DOI:
10.1039/C6CS00632A
(Review Article)
Chem. Soc. Rev., 2017,
46, 2091-2126
Colloidal capsules: nano- and microcapsules with colloidal particle shells
Received
23rd August 2016
First published on 23rd February 2017
Abstract
Utilizing colloidal particles for the assembly of the shell of nano- and microcapsules holds great promise for the tailor-made design of new functional materials. Increasing research efforts are devoted to the synthesis of such colloidal capsules, by which the integration of modular building blocks with distinct physical, chemical, or morphological characteristics in a capsule's shell can result in novel properties, not present in previous encapsulation structures. This review will provide a comprehensive overview of the synthesis strategies and the progress made so far of bringing nano- and microcapsules with shells of densely packed colloidal particles closer to application in fields such as chemical engineering, materials science, or pharmaceutical and life science. The synthesis routes are categorized into the four major themes for colloidal capsule formation, i.e. the Pickering-emulsion based formation of colloidal capsules, the colloidal particle deposition on (sacrificial) templates, the amphiphilicity driven self-assembly of nanoparticle vesicles from polymer-grafted colloids, and the closely related field of nanoparticle membrane-loading of liposomes and polymersomes. The varying fields of colloidal capsule research are then further categorized and discussed for micro- and nano-scaled structures. Finally, a special section is dedicated to colloidal capsules for biological applications, as a diverse range of reports from this field aim at pharmaceutical agent encapsulation, targeted drug-delivery, and theranostics.
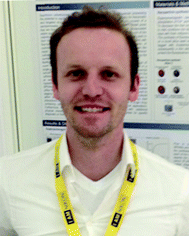
Tobias Bollhorst
| Tobias Bollhorst is currently a PhD student at the Department of Production Engineering at the University of Bremen. He received his MSc from the University of Bremen in 2012 and his BSc in Materials Science from the Technical University of Berlin in 2010. His research is focused on the synthesis and surface functionalization of inorganic and polymeric nanoparticles, nanoparticle self-assembly, and colloidal particles for drug delivery. |
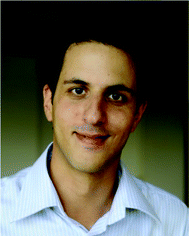
Kurosch Rezwan
| Kurosch Rezwan is a professor of Advanced Ceramics at the University of Bremen. He earned his Materials Science and Engineering Diploma (2001) and PhD degree (2015) from the ETH Zurich in Switzerland. Subsequently, he was a postdoctoral fellow at the Imperial College of Science and Engineering in London, before he joined the Faculty of Production Engineering Department at the University of Bremen in 2006. His research focuses on the interaction of advanced ceramics at the biointerface for biomedical and biotechnological applications. |
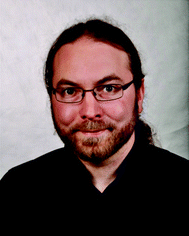
Michael Maas
| Michael Maas is a senior scientist at the Advanced Ceramics group at the University of Bremen. He received his diploma in chemistry in 2005 and completed his PhD in Physical Chemistry in 2008 at TU Dortmund. He then spent two years as a postdoc at Stanford University. His research focuses on the colloidal assembly of nanostructured materials from nano-sized building blocks, (bio)molecules and minerals. His work includes fundamental research on thin films for the formation of multifunctional colloidal capsules, utilizing biomineralization strategies for designing biodegradable nanocarriers, and exploring assembly and functionalization techniques for the preparation of anisotropic and patchy nanoparticles. |
1. Introduction
Various methods for the synthesis of nano- and microcapsules with densely packed colloidal particle shells have been introduced and greatly advanced over the course of the last two decades. These mainly include self-assembly routes based on Pickering-emulsification, templating of (sacrificial) particles, or the amphiphilicity-driven capsule formation from polymer-grafted colloids. And even though these diverse synthesis techniques rely on different assembly principles, the resulting structures feature one concurrent characteristic: a shell formed from one or more closely packed layers of colloidal particles.
Generally, micro- and nanoscaled capsules, which have been of intense research interest for many decades, comprise an organic, metallic, or inorganic shell to separate themselves from the surrounding environment and to protect a solid, liquid- or gas-filled interior. Such structures may be used for the encapsulation of a wide variety of compounds, including; pharmaceuticals,1 nutrients,2 catalysts,3,4 or fragrances.5 Until recently, the outer layer of nano- and microcapsules has mainly been utilized for the controlled interaction with its environment and for the protection and release of encapsulated cargo. Here, the outer layer functions either as a non-, partially, or highly permeable membrane. However, lately this paradigm is shifting and the shell itself is subjected to significant engineering efforts, with the goal to add distinct morphological, chemical, or physical properties; increasingly often by taking advantage of specific interactions of colloidal particles in the shell of a nano- or microcapsule. Utilizing modular colloidal building blocks to form one or more densely packed layers of a capsule's shell can lead to unprecedented material properties and may be advantageous in comparison to conventional encapsulation structures.
For instance, liposomes6–8 and polymersomes,9–11 artificial vesicles formed from natural and synthetic amphiphiles (phospholipids or block-copolymers), have been successfully developed for the entrapment and transport of active ingredients and play a critical role e.g. in the biomedical field for targeted pharmaceutical delivery. To further improve and to meet the growing demand for new multifunctional vesicular structures, a dense loading of the membrane of liposomes or polymersomes with nanoparticles or the self-assembly of capsules with a densely packed shell of colloidal particles can lead to advanced material properties. By carefully selecting the types of the shell-forming colloidal particles tailor-made exploitable characteristics can be achieved within such vesicle-like capsules.
In analogy to liposomes and polymersomes, these structures are often described as colloidosomes,12 after this term was coined for a distinct type of capsule with a shell of densely packed colloidal particles. However, it has come to our attention that the term ‘colloidosome’ may not be the most ideal match for the description of spherical structures with colloidal particle shells. The term was initially introduced to solely describe Pickering emulsion-based capsules, but was recently also used for capsules based on other assembly routes, e.g. template-based structures.13,14 In contrast, other terms were introduced by different authors who may have felt that their structures did not satisfy the original colloidosome definition, e.g. ‘nanoparticle vesicles’,15 ‘nanoparticle-stabilized nanocapsules’,16 or ‘raspberry-like nanocapsules’.17 This, and a clear evidence of a lack of cross-citation of the herein reviewed fragmented research fields, calls for a more general term that unifies the herein reviewed capsules. This would also greatly simplify the review of the literature from this field in the future. We think that the previously introduced term ‘colloidal capsule’18 fulfills the description of all types of capsules with a closely packed shell of colloidal particles more clearly. Hence, we advise the future use of this term to unify this research field.
Since the pioneering works by Caruso and Möhwald et al.,19 Dinsmore and Weitz et al.,12 and Nie and Kumacheva et al.,20 research on colloidal capsules has gained substantial momentum and shows great promise for their application in a wide range of fields, such as drug delivery,21 catalysis,22 energy storage,23 or photonics.24,25 The successful implementation of these colloidal capsules will strongly depend on the possibility to precisely control structural characteristics, such as size, morphology, and surface chemistry of the capsule itself as well as the shell-forming building blocks, and will further depend on the mechanical stability, shell permeability, monodispersity, and biocompatibility of the resulting colloidal capsule.
