |
— |
Mn |
38.1 ± 20.5 |
41.1 ± 15.1 |
31.7 ± 16.7 |
32.7 ± 12.5 |
32.5 ± 11.6 |
−20 |
−30* |
Ni |
0.90 ± 0.37 |
1.28 ± 0.46 |
0.61 ± 0.19 |
1.04 ± 0.38 |
0.84 ± 0.46 |
−46 |
−109* |
Pb |
0.48 ± 0.44 |
0.65 ± 0.27 |
0.32 ± 0.09a |
3.27 ± 2.56b |
0.34 ± 0.28a |
−47 |
−100 |
Sb |
0.06 ± 0.03 |
0.07 ± 0.04 |
<0.01 |
0.08 ± 0.09 |
0.04 ± 0.02 |
−457* |
−519* |
Sn |
0.18 ± 0.12 |
0.27 ± 0.21 |
1.22 ± 0.21 |
0.60 ± 0.18 |
0.50 ± 0.48 |
85* |
78* |
Tl |
0.03 ± 0.03 |
<0.03 |
<0.03 |
<0.03 |
<0.03 |
−286 |
— |
V |
0.54 ± 0.16 |
0.21 ± 0.12 |
0.12 ± 0.04a |
0.36 ± 0.26b |
0.26 ± 0.17a |
−363* |
−85 |
Zn |
25.9 ± 8.99 |
34.9 ± 17.3 |
20.6 ± 9.50a |
43.8 ± 10.7b |
17.6 ± 4.36a |
−26 |
−69* |
PCDD/Fs |
0.39 ± 0.37 |
0.30 ± 0.12 |
0.13 ± 0.04 |
0.24 ± 0.28 |
0.18 ± 0.10 |
−199 |
−255* |
Air
Table 5 shows the concentrations of metals associated with PM10 particles and PCDD/Fs in air in the surroundings of the cement plant. In addition, Table 6 shows the current mean levels together with those found in the previous studies carried out before and after the employment of alternative fuel in July 2011 and June 2013, respectively, and the trends are shown in Fig. 3 and 6 in the ESI.† The PM10 levels were 32.50, 29.20, 27.10 and 21.90 μg m−3 at sampling points no. 1, no. 2, no. 3 and no. 4, respectively. Mercury and Tl exhibited the lowest concentrations, which ranged from <0.02 to 0.05 and from 0.01 to 0.05 ng m−3, respectively. The concentrations of As, Cd and Co were between 0.35 and 1.37 ng m−3, 0.16 and 0.24 ng m−3, and 0.13 and 0.20 ng m−3, respectively. Chromium, Ni, Sb, Sn and V displayed the following ranges: 0.76–4.20 ng m−3, 2.09–3.86 ng m−3, 0.67–0.76 ng m−3, 1.51–2.47 ng m−3 and 3.61–6.65 ng m−3, respectively, whereas Pb levels ranged between 5.70 and 10.8 ng m−3. The principal elements in PM10 were Zn (range: 66.7–330 ng m−3), Cu (range: 19.5–76.5 ng m−3) and Mn (range: 10.3–15.3 ng m−3). Although temporal fluctuations were observed for most elements, they were not statistically significant in any case (p > 0.05). The concentrations of metals in ambient PM10 were similar to those found in similar areas.10,26,27 PCDD/F levels in air in June 2015 ranged between 0.006 and 0.010 pg WHO-TEQ per m3, with a mean value of 0.008 pg WHO-TEQ per m3. The current PCDD/F levels were similar to those found in July 2011 and June 2013, which were 0.009 and 0.018 pg WHO-TEQ per m3, respectively (p > 0.05). In the same way as was noted for vegetation, the concentrations of PCDD/Fs in air were lower than those found in surveys conducted in winter in the same area.2