Recently, an increasing research interest has been dedicated to the novel physical features that may be derived from forming a closely packed spherical shell from modular colloidal building blocks. Here, plasmonic near-field coupling promoted by the accumulation of gold nanoparticles,26,27 the enhancement of various bioimaging techniques by e.g. utilizing the distinct superparamagnetic properties of iron oxide nanoparticles,28 the decreased fluorescence intensity proximity quenching of fluorophore-doped core–shell silica particles,29 or the integration of different nanoparticles with distinct functionalities in a single capsule30,31 underscore just a few functionalities of such novel nano- and microcapsules. Beyond the utilization of these aforementioned modular building blocks with specific physical properties, building blocks with unique morphological and biological characteristics from the materials and life sciences fields have also been employed to form densely packed shells of colloidal particles. These include e.g. cubical metal organic frameworks,32 mesoporous silica particles,33 janus particles,34 nanodiamonds,35 carbon nanotubes,36 nano-/microrods,37,38 as well as polymersomes,39,40 enzyme-loaded liposomes,41 or viruses (bionanoparticles).42 Furthermore, proteins are also being intensively explored as shell-forming biological building blocks, bringing about the nascent field of proteinosomes.43 These protein capsules, representing a special class of so called protocells,44–47 may be utilized as a tool for the investigation of the early origin of primitive cell-like structures.48 Hence, the inclusion of functional building blocks, particularly through specific nanoparticles, is inherent to this colloidal capsule platform and one of the main features of these structures. Furthermore, a strong focus in colloidal capsule research lies in pushing the structures ever closer towards biomedical applications, specifically for parenteral drug delivery, bioimaging and hyperthermia treatment. Here, the main challenges were found in miniaturization16,49,50 of the structures to nano-scaled sizes with tailor-made pharmaceutical agent release51 and controlled capsule disassembly, combined with particle clearance from the body in vivo.52
In the following sections we will review and discuss recent advances of the varying synthesis strategies of forming colloidal capsules, the exploitation of various building blocks with different chemical, physical, and morphological properties, capsule miniaturization, and their potential utilization for bionanotechnology applications. It should be noted that the structures described here are clearly to be distinguished from colloidal/nanoparticle clusters53,54 which represent another class of supraparticles. The goal of this review is to give a comprehensive overview on the recent progress in the field of nano- and microcapsules with colloidal particle shells and closely related structures. Although the majority of the herein discussed papers have been published in the last 10 years, we will also discuss seminal papers that defined a subfield of colloidal capsule research and preceded the bulk of the publications from the last years. We will start the discussion by introducing the different methods for the creation of colloidal capsules and nanoparticle membrane-loaded vesicles. Thereafter, we categorize the capsules based on their size and the building block materials. The review will then close with an overview of recent colloidal capsules for biological applications, including a critical discussion on whether such structures should be considered for in-human use.
2. Strategies for the synthesis of colloidal capsules
Various approaches for the synthesis of spherical structures with densely packed colloidal particle shells have been investigated in the past two decades. We categorize these into their four major themes, i.e. their Pickering emulsion-based synthesis (Section 2.1), the deposition and formation of densely packed colloids on template particles (Section 2.2), the amphiphilicity-driven self-assembly of polymer-brush functionalized colloids to ‘nanoparticle vesicles’ (Section 2.3), and the closely related field of liposomes and polymersomes with nanoparticle-loaded membranes (Section 2.4). The formation of these structures is based on bottom-up self-assembly processes of systems usually forced into a non-equilibrium state which are directed towards a thermodynamic minimum-energy state. The synthesis routes will be introduced and discussed in sequence of their historical appearance in the literature.
2.1 Emulsion-based synthesis routes for colloidal capsule assembly
Beginning in 1996, Velev et al.55–57 published pioneering works on the formation of supraparticles from oil–water emulsions, utilizing latex particles for the formation process. The latexes were sulfated or amidined to induce negative or positive surface charges to perform an interaction-tailored colloidal assembly in the particle/droplet system. This was followed by Dinsmore's 2002 paper who accomplished a further stabilization of these Pickering58–Ramsden59-emulsion-capsules which enabled their transfer to a fresh water phase resulting in water dispersed capsules with an aqueous core.12
In general, the original synthesis route for the formation of colloidal capsules via Pickering-emulsions, involves three major steps, which are depicted in Fig. 1A(A.1) the emulsification of a water-in-oil phase, with colloidal particles either dispersed in the oil or water phase, Fig. 1B(B.1) the confinement of the colloidal particles at the emulsion droplet oil–water interface, and finally a transfer of the stabilized emulsion-based capsule to a fresh continuous phase, usually water. Fig. 2 shows an example of one of the first dried ‘colloidosomes’, which was assembled from 0.9 μm sized polystyrene (PS) particles, whilst the PS particles were slightly sintered to form a stable shell.12
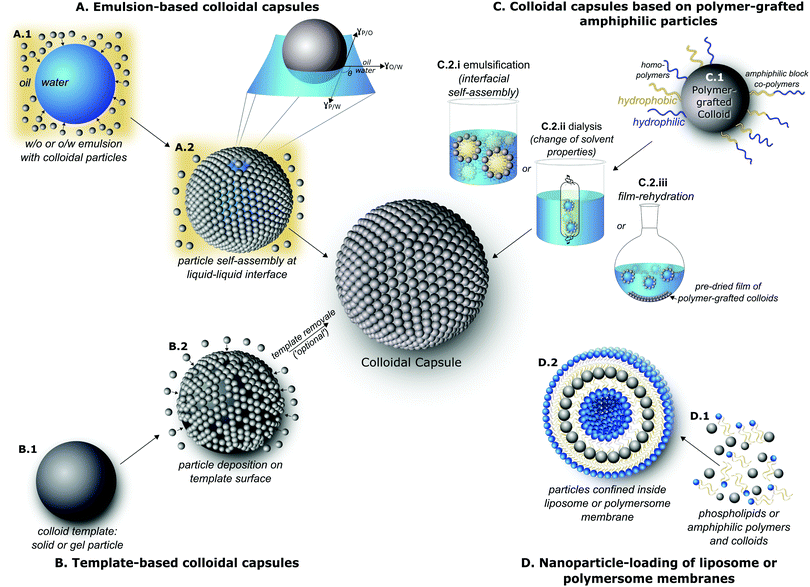 |
| Fig. 1 Overview of utilized routes for the formation of colloidal capsules: (A) emulsion-based colloidal capsules via interfacial assembly of particles at the oil–water interface of droplets; (B) colloidal capsules formation via templating against (sacrificial) particles; (C) colloidal capsule synthesis via amphiphilicity-driven self-assembly of polymer-grafted nanoparticles; (D) dense nanoparticle loading of the membrane of liposomes/polymersomes. | |
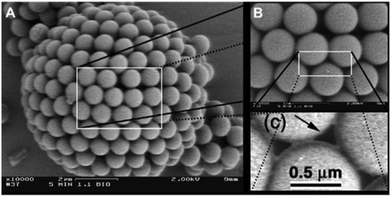 |
| Fig. 2 Initial ‘colloidosome’, formed from polystyrene particles (From ref. 12. Reprinted with permission from AAAS (2002).). | |
A great number of research papers and several reviews18,60,61 have since been published on emulsion based colloidal capsules, including a recent review by Thompson et al.62 which we like to point out for secondary citations. This review particularly summarizes the key routes for the stabilization of the shell of the particle-stabilized emulsion droplet, i.e., thermal annealing (shell sintering),12,63,64 gel trapping,65–67 covalent cross-linking,68–70 and the polymerization of either the inside or the surface of the emulsion droplet.71–74
2.1.1 Particle confinement at the liquid–liquid interface.
To successfully synthesize colloidal capsules via Pickering emulsification, a confinement of colloidal particles at the liquid–liquid interface of the emulsion droplet is a major prerequisite. The self-assembly of colloidal (nano-)particles at liquid–liquid interfaces is driven by the reduction in Helmholtz free energy, where the fluid–fluid surface area is replaced with particle–fluid surface area.12 Additionally, the tendency for colloidal particles to adsorb and stay at the interface depends on various factors, including the particles surface chemistry, which influences the particles wettability by the oil and water phase, and the particles radius (r). Fig. 1B.1 (zoom-in) schematically depicts a particle confined at the emulsion-droplet interface, including the contact angle (θ) of the particle with the interface, which depends on the surface free energies (interfacial tensions (γi/j)) at the particle–water (P/W), particle–oil (P/O) and oil–water interface (O/W).75 The energy change (ΔE) for one particle confined at the interface, reducing the total free energy, was initially introduced by Pieranski76 and has been greatly used by other authors for the description of particle behaviour at liquid–liquid interfaces since then:75,77,78
Hence, with decreasing particle sizes, ΔE is decreased as well and approaches similar values as kBT (kB: Boltzmann constant) making the colloidal particles more susceptible to momentum transfer from solvent molecules. Consequently, for very small particles (d ≪ 50 nm) and depending on particle wettability by either phase, this can result in diminished confinement at and possible detachment of particles from the liquid–liquid interface due to thermal fluctuations.78 This makes the emulsion-based synthesis of miniaturized colloidal capsules with small particles more complex.
Significant research interest is dedicated to the study and advancement of the adsorption and desorption of colloidal particles at fluid interfaces. However, the elucidation of the full details of this field would go well beyond the scope of this review, as why we would like to redirect the interested reader to the books by Binks75 and Ngai et al.79 (which both include detailed mathematical descriptions) and recent research studies,80–91 novel measurement techniques,92–95 and recent reviews96–105 for further information.
2.1.2 Microfluidic-based colloidal capsule synthesis.
The formation of colloidal capsules via classical emulsification approaches tends to lead to a fairly broad size range of the capsule diameters in a single sample. To decrease the rather high polydispersity observed in emulsion-based capsules, various groups investigated the utilization of microfluidics to further control the synthesis of these structures. For instance, Subramaniam et al. utilized a three-channel hydrodynamic focusing device to assemble 4 μm polystyrene colloids to a “jammed shell”.106 Similarly, Utada and Weitz et al. used microcapillaries to control the formation of double emulsions,107 which were later used for capsule synthesis. Within the double-emulsion synthesis route, hydrophobic particles are usually dispersed in the organic phase of a water-in-oil-in-water double-emulsion, and the evaporation of the organic solvent then leads to the final colloidal capsule formation.108 This method has since been extended by Weitz and co-workers to form nonspherical colloidal capsules,109 to incorporate a Pluronic polymer core for thermally switched release,110 for the generation of stimuli-responsive capsules formed from poly(N-isopropylacrylamide) particles,111 and by Sanders and Studart et al. by utilizing nanoparticles with magnetic, photocatalytic, and potentially biocompatible functionalities in the shell.112 Additionally, Park and Kumacheva et al. synthesized closely related structures, by assembling poly(styrene-co-acrylic acid) particles on CO2 bubbles.113 In a follow-up report the process was further tailored by using a temperature-dependent dissolution of CO2.114 Nie and Kumacheva et al. also investigated an “inside-out” approach by emulsifying a dispersion in a particle-free continuous phase.115 Besides this, Abell et al. used polymer–gold nanoparticles to also synthesize micrometer-sized colloidal capsules.116 Albeit, two challenges within this synthesis route remain, the first being a controlled miniaturization of the final structures, which has been approached by Nie et al. with block-copolymer (BCP)-tethered colloids,117,118 and secondly an increase of the overall yield and throughput of this method.
2.2 Templated synthesis of colloidal capsules
In 1998 Caruso laid ground for the field of the templated formation of colloidal capsules by first reporting the sequential adsorption of SiO2 nanoparticles and oppositely charged polyelectrolytes on polystyrene (PS) template particles119via electrostatic interactions. Shortly afterwards, this process was advanced by etching the PS particles and creating hollow capsules19 (Fig. 3). This layer-by-layer (LbL) templating approach allows for a precise control of the thickness of the shell by depositing a defined number of layers on the template, which at the same time also determines the capsule's size and morphology. Various template structures have been investigated, including inorganic and hard or soft polymeric colloids. Since the introduction of this technique, numerous excellent reviews on layer-by-layer templating routes have been published, including articles by Caruso et al.120,121 and a review by X. Lou et al.,122 which we would like to point out for secondary citations. Also discussing various types of capsules and other superstructures with non-colloidal particle shells. For means of a simpler overview, we divided this section into hard (Section 2.2.1) and soft (Section 2.2.2) template-based structures.
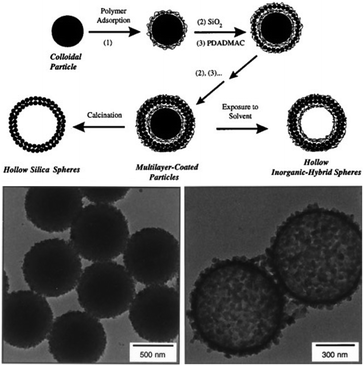 |
| Fig. 3 (top) Template based synthesis route; (bottom) colloid templated structures before (left) and after (right) core etching. (From ref. 19. Reprinted with permission from AAAS (1998).) | |
2.2.1 Hard templates.
The initial synthesis route utilizing hard colloids as templates is illustrated in Fig. 3 and consists of polymers (polyelectrolytes) (1) and nanoparticles (2) which are sequentially assembling on the template particle via electrostatic interactions. This may be followed by either a solvent-induced dissolution of the template core or by calcination, which, besides the dissolution of the template particle, usually also removes the polyelectrolyte layers, only leaving behind the self-assembled nanoparticle shell. In 2000, Caruso published a concept paper further elucidating this process.123 Recently, Caruso et al. utilized polyphenol-conjugated colloidal building blocks, which, among the formation of other colloidal superstructures, could be assembled on spherical PS templates for colloidal capsule formation. After the assembly process, the shell-forming building blocks were linked and stabilized via metal–phenol complexation utilizing different metal ions.124 Another templating route also without using polyelectrolytes, solely based on the deposition of PS nanoparticles onto silica microspheres, was introduced by Fleming and co-workers. Here, the PS particles are sintered prior to the etching of the silica template, leading to a fused surface.125 In addition to the direct use of nanoparticles for the assembly on a colloidal template, ionic precursor solutions have been investigated as well, to initiate nucleation and growth of nanoparticles on the template. For instance, Kim et al.126 coated thiolated silica particles with a palladium precursor and induced a formation of approx. 10 nm-sized Pd nanoparticles, and subsequently etched the core with hydrofluoric acid (HF) to retrieve hollow capsules.