Table 5 Concentrations of metals (ng m−3), PM10 (μg m−3) and PCDD/Fs (pg WHO-TEQ per m3) in ambient air around the cement plant in June 2015
|
No. 1 |
No. 2 |
No. 3 |
No. 4 |
As |
1.37 |
0.53 |
0.59 |
0.35 |
Cd |
0.24 |
0.17 |
0.16 |
0.17 |
Co |
0.20 |
0.19 |
0.15 |
0.13 |
Cr |
4.20 |
0.97 |
0.76 |
1.12 |
Cu |
27.5 |
19.5 |
76.5 |
74.7 |
Hg |
0.02 |
<0.02 |
0.05 |
0.04 |
Mn |
10.3 |
14.4 |
13.6 |
15.3 |
Ni |
3.86 |
2.41 |
3.09 |
2.09 |
Pb |
10.8 |
6.24 |
8.57 |
5.70 |
Sb |
0.71 |
0.67 |
0.76 |
0.68 |
Sn |
1.51 |
1.91 |
2.26 |
2.47 |
Tl |
0.04 |
0.02 |
0.03 |
0.01 |
V |
5.54 |
3.63 |
6.65 |
3.61 |
Zn |
330 |
86.4 |
110 |
66.7 |
PM10 |
32.50 |
29.20 |
27.10 |
21.90 |
PCDD/Fs |
0.006 |
0.005 |
0.010 |
0.010 |
Table 6 Levels of metals (ng m−3), PM10 (mg m−3) and PCDD/Fs (pg WHO-TEQ per m3) in air samples collected around the cement plant in the current survey 4 years after alternative fuel implementation (June 2015) together with those obtained in previous surveys before (July 2011) and after (June 2013) fuel implementation
|
Traditional fuel |
Alternative fuel |
July 2011 |
June 2013 |
June 2015 (current levels) |
As |
1.27 ± 1.06 |
0.37 ± 0.14 |
0.49 ± 0.13 |
Cd |
0.13 ± 0.12 |
0.12 ± 0.09 |
0.19 ± 0.04 |
Co |
0.09 ± 0.07 |
0.16 ± 0.09 |
0.17 ± 0.03 |
Cr |
3.29 ± 1.02 |
3.69 ± 0.42 |
1.76 ± 1.63 |
Cu |
25.6 ± 9.59 |
37.5 ± 31.2 |
49.5 ± 30.3 |
Hg |
<0.18 |
<0.18 |
<0.18 |
Mn |
8.26 ± 3.53 |
6.91 ± 2.51 |
13.39 ± 2.16 |
Ni |
0.50 ± 0.83 |
3.29 ± 1.87 |
2.86 ± 0.78 |
Pb |
8.93 ± 3.07 |
5.79 ± 3.82 |
7.83 ± 2.35 |
Sb |
0.59 ± 0.29 |
0.95 ± 0.29 |
0.71 ± 0.04 |
Sn |
2.03 ± 0.58 |
1.76 ± 1.33 |
2.04 ± 0.42 |
Tl |
0.03 ± 0.02 |
<0.05 |
<0.05 |
V |
5.20 ± 2.49 |
4.36 ± 2.06 |
4.86 ± 1.50 |
Zn |
147 ± 62.0 |
117 ± 148 |
148 ± 122 |
PM10 |
19.9 ± 12.5 |
15.1 ± 5.08 |
27.6 ± 4.45 |
PCDD/Fs |
0.009 ± 0.001 |
0.018 ± 0.011 |
0.008 ± 0.003 |
Principal component analysis
The objective of principal component analysis (PCA) is to derive a few new principal components (PCs) that are linear combinations of the original variables, which provide a description of the data structure with the minimum loss of information. In this study, PCA was executed with a double objective: (a) to compare the profiles of the samples collected around the cement plant (samples no. 1 to no. 5) with the profiles of those collected outside the direct influence of the cement plant (samples no. 6 (B + T) and no. 7 (B), respectively) and (b) to evaluate potential changes in the profiles of environmental pollutants caused by the recent change in fuel in the cement plant by comparing the samples collected before (July 2011) and after (June 2015 and June 2013) the employment of alternative fuel.