Besides the use of solid colloidal particles for the formation of the capsule's shell, proteins have also been employed as building blocks. Merz and Caruso et al. have prepared nano- and microcapsules with a protein building block shell from human serum albumin (HSA) on nonporous silica templates,127 doxorubicin-conjugated capsules using various different types of proteins,128 and nano-scaled HSA based capsules with MRI and gene-silencing functionalities by using a mesoporous silica template for encapsulation prior to the templating step.129
2.2.2 Soft (gel) templates.
Along with hard particle templates, “soft” gel-like particles have also been studied significantly for the formation of a closely packed layer of colloidal particles on their surface. In many works, a full coverage of the soft/gel-like templates is achieved by shrinking the bulk template after covering its surface with a certain amount of particles. In 2007, Kim and Weitz et al. used temperature sensitive microgel templates with sizes ranging from 50–60 μm which were based on poly(N-isopropylacrylamide) (PNIPAm). The PNIPAm particles were first sparely covered with smaller polystyrene particles and then jammed by shrinking the PNIPAm template to achieve a closely packed particle coverage on the surface.13 A very similar strategy was shortly later applied by Karg et al. for smaller poly-(NIPAM-co-allylacetic acid) nanogel templates featuring final sizes of approx. 320 nm. The nanogels were templated with gold-nanorods to investigate their shifted resonance peak, induced by the near-field plasmonic coupling of the Au rods.37 Gawlitza and Karg et al. later on also used spherical gold nanoparticles for a more sparse decoration of PNIPAm microgels.130 Additionally, PNIPAm was also used as a template material by Du et al. who added a pre-silanized aqueous TEOS solution (silica sol) to form a closed shell of silica particles on PNIPAm aggregates.131 In contrast to these previous reports utilizing PNIPAm, we recently investigated chitosan nanoparticles as substrates for the adsorption of 5 nm and 15 nm fluorescent SiO2 particles with the intention to increase the biocompatibility of the template material.132 Another approach was reported by Destribats et al. who adsorbed CTAB-coated silica particles on molten wax droplets, which were in turn able to release the wax upon heating to approx. 44 °C.133 The release of various agents from microgel templates enclosed with colloids was shortly afterwards examined systematically by Rosenberg and Dan and co-workers.134–136 Recently, Huang et al. reported a novel approach by templating vesicles formed from poly(3-ethyl-3-oxetanemethanol)-PEO block-co-polymers by in situ formation of gold, silver, or platinum nanoparticles on the vesicle surface,137 which is however different from the works described in the next section of colloidal capsules formed from polymer-grafted particles.
2.3 Amphiphilicity-driven colloidal capsule self-assembly from polymer brush grafted nanoparticles
Two decades ago, Eisenberg et al. pioneered the self-assembly of amphiphilic block copolymers into various morphologies, including spherical vesicular structures.9,138,139 Shortly after, the term polymersome was coined by Disher et al.10 to describe polymer vesicles, in analogy to liposomes. Diblock or triblock copolymers are now ubiquitous and widely used for the synthesis of polymersomes, by making use of the hydrophobic effect140 which induces a water-mediated association of the hydrophobic polymer units, while the hydrophilic units protrude towards aqueous media.141 Numerous reviews have since been published on polymersomes.11,142,143
Recently, the principle of amphiphilicity-driven self-assembly has been transferred to colloidal particles for the formation of capsules with colloidal particle shells. Among this, three routes have mainly been used to control the self-assembly of amphiphilic polymer-grafted particles to vesicle-like capsules, including emulsification by preparing oil-in-water emulsions and subsequently evaporating the organic solvent (Fig. 1C.2.i),52 dialysis from a selective solvent against water with the intention to slowly change the solvent composition (Fig. 1C.2.ii),144 or by drying a thin-film of the particles on the wall of a vial and then rehydrating the film (Fig. 1C.2.iii).145 Due to various similarities to molecular amphiphile based vesicles – liposomes and polymersome – these structures are often termed ‘nanoparticle vesicles’.15 However, their overall structural build-up and inherent properties are correspondent to colloidal capsules.
Following a first report on the assembly of polymer-conjugated nanoparticles to spherical aggregates,146 in 2006 Zubarev et al.147 and in 2007 Nie et al.20 published the first works on the three dimensional self-assembly of polymer-brush functionalized colloids to hollow tubular and spherical structures. Zubarev et al. grafted a V-shaped PS40–PEO50 amphiphile to the surface of 2 nm gold or silver particles and then induced the self-assembly by first slowly adding a certain amount of water to a dispersion of the particles in organic media (i.e. DMF or THF) and secondly dialyzed this mixture against water to remove the remaining organic media. Depending on the NP concentration and organic solvent either worm-like or vesicular assemblies were reported by the authors. Similarly, ‘Pom-pom’-like gold nanorods (Fig. 4 – left), coated with CTAB and conjugated on each end with hydrophobic polystyrene brushes reported by Nie et al., showed, in analogy to ABA block-copolymers, a self-assembly to structures with varying geometries, including vesicle-like capsules (Fig. 4 – right), which depended on the solvent composition. Grubbs mainly attributed this solvent-tuned self-assembly process of the amphiphilic-like colloids to a non-thermodynamically stable ‘trapping’ of the structures based on a solidification of the polymer domains in ‘kinetically stable amorphous phases’.148
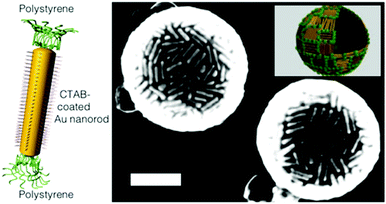 |
| Fig. 4 (left) Illustration of a hydrophilic CTAB-mediated gold nanorod with hydrophobic polystyrene brushes at its ends; utilized for nanoparticle vesicle formation, represented in the SEM micrograph (right). The inset in the SEM micrograph schematically showcases the arrangement of the Au nanorods in the capsule shell (Reprinted with permission from Macmillan Publishers Ltd: [Nature Materials] ref. 20, copyright (2007).). | |
Since these two pioneering reports, an especially strong focus has laid on polymer-brush functionalization of gold nanoparticles with their subsequent self-assembly to nanoparticle vesicles. Due to the distinct association of the hydrophobic units with each other, most of these vesicle-like structures feature a closed hydrophobic polymer membrane layer on their surface, allowing for an efficient encapsulation of a wide variety of active agents. The main focus in this field has since been on forming vesicles from nanoparticles with either diblock, mixed, or Janus149 polymer brush architectures. Nie et al. as well as Song et al. have published a series of papers, including various recent reviews by each,26,27,150,151 bringing significant progress to this field, with a strong focus on utilizing these vesicles for biomedical applications. Nie and co-workers concentrated on grafting gold nanoparticles with block-copolymers, whilst Song and co-workers laid their focus on grafting gold nanoparticles with a mixed homopolymer architecture (see Fig. 1C.1).