Although we obtained measurements of metal and PCDD/F levels in soil, vegetation and air, we only used vegetation samples to investigate pollutant patterns by means of PCA. Soil reflects the long-term accumulation of pollutants, and it is therefore possible that it has been influenced by different significant sources over the years. On the other hand, air samples indicate daily concentrations. Meteorological variations and point emissions can dramatically alter concentrations of pollutants in air during short time periods. Therefore, we would need a long series of air samples to accurately represent the patterns in ambient air. Vegetation has been widely used as a short-term indicator that represents the levels of ambient pollution deposited on plant leaves over a few months.28 Accordingly, this indicator was selected as the most suitable for examining potential different patterns of pollutants in different areas (influenced by the cement plant and background controls) and periods (before and after the employment of alternative fuel). Because of the relatively small number of vegetation samples (N = 21), we decided to perform separate PCAs for metals and PCDD/Fs. In addition, pollutants with concentrations below the detection limit in more than 40% of the samples were excluded. In both PCAs, the final number of variables (N = 11) was less than the number of samples (N = 21), as is recommended when performing a PCA. For metals, the PCA provided a three-dimensional model with three principal components (PC1, PC2 and PC3), which explained 44.5%, 14.4% and 13.9% of the variance, respectively. The score plots of PC1 vs. PC2 and PC1 vs. PC3 are shown in Fig. 2. The score plot of PC1 vs. PC2 shows that the samples collected in July 2011 and June 2013 are clustered together whereas the samples collected in June 2015 appear separately, which indicates a difference in patterns between periods. In addition, the samples from point no. 6 (B + T) collected in July 2011 and June 2013 and point no. 4 collected in June 2013 exhibit a different pattern. However, in the plot of PC1 vs. PC3 almost all the samples appear clustered together regardless of the sampling period. Considering that PC2 and PC3 explained similar percentages of the variance, the results indicate that the differences among the metal profiles were minor irrespective of whether they were collected before or after the employment of alternative fuel. In addition, the B samples appear together with those collected near the cement plant, which indicates that the impact of the plant in terms of metal emissions is low. For PCDD/Fs, the PCA provided a two-dimensional model with two principal components (PC1 and PC2), which explained 67.5% and 15.8% of the variance, respectively. Again, the score plot (Fig. 3) does not show differences between areas or periods, and most samples are clustered together with the exception of the samples from points no. 4, 2 and 6 collected in July 2011. These results demonstrate that neither the cement plant nor the change in fuel significantly affected the PCDD/F profiles. Diffuse sources, which also affected the background areas (B + T and B), seem to have been responsible for the short-term burden of PCDD/Fs.
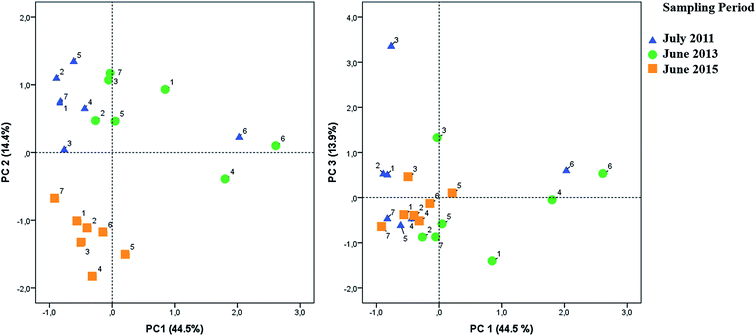 |
| Fig. 2 Score plots of PC1 vs. PC2 and PC1 vs. PC3 for metals in vegetation samples. | |
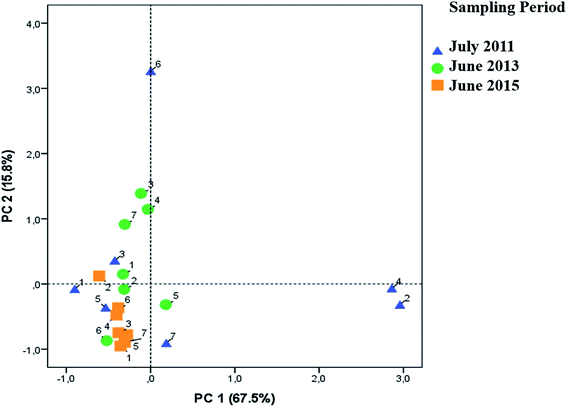 |
| Fig. 3 Score plot of PC1 vs. PC2 for PCDD/Fs in vegetation samples. | |
Human health risks
The environmental concentrations determined after 4 years of the employment of alternative fuel were used to calculate the environmental exposure and risks for the population living in the surroundings of the cement plant. Table 7 shows the exposure from soil ingestion, dermal contact and air inhalation. The exposures due to inhalation of Hg and Tl and ingestion of Sb could not be calculated, because their concentrations were found to be below the detection limit in air and soil, respectively. For metals, the maximum exposures from soil ingestion and dermal contact were found to occur for Mn (7.94 × 10−4 and 2.82 × 10−5 mg per kg per day, respectively), whereas for inhalation the maximum exposure was found for Zn (4.06 × 10−5 mg per kg per day). On the other hand, the minimum exposures from soil