Song et al. used combinations of PEG with either PMMA/PMMAVP,49,152 PNBA,153 PLA,154 or PLGA52,155 for vesicle formation, conjugating the particles either via a two step grafting-“To” and subsequent grafting-“From” approach, or by concurrently bonding pre-synthesized hydrophilic and hydrophobic homopolymers onto the nanoparticles via grafting-“To”. Song et al. also recently published a comprehensive summary of the various protocols for the synthesis and characterization of the previously reported polymer-grafted gold particles and self-assembled capsules.156
Following their work on the self-organization behaviour of PS-grafted ‘Pom-Pom’-like gold nanorods,20,157,158 Nie et al. mainly focused on conjugating gold colloids with block copolymers. When utilizing PEOx-b-PSy BCPs, the tendency of the polymer-grafted colloids to form vesicles was observed to depend on a combination of the size of the colloidal particles and the length of the hydrophilic and the hydrophobic polymer units.15 Submicron-sized vesicles were assembled from nanoparticles with a flower-like145 or spherical144,159 morphology and larger, micron-sized vesicles,160 were formed from gold-nanrods. The capsules could be synthesized using varying assembly methods and PEO-b-PS BCPs of different lengths, and allow for a near-infrared light triggered release of encapsulated cargo due to a red shift of the plasmon resonance bands. In addition to this light-triggered disaggregation, light (ultra violet) has also been used to assemble vesicles from various thiol-capped inorganic nanoparticles by oxidation of mercapto containing ligands which supposedly induces a rearrangement of the ligands.161 By further controlling the grafting density of the BCPs on gold nanoparticles, vesicles with string-like assemblies could be formed.162,163 In another report, the hydrophobic PS block was substituted for a biodegradable poly(ε-caprolactone) block for increased biocompatibility.50
In addition to Song's and Nie's works, Förster et al. described the spontaneous self-assembly of hydrophobic CdSe/CdS particles, also featuring a hydrophilic PEO chain, into various structures, including large vesicles. They compared the self-assembly behaviour of the particles to that of surfactants and lipids.164 Moffitt et al. coated CdS NPs with a PS-b-PAA-b-PMMA triblock copolymer and were also able to form vesicle-like assemblies.165 Hu et al. synthesized polymer-grafted nanoparticles with a Janus-like brush architecture, by binding the central block of an amphiphilic triblock copolymer (PEO-b-P(LAMP-co-GMA)-b-PS) to 2.0–3.8 nm sized gold nanoparticles. The particles assembled to various structures, including vesicles that are believed to form a polymersome-like double-layer of particles.149 Overall, the here described colloidal capsules feature some physico-chemical similarities to the structures reviewed in the following section. However, in the next section the colloidal building blocks are not functionalized with polymer brushes to mimic the behavior of amphiphilic molecules, but instead conjugated with hydrophobic ligands to enable their dense confinement in a vesicle's membrane formed from free lipids or block copolymers.
2.4 Liposomes and polymersomes with nanoparticle-loaded membranes
With the first works on liposomes dating back to the 1960s by Bangham et al.,166–168 liposomes are now omnipresent and have found their way into numerous consumer applications and represent a crucial instrument in the pharmaceutical field.169 A large amount of research was and still is devoted to the functionalization of liposomes. We are aware of a myriad of excellent studies in the field of liposomes/polymersomes in general and vesicle–nanoparticle hybrids specifically, for instance previously reviewed by Al-Jamal et al.170 as well as Amstad and co-workers.171 However, we will here exclusively concentrate on amphiphile-assembled vesicles which resemble a colloidal capsule by featuring a closely packed spherical layer of nanoparticles inside the membrane of liposomes or polymersomes, as depicted in Fig. 1D.2.
2.4.1 Liposomes with nanoparticle-loaded bilayers.
In 2003 Cha et al. demonstrated the incorporation of quantum dots in the shell of poly-L-lysine vesicles.172 Following this and other pioneering works of sparely loaded particles in a vesicle membrane,173–185 in 2010 Rasch et al. were the first to assemble closely packed nanoparticles inside the lipid bilayer of liposomes from phosphatidylcholine and thoroughly characterized them via cryo-TEM (Fig. 5).186 Here, dodecanethiol-coated gold nanoparticles were used for the membrane loading, where the core was merely 2 nm in diameter. The small particle size was reportedly chosen to inhibit lipid adsorption around the nanoparticle and to reduce disrupting effects to the bilayer, which might be induced if larger nanoparticles are intended to be loaded into the membrane. The dodecanethiol-coated NP were loaded inside the membrane of the vesicles using a synthesis route based on a combination of film-rehydration and mini-extrusion, leading to vesicle sizes as low as 50 nm. Interestingly, only vesicles with either no particles or a closely packed monolayer of particles were formed. In a follow-up report, Rasch et al. further examined parameters that lead to vesicle disruption.187
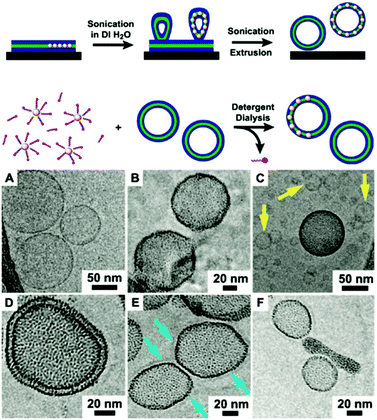 |
| Fig. 5 (top) Illustration of the initially reported synthesis routes for densely nanoparticle-loaded liposome membranes; (top) either via the rehydration of lipid Au NP thin film followed by extrusion through a 50 nm-sized filter, or (bottom) by dialyzing pre-formed and unloaded liposomes in combination Au NP and detergents. (bottom) Cryo-TEM micrographs of (A) unloaded vesicles and (B–F) Au NP-loaded liposomes with the particles embedded in the phospholipid bilayer (Adapted with permission from ref. 186. Copyright (2010) American Chemical Society.). | |
The particle size in relation to the membrane thickness, which is usually in the range of 5 nm, plays a pivotal role regarding the particle confinement inside the bilayer of liposomes. Ultrasmall particles with diameters below 2 nm form densely packed layers, particles with diameters between 2 and 6.5 nm tend to disrupt the bilayer and may create small cavities in their close periphery or bridge adjacent liposomes, whilst particles above 6.5 nm tend to adsorb the lipids, which inhibits vesicle formation and leads to micellization.188 For a thorough discussion on the interaction of nanoparticles with liposome and polymersome membranes we recommend the review by Schulz and co-workers.189
Besides embedding Au NP in the lipid bilayer of liposomes, a strong focus in this field also lays on the incorporation of ultra small iron oxide nanoparticles (USPIONs) inside the bilayer, which accordingly fall into the field of magnetoliposomes.190 Amstad et al. incorporated palmityl-nitroDOPA stabilized <5.5 nm iron oxide NPs in the membrane of liposomes from 2-dis-tearoyl-sn-glycero-3-phosphocholine (DSPC).191 Using an alternating magnetic field to heat the embedded iron oxide NP, the authors were able to change the permeability of the membrane, allowing for a controlled release of an encapsulated fluorescent dye from the liposomes. A similar process was later examined by Qiu and co-workers.192,193 Furthermore, Katagiri et al. also used Fe3O4 NP in the lipid bilayer to synthesize magnetoresponsive liposomes which were additionally conjugated with thermosensitive polymers.194