ingestion and dermal contact were found to occur for Hg (6.25 × 10−8 and 2.22 × 10−9 mg per kg per day, respectively), whereas for inhalation the minimum exposure was found for Co (4.58 × 10−8 mg per kg per day). In general, the ingestion of soil was the main pathway of environmental exposure to metals (range: 7.94 × 10−4 to 6.25 × 10−8 mg per kg per day), with the sole exception being As, for which dermal exposure (2.48 × 10−5 mg per kg per day) was dominant. The minimum exposure, which depended on the metal, occurred via inhalation for As, Co, Cr, Mn, Ni and V (range: 1.41 × 10−8 to 6.75 × 10−6 mg per kg per day) and via dermal contact for Cd, Cu, Pb, Sn and Zn (range: 1.41 × 10−8 to 3.67 × 10−6 mg per kg per day). For PCDD/Fs, the exposures were 1.78 × 10−6, 1.89 × 10−6 and 2.10 × 10−6 ng WHO-TEQ per kg day for soil ingestion, dermal contact and inhalation, respectively. It is well established that dietary intake is the major source of exposure to metals and PCDD/Fs for the general population.29,30 We used data for the dietary intake of PCDD/Fs and metals that were representative of the Catalan population and were obtained in recent studies15–17 to establish the percentages of environmental and dietary exposure for the population living in the surroundings of the cement plant (Fig. 4). It can be seen that for most metals diet contributes more than 97% of the total exposure, the only exceptions being As and Pb, for which dietary intake accounted for 43% and 71% of the total exposure, respectively. It has to be noted that in the assessment of dietary exposure to As only the inorganic fraction was examined, because that is the most toxic, although the total content of As in food is much higher.15 For the environmental assessment, however, total As was determined. In the same way, for PCDD/Fs diet was the major contributor to the total exposure (97.5%).
Table 7 Exposure to metals (mg per kg per day) and PCDD/Fs (ng WHO-TEQ per kg day) for individuals living in the area under the influence of the cement plant after 4 years of the employment of alternative fuel (June 2015)
|
Soil ingestion |
Dermal contact |
Inhalation |
Total environmental |
Dietary |
Data obtained from Perelló et al.15
Data obtained from Perelló et al.16
Data obtained from Perelló et al.17 NC: not calculated because the environmental levels were below the detection limit. NA: not analyzed.
|
As |
2.33 × 10−5 |
2.48 × 10−5 |
1.95 × 10−7 |
4.83 × 10−5 |
3.71 × 10−5a |
Cd |
3.98 × 10−7 |
1.41 × 10−8 |
5.09 × 10−8 |
4.63 × 10−7 |
1.24 × 10−4a |
Co |
2.12 × 10−5 |
7.52 × 10−7 |
4.58 × 10−8 |
2.20 × 10−5 |
NA |
Cr |
3.52 × 10−5 |
1.25 × 10−6 |
4.83 × 10−7 |
3.69 × 10−5 |
1.08 × 10−2b |
Cu |
5.72 × 10−5 |
2.03 × 10−6 |
1.36 × 10−5 |
7.28 × 10−5 |
1.49 × 10−2b |
Hg |
6.25 × 10−8 |
2.22 × 10−9 |
NC |
6.47 × 10−8 |
1.43 × 10−4a |
Mn |
7.94 × 10−4 |
2.82 × 10−5 |
3.67 × 10−6 |
8.26 × 10−4 |
3.86 × 10−2b |
Ni |
4.36 × 10−5 |
1.55 × 10−6 |
7.84 × 10−7 |
4.59 × 10−5 |
5.77 × 10−3b |
Pb |
4.59 × 10−5 |
1.63 × 10−6 |
2.15 × 10−6 |
4.97 × 10−5 |
1.20 × 10−4a |
Sb |
NC |
NC |
1.93 × 10−7 |
1.93 × 10−7 |
1.31 × 10−4b |
Sn |
2.39 × 10−6 |
8.50 × 10−8 |
5.59 × 10−7 |
3.04 × 10−6 |
NA |
Tl |
6.40 × 10−7 |
2.27 × 10−8 |
NC |
6.63 × 10−7 |
NA |
V |
6.78 × 10−5 |
2.41 × 10−6 |
1.33 × 10−6 |
7.15 × 10−5 |
NA |
Zn |
1.90 × 10−4 |
6.75 × 10−6 |
4.06 × 10−5 |
2.37 × 10−4 |
2.84 × 10−1b |
PCDD/Fs |
1.78 × 10−6 |
1.89 × 10−6 |
2.10 × 10−6 |
5.77 × 10−6 |
2.30 × 10−4c |
 |
| Fig. 4 Percentages of environmental and dietary exposure to metals and PCDD/Fs in the area under the influence of the cement plant after 4 years of the employment of alternative fuel (June 2015). | |