2.4.2 Polymersomes with nanoparticle-loaded membranes.
Analogous to liposomes, polymersomes also possess particle incorporation capacities within their membrane and are gaining significant momentum as multivalent nanocontainers. For instance, Wei et al. synthesized HOOC–PEG–PLA BCP based polymersomes, which were first loaded with a dense spherical layer of 6 nm-sized hydrophobic Fe3O4 particles, and subsequently conjugated with an antibody using EDC-NHS cross-linking chemistry.195 The polymersomes were then used as novel immunosensors. Similarly, Sanson and Lecommandoux et al. incorporated <8 nm γ-Fe2O3 NP in the membrane of poly(trimethylene carbonate)-b-poly(L-glutamic acid) (PTMC-b-PGA) based polymersomes using a nanoprecipitation approach.196 In a follow-up report, Oliveira and Lecommandoux et al. used these PTMC-b-PGA vesicles as stimuli-responsive magneto-polymersomes for the local induction of a hyperthermia effect in tissue in close proximity to the polymersome membrane.197 Furthermore, various reports in this magneto-polymersome field were made by Park et al., who achieved a control of the architectural build-up of magneto-polymersomes and nanoparticle–micelle hybrids (magneto-micelles). The structures were formed using poly(acrylic acid) and PS BCPs and the resulting morphology could be controlled by varying the relative volume ratio of the hydrophilic and the hydrophobic blocks.198 Recently, Park et al. also accomplished a precise tailoring of the size of magneto-polymersomes and thoroughly characterized the morphological properties of the structures. The authors were able to control the size of the polymersomes via the incorporation of iron oxide particles with different sizes.199 Additionally, Park et al. were able to create multicompartment polymersomes by utilizing 11-mercapto-1-undecanol-capped gold nanoparticles for the membrane loaded polymersome synthesis.200 Interestingly, it was also recently reported that the combination of PEG–PS BCP-tethered colloids and a second free amphiphile PS–PEO BCP led to the concurrent assembly of further Janus-like polymersome–nanoparticle hybrid structures, including (hemi-) spherical janus, disc-like janus, and patchy vesicle morphologies (Fig. 22).201 In follow-up report, Nie et al. further advanced these janus vesicles by incorporating hydrophobic Fe3O4 NP along with Au NP grafted with PS-b-PEO inside the membrane of vesicles formed from free PS-b-PAA BCPs.202
3. Micron-sized colloidal capsules
Micron-sized colloidal capsules have been covered extensively in the scientific literature in the past decades and are usually more simple to synthesize and to characterize than their smaller submicron-sized siblings. Due to their larger size, there will most likely not be much reasoning to implement them in advanced applications. However, they are indispensable tools for the investigation of colloidal self-assembly phenomena at the micron-scale, allowing for a simple in situ visualization.
Most of the above described routes have been utilized to generate >1 μm colloidal capsules. However, there is clearly a great majority of structures created via the emulsion route in comparison to the other techniques. Since emulsion-based colloidal capsules are either themselves Pickering emulsions or a derivative of them, there are a myriad of structures formed and described in the literature based on this route. Hence, it would go well beyond the scope of this review to describe every structure ever generated and described in the available literature which is based on the Pickering-emulsion route. Besides recent reviews on Pickering-emulsions61,203 and the recently published book by Bon and Ngai,79 we like to again point out the review by Thompson et al.204 and another review by Patra et al.,18 which summarized a majority of structurally stabilized colloidal capsules from Pickering emulsions and we will refrain from describing the therein reviewed works in detail. Therefore, in the following section of micron-sized colloidal capsules, we will mainly focus our descriptions on recently reported structures with unique morphological and physical properties, formed from any of the above described synthesis routes.
3.1 Building blocks and colloidal capsules with distinct morphological characteristics
In the past few years a broad range of colloidal capsules with unique morphological characteristics have been reported in the literature. Capsules were e.g. formed from cubical metal organic frameworks (MOFs) based on a solvothermal synthesis route (Fig. 6). Here, Pang and co-workers heated a precursor solution to 120 °C that eventually formed Fe-soc-MOF particles which then spontaneously self-assembled on emulsion droplets.32 Further colloidal capsules also formed from MOFs and eventually termed by the authors as “MOFsomes” were prepared by incorporating spherical MOF particles into the surface of hollow polystyrene capsules.205 Utilizing a one-pot synthesis route, Xu et al. formed homogeneous and ‘particle-doped’ (Fig. 7) colloidal capsules by assembling the shell-forming building blocks at a water–gas bubble interface. A precursor solution either containing a certain amount of phenol monomer, or a combination of the phenol monomer with AgNO3 or HAuCl4 led to the formation of a phenol formaldehyde resin (PFR) shell, giving rise to homogeneous Ag@PFR or Au@PFR colloidal capsules. When the authors added some pre-synthesized Ag@PFR particles to the initial reaction system they observed the formation of novel “doped Ag@PFR-PFR colloidosomes”.206 Further structures formed from a set of differently sized particles of silica or polystyrene microspheres and silica or titania nanoparticles were described by Cho and co-workers. By combining two particle types of different length scales, bimodal colloidal clusters were reported by the authors. Removale of larger PS particles via calcination at 500 °C then led to hollow colloidal structures (Fig. 8).207 For more colloidal capsules with a patchy surface structure we recommend the recent review by Rozynek and Jozefczak.208
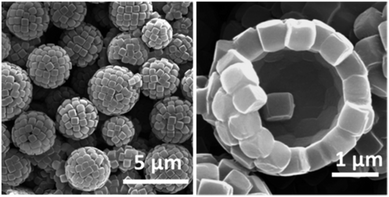 |
| Fig. 6 SEM images of colloidal capsules prepared from metal organic framework building blocks (Reprinted (adapted) with permission from ref. 32. Copyright (2013) American Chemical Society.). | |
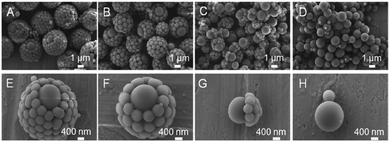 |
| Fig. 7 SEM images of “doped Ag@PFR-PFR colloidosomes (Reprinted with permission from ref. 206. Copyright (2013) American Chemical Society.). | |
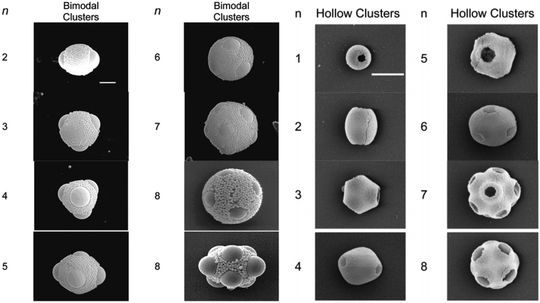 |
| Fig. 8 Left: SEM micrographs of structures formed from two sets of silica particles (scale bar: 2 μm). Right: Structures formed from larger PS and silica NP after removal of PS particles via calcination. (Adapted with permission from ref. 207. Copyright (2005) American Chemical Society.) | |
Besides these MOF-based and bimodal structures, a variety of capsules with oblong building blocks have been reported. Noble and co-workers first reported the use of microrods for the stabilization of a Pickering-like emulsion and subsequent capsule formation. They adsorbed SU-8 polymeric microrods at the interface of an aqueous agarose microgel which was intermediately washed with an ethanol–water solution and finally dispersed in pure water. To further retain the microrods in the shell, the authors stabilized the shell by using small amounts of glutaraldehyde to cross-link the shell-forming rods.38 Similarly, Datskos et al. also used a Pickering-emulsion approach to form colloidal shells from silica microrods.209 Vanadium pentoxide (V2O5) nanorods were formed by Cao et al. from a vanadium(III) acetylacetonate precursor.23 Using a process based on the Kirkendall210 effect Liu and Zeng formed “Dandelion” structures with a shell of ZnO nanrods.211 Furthermore, the authors also investigated Dandelion-like hollow capsules with a shell of CuO nanoribbons212 and smaller unidirectionally attached ZnO nanorods.213 Hollow capsules with a shell of multi-walled carbon nanotubes (MWNT), termed as “carbon nanotubosomes”, were synthesized by Paunov an co-workers. In their first work, the authors explored an emulsion-based approach214 and in a second report templated aminated MWNTs against sulfated PS particles which were later dissolved in toluene.36 In both approaches the MWNT shell was cross-linked with glutaraldehyde.
Plate-like building blocks have also been utilized for the stabilization of Pickering-emulsions215 and utilized in other approaches. For instance, Wang et al. formed Ni(OH)2-based nanoflakelets on styrene-acrylic acid copolymer particles and retrieved hollow structures after dissolving the cores in toluene.216 Subramaniam et al. synthesized semi-permeable capsules from natural clay montmorillonite building blocks by assembling the clay from water on gas bubbles.217 Sander and Studart used double-emulsions prepared via microfluidics to assemble monodisperse capsules from 8–10 μm sized montmorillonite clay particles. In addition to these oblong- and plate-like shaped building blocks further particles with unique morphological properties for the formation of the capsule's shell include cross-linked polymersomes,39 mesoporous silica particles,33 or Janus particles.34,218–221 A distinct kind of the latter anisotropic colloid type with a ‘snowman-like’ shape and hydrophobic and hydrophilic moieties, was recently explored by Evers et al. for colloidal capsule formation. Based on ‘colloidal bond hybridization’, defined by the authors as a redistribution of flexible surface groups of mutually attractive and deformable anisotropic particles, in analogy to bond hybridization, 3.7 ± 0.8 μm sized capsules were prepared.222
3.2 Distinct physical properties of building blocks and resulting colloidal capsules
Colloidal particles with distinct physical properties such as iron oxide nanoparticles with superparamagnetic properties and noble metal colloids with near-field plasmonic coupling characteristics have been significantly investigated to create tailor-made functionalities in micron-sized colloidal capsules. Taking advantage of adjacent particles in the shell of colloidal capsules is a unique characteristic of these structures and has allowed the development of distinct material properties in colloidal capsules.
3.2.1 Colloidal capsules with magnetic nanoparticles.
Duan et al. formed micron-sized colloidal capsules from hydrophobic iron oxide nanoparticles with different sizes (4.0, 5.0, and 8.0 nm). The authors dispersed 2-bromo-2-methylpropionic acid capped particles in toluene and then assembled the particles on emulsion droplets from pure water or from a heated aqueous agarose solution. Decreasing the temperature, and by that gelling the agarose core, led to a structural stabilization of the capsules. The resulting colloidal capsules could easily be washed by magnetic separation.67 Based on a Cu(I)-catalyzed Huisgen click reaction, Rotello et al. cross-linked iron oxide nanoparticles at oil–water interfaces. Here, 11 nm-sized oleic acid-capped particles were functionalized prior to their assembly via a ligand-exchange reaction with alkyne and azide groups and led to capsules with diameters of 49 ± 15 μm.223 In addition to iron oxide nanoparticles, Rotello and co-workers also used magnetic FePt particles for the creation of colloidal capsules. The FePt particles featured sizes of 7 ± 1 nm which were capped with terpyridine thiol groups and dispersed in toluene. Upon emulsification with an aqueous Fe(II) tetrafluoroborate hexahydrate solution, the particles assembled and formed a stable interconnected membrane at the droplet interface.69 Sander et al. successfully increased the monodispersity of magnetic capsules via a microfluidic w/o/w double-emulsion approach. Here, the capsules were assembled from a combination of Al2O3 and Fe3O4 particles which were dispersed in an organic toluene phase with the surfactant sorbitan trioleate. The authors were able to track the movement of the capsule with a microscope, where a magnetic field rearranged the particles within the shell leading to Janus-like capsules.112 Zhang and co-workers assembled magnetic colloidal shells from rod-like Fe3O4–SiO2 Janus nanoparticles. Stemming from the superparamagnetic properties of the rod-like janus particles, the capsules could be aligned under a magnetic field (Fig. 9).218 Furthermore, colloidal capsules formed via an emulsion-based synthesis were also created from PS-coated FeOx particles by Kaiser et al. were investigated to serve as a controlled release system.224 Akartuna et al. used Fe3O4 – among other particles – for the stabilization of oil-in-water colloidal capsules which were in situ hydrophobized with water-soluble surfactants.225 Liu et al. reported drug-containing colloidal capsules with a gelled agarose core and a Fe2O3 shell.226
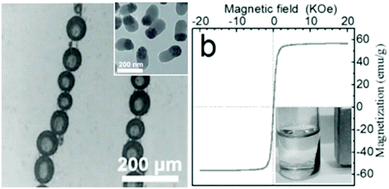 |
| Fig. 9 (left) Emulsion-based capsules aligned under a magnetic field; inset: shell-forming rod-like SiO2–Fe3O4 Janus particles; (right) Superparamagnetic properties of rod-like particles and magnetic response of capsules to an external magnetic field (From ref. 218. Reproduced with permission from The Royal Society of Chemistry.) | |
3.2.2 Colloidal capsules with noble metal particles or quantum dots.
As briefly mentioned above, quantum dots and noble metal particles have been explored as well for the creation of micron-sized colloidal shells. In specific, noble metal particles may induce plasmonic near field coupling of particles in close proximity to each other. Here, new optical properties may be created due to the coupling of surface plasmons, which is indicated by shifts of the coupled plasmon modes towards longer wavelengths.227 We will start the discussion of this section with quantum dots.