The environmental non-carcinogenic risks for metals and PCDD/Fs, as represented by the hazard quotient (HQ), which is calculated as the ratio between the predicted exposure and the corresponding reference dose, are shown in Fig. 5. It can be observed that in all cases the HQs were well below unity, which is considered to be the safety threshold. The maximum and minimum HQ were found for Mn (3.19 × 10−1) and Sn (4.13 × 10−6), respectively. Table 8 shows the environmental carcinogenic risks. Cancer risks of less than 10 × 10−5 are considered to be acceptable, according to Spanish legislation.31 The current risks were below that threshold for all carcinogenic metals with the exception of As (3.34 × 10−5) and Cr (1.32 × 10−5). However, they were within the range of 10 × 10−6 to 10 × 10−4, which can be considered to be acceptable on the basis of the highly variable characteristics of each individual.32 In addition, it has to be noted that in our study the total As and Cr contents were determined in environmental samples, although the carcinogenic forms are inorganic As and Cr(VI). The environmental chemistry of chromium is complex. The speciation of Cr into Cr(III) and Cr(VI) depends on a variety of environmental parameters (pH, concentration, and the ligands available in the matrix). Naturally occurring Cr(VI) is rare, as it is readily reduced by organic matter in the environment; however, industrial sources may release Cr(VI) compounds. The behavior of both Cr(VI) and Cr(III) and the interconversion between them must be understood when attempting to characterize the bioaccessibility and risks associated with chromium.33 However, when no data are available regarding speciation, as was the case in this study, it is normally assumed that Cr(VI) comprises one-sixth of total Cr.2 This assumption, however, may lead to an overestimation of the risks, as has been pointed out in other studies with similar results.34 In addition, Catalan soils are enriched in As owing to their geological origin, and background and reference values are set at 10.0 and 49.5 mg kg−1, respectively.35 As a consequence, in Catalonia carcinogenic risks due to environmental exposure to As are commonly found to lie at the upper end of the probability range.36,37 Regarding PCDD/Fs, the total carcinogenic risk was 3.24 × 10−7. In fact, current risks to human health due to environmental exposure to metals and PCDD/Fs were similar to those determined when traditional fuel was used in the cement plant, as well as those found in similar urban and industrial areas in Catalonia.2,36,38,39
 |
| Fig. 5 Non-carcinogenic risks for metals and PCDD/Fs (hazard quotients) for individuals living in the area under the influence of the cement plant after 4 years of the employment of alternative fuel (June 2015). | |
Table 8 Carcinogenic risks for individuals living in the area under the influence of the cement plant after 4 years of the employment of alternative fuel (June 2015)
|
Ingestion |
Dermal |
Inhalation |
Total |
As |
1.50 × 10−5 |
1.60 × 10−5 |
2.43 × 10−6 |
3.34 × 10−5 |
Cd |
NC |
NC |
1.38 × 10−7 |
1.38 × 10−7 |
Co |
NC |
NC |
6.18 × 10−7 |
6.18 × 10−7 |
Cr(VI) |
1.26 × 10−6 |
1.79 × 10−6 |
1.02 × 10−5 |
1.32 × 10−5 |
Ni |
NC |
NC |
3.06 × 10−7 |
3.06 × 10−7 |
Pb |
1.67 × 10−7 |
5.94 × 10−9 |
3.86 × 10−8 |
2.12 × 10−7 |
PCDD/Fs |
9.90 × 10−8 |
1.05 × 10−7 |
1.20 × 10−7 |
3.24 × 10−7 |
Conclusions
Environmental levels of metals and PCDD/Fs were determined in the surroundings of a cement plant after 4 years of the employment of sewage sludge as an alternative fuel. In general, levels of metals in soils were similar to those determined in previous surveys before and after the employment of alternative fuel, whereas in vegetation a decrease was observed. PCDD/F levels increased in soils, which was especially due to an increase observed at a specific point; this indicates the heterogeneity of concentrations in soils, which may be caused by specific point emissions. On the other hand, metal and PCDD/F levels decreased in vegetation, which indicated a possible wash-off effect due to rain in the week prior to sampling. Metal and PCDD/F levels in air were similar to those found in previous samplings. PCA of vegetation samples did not show significant differences between the profiles of metals and PCDD/Fs before and after the employment of alternative fuel, or between samples collected around the cement plant and those collected at background sites. These results show that the cement plant and the change in fuel have not affected the environmental profiles of metals and PCDD/Fs. Estimates of exposure to metals and PCDD/Fs indicated that diet was the major contributor, the sole exception being As. However, it has to be noted that calculations of dietary exposure only considered the inorganic fraction of As. Environmental non-cancer and cancer risks were within the limits considered to be acceptable by international standards.
Acknowledgements
The present study was financially supported by Cementos Molins Industrial S.A.U., Barcelona, Spain.
References
-
ECOFYS, Market opportunities for use of alternative fuels in cement plants across the EU, Assessment of drivers and barriers for increased fossil fuel substitution in three EU member states: Greece, Poland and Germany, CEMBUREAU, 2016 Search PubMed.
- J. Rovira, M. Nadal, M. Schuhmacher and J. L. Domingo, Sci. Total Environ., 2014, 485–486, 121–129 CrossRef CAS PubMed.
- J. Rovira, M. Nadal, M. Schuhmacher and J. L. Domingo, Arch. Environ. Contam. Toxicol., 2016, 71, 473–484 CrossRef CAS PubMed.
- J. Rovira, M. Mari, M. Nadal, M. Schuhmacher and J. L. Domingo, Sci. Total Environ., 2010, 408, 5372–5380 CrossRef CAS PubMed.
-
G. T. Z. Holcim, Guidelines on co-processing Waste Materials in Cement Production, 2006 Search PubMed.
-
M. P. M. Chinyama, Alternative Fuels in Cement Manufacturing, in Alternative Fuel, ed. M. Manzanera, 2011, ch. 11, DOI:10.5772/22319.
- G. Richards and I. E. Agranovski, J. Hazard. Mater., 2017, 323, 698–709 CrossRef CAS PubMed.
- C. A. Tsiliyannis, Energy, 2016, 113, 1202–1218 CrossRef CAS.
- A. Rahman, M. G. Rasul, M. M. K. Khan and S. Sharma, Procedia Eng., 2013, 56, 393–400 CrossRef CAS.
- M. Mari, M. Nadal, M. Schuhmacher and J. L. Domingo, Environ. Int., 2009, 35, 1034–1039 CrossRef CAS PubMed.
-
Pembina Institute and Environmental Defence, Alternative Fuel Use in Cement Manufacturing Implications, opportunities and barriers in Ontario, White paper for Workshop on Alternative Fuels in Cement Kilns, Canada, 2014 Search PubMed.
- C. Valderrama, R. Granados, J. L. Cortina, C. M. Gasol, M. Guillem and A. Josa, J. Cleaner Prod., 2013, 51, 205–213 CrossRef CAS.
- L. Vilavert, M. Nadal, M. Mari, M. Schuhmacher and J. Domingo, J. Environ. Sci. Health, Part A: Toxic/Hazard. Subst. Environ. Eng., 2009, 44, 1343–1352 CrossRef CAS PubMed.
- L. Vilavert, M. Nadal, M. Schuhmacher and J. L. Domingo, Sci. Total Environ., 2014, 466–467, 733–740 CrossRef CAS PubMed.
- G. Perelló, J. M. Llobet, J. Gómez-Catalán, V. Castell, F. Centrich, M. Nadal and J. L. Domingo, Biol. Trace Elem. Res., 2014, 162, 26–37 CrossRef PubMed.
- G. Perelló, E. Vicente, V. Castell, J. M. Llobet, M. Nadal and J. Domingo, Food Addit. Contam., Part A, 2015, 32, 748–755 Search PubMed.
- G. Perelló, J. Gómez-Catalán, V. Castell, J. M. Llobet and J. L. Domingo, Food Chem. Toxicol., 2012, 50, 399–408 CrossRef PubMed.
-
USEPA, Risk assessment guidance for Superfund. Volume I. Human health evaluation manual, Office of Superfund Remediation and Technology Innovation, Washington, D. C. 20450, 1989, EPA/540/1-89/002 Search PubMed.
-
USEPA, Risk assessment guidance for superfund volume I: Human Health Evaluation Manual (Part F, Supplemental guidance for inhalation risk assessment), United States Environmental Protection Agency, 2009 Search PubMed.
- ARC, Valors dels NGR per metalls i metalloides i protecció de la salut humana aplicables a Catalunya, Agència de Residus de Catalunya, available from: http://residus.gencat.cat/ca/ambits_dactuacio/sols_contaminats/nivells_generics_de_referencia_ngr/valors_dels_ngr_per_metalls_i_metal_loides_i_proteccio_salut/2009.
- J. Pey, N. Pérez, S. Castillo, M. Viana, T. Moreno, M. Pandolfi, J. M. López-Sebastián, A. Alastuey and X. Querol, Atmos. Res., 2009, 94, 422–435 CrossRef CAS.
- Y. Y. Deng, L. J. Jia, K. Li, Z. Y. Rong and H. W. Yin, Bull. Environ. Contam. Toxicol., 2011, 86, 65–70 CrossRef CAS PubMed.
- C. A. Belis, F. Karagulian, B. R. Larsen and P. K. Hopke, Atmos. Environ., 2013, 69, 94–108 CrossRef CAS.
- M. Schuhmacher, K. C. Jones and J. L. Domingo, Environ. Pollut., 2006, 142, 143–150 CrossRef CAS PubMed.
- Y. Tian, Z. Nie, S. Tian, F. Liu, J. He, Y. Yang, X. Wang, Q. Die, Y. Fang and Q. Huang, Environ. Sci. Pollut. Res., 2015, 22, 13243–13250 CrossRef CAS PubMed.
- M. Nadal, M. Mari, M. Schuhmacher and J. L. Domingo, Environ. Int., 2009, 35, 227–235 CrossRef CAS PubMed.
- J. Rovira, M. Mari, M. Schuhmacher, M. Nadal and J. L. Domingo, Arch. Environ. Contam. Toxicol., 2011, 60, 372–384 CrossRef CAS PubMed.
- M. Schuhmacher, A. Bocio, M. C. Agramunt, J. L. Domingo and H. A. M. de Kok, Chemosphere, 2002, 48, 209–217 CrossRef CAS PubMed.
- V. Linares, G. Perello, M. Nadal, J. Gomez-Catalan, J. M. Llobet and J. L. Domingo, J. Environ. Monit., 2010, 12, 681–688 RSC.
- V. Linares, G. Perelló, M. Nadal, J. Gómez-Catalán, J. Llobet and J. Domingo, J. Environ. Monit., 2010, 12, 681–688 RSC.
-
MMA, Guía Técnica de aplicación del RD 9/2005, de 14 de enero, por el que se establece la relación de actividades potencialmente contaminantes del suelo y los criterios y estándares para la declaración de suelos contaminados, Ministerio de Medio Ambiente, Madrid, Spain, 2007 Search PubMed.
-
USEPA, Soil Screening Guidance: Technical Background Document, Office of Solid Waste and Emergency Response, Washington, D.C., 1996 Search PubMed.
-
USEPA, Toxicological Review of Hexavalent Chromium – DRAFT, EPA/635/R-10/004A, 2010 Search PubMed.
- M. Nadal, J. Rovira, J. Díaz-Ferrero, M. Schuhmacher and J. L. Domingo, Environ. Int., 2016, 97, 37–44 CrossRef CAS PubMed.
- X. Martinez-Lladó, M. Vilà, V. Martí, M. Rovira, J. Domènech and J. de Pablo, Environ. Eng. Sci., 2008, 25, 863–878 CrossRef.
- L. Vilavert, M. Nadal, M. Schuhmacher and J. L. Domingo, Biol. Trace Elem. Res., 2012, 149, 435–442 CrossRef CAS PubMed.
- J. Rovira, M. Mari, M. Nadal, M. Schuhmacher and J. L. Domingo, Chemosphere, 2010, 80, 1183–1189 CrossRef CAS PubMed.
- J. Rovira, L. Vilavert, M. Nadal, M. Schuhmacher and J. L. Domingo, Waste Manage., 2015, 43, 168–175 CrossRef CAS PubMed.
- L. Vilavert, M. Nadal, M. Schuhmacher and J. L. Domingo, Arch. Environ. Contam. Toxicol., 2015, 69, 241–253 CrossRef CAS PubMed.
Footnote |
† Electronic supplementary information (ESI) available. See DOI: 10.1039/c7em00121e |
|
This journal is © The Royal Society of Chemistry 2017 |