In one of the first reports, in 2003, Lin et al. pioneered the crosslinking of colloidal shells from vinylbenzene-capped CdSe nanoparticles. The cross-linking was performed at a toluene emulsion droplet interface within an aqueous solution of 2,2′-azobis(2-(2-imidazolin-2-yl)propane) dihydrochloride at 60 °C for 6 h.228 Skaff et al. later advanced this approach with cyclic olefins-capped CdSe/ZnS nanoparticles and interfacial cross-linking via so called ring-opening metathesis polymerization (ROMP).229 Rotello and co-workers investigated another approach of ligand-capped colloidal shells. The authors pre-synthesized hydrophilic and hydrophobic gold nanoparticles, by respectively capping a first type of colloid with beta-cyclodextrin and a second type with adamantane. Interaction of the two moieties at a droplet interface led to stable colloidal capsules. However, in contrast to cross-linked shells, the particle interaction could be mitigated by adding an amphiphilic molecule that led to partial coalescence and eventually capsule growth.230 Beyond this, Rotello et al. also used enzymes (Candida rugosa lipase), similar to their work on nanoscaled colloidal capsules discussed below, to stabilize micron-sized oil-in-water gold particle shells.231 Using MF particles as templates, Caruso et al. prepared near-infrared (NIR) responsive polyelectrolyte–gold nanoparticle capsules. The resulting capsules could be ruptured by pulsing them with laser light allowing a controlled release of encapsulated cargo.232,233 Also utilizing gold nanoparticles, Glogowski et al. assembled PEGylated 2 nm gold colloids at the interface of an oil-in-water emulsion. The colloidal shells were assembled on large 60–200 μm droplets and could be downsized by extrusion through polycarbonate membranes with defined pore sizes to diameters below 10 μm.234 Gold nanorods tethered with PEO45-b-PS211 were assembled to giant vesicles with diameters of up to 2 μm via a microfluidic-based continuous self-assembly.160 In contrast, Liu et al. used larger gold nanoparticles with a monodisperse size of about 100 nm for the synthesis of a colloidal shell via a water-in-butanol emulsion. The micron-sized capsules display a plasmonic coupling effect, evidenced in a shift of the LSPR peak (Fig. 10).235 Besides gold colloids, Anandhakumar et al.236 and Radziuk et al.237 created colloidal capsules from silver nanoparticles, synthesizing the capsules via the classical LbL colloidal templating approach.
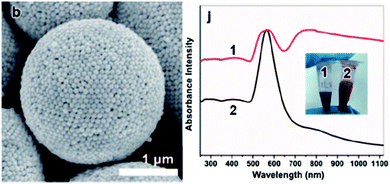 |
| Fig. 10 (left) “Black Gold” colloidal capsule, (right) displaying plasmonic coupling (curve 1) in comparison to freely dispersed gold particles (curve 2). (Reproduced with permission ref. 235. Copyright 2015, Wiley-VCH Verlag GmbH & Co. KGaA.) | |
3.3 Capsules with proteins as shell-forming building blocks
Besides these aforementioned capsules based on hard colloidal particles, proteins have been utilized as modular building blocks for micron-sized shell formation, as well. In this respect, viruses – bionanoparticles constructed from proteins – were investigated42,238 by Russel et al. and reviewed239 by Böker et al. for their ability to assemble to colloidal capsules. First reports on albumin-based LbL-based microspheres date back several decades.240–242 However, the above discussed techniques were only recently implemented to prepare protein capsules with sophisticated shell properties. In first works, various groups combined proteins and a second component for electrostatic interactions in LbL synthesis routes to create protein shells.243–246 LbL-templating of proteins, which were subsequently cross-linked to form an intrinsically stable shell, were employed by Möhwald and co-workers. The authors first templated against MnCO3 particles and then added the dispersion to a glutaraldehyde solution to induce a reaction of its aldehyde groups with BSA amine groups. Cores could be dissolved by incubation in low molar HCl leading to hollow and 5 μm-sized capsules with BSA shells.247 Glutaraldehyde induced cross-linking was also employed for hemoglobin248 and glucose oxidase based capsules.249 A non-covalent linking method based on physical adsorption was later on developed by Mertz and Caruso et al., by first functionalizing silica templates with bromoisobutyramide, which subsequently acts as an intermolecular linker of adsorbed human serum albumin (HSA).250 HSA was further used in a follow-up study by the authors for the creation of protein shells (Fig. 11) and their biofunctionalization for cell viability experiments.127
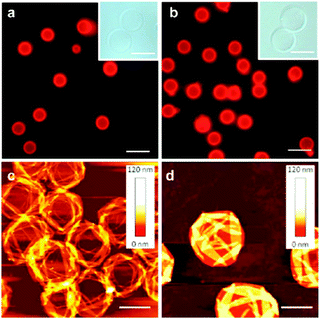 |
| Fig. 11 Fluorescence microscopy (a and b), bright field microscopy (insets) and AFM micrographs of template-based HSA-shelled capsules. (Scale bars: (a and b) 10 μm, (insets), 5 μm, (c and d) 2 μm). (Adapted with permission from ref. 127. Copyright (2012) American Chemical Society.) | |
Besides these LbL approaches, which are mainly derived from polymer chemistry, capsules with proteins as shell-forming building blocks were also created by various emulsion-based routes using proteins that more strongly resemble nanoparticles. For instance, ferritin was employed as a Pickering-emulsifier by Fujii et al.,251 and besides their work on bionanoparticles, Böker et al. also investigated ferritin–PNIPAAm conjugates as shell forming conjugates.252 Furthermore, ‘protein particles’ were also investigated for the stabilization of dextran/PEO-based water-in-water emulsions.253 Also by stabilizing all-aqueous emulsions, colloidal capsule-like structures were assembled from protein nanofibrils and termed as ‘fibrillosomes’.254 Another exceptional report was recently made by Mann et al. on protein–polymer based protocells. Here, the protein shells, termed as ‘proteinosomes’, were assembled from PNIPAAm-conjugated BSA at water-in-oil emulsion droplets prior to shell cross-linking.43 These proteinosome structures were further advanced in subsequent studies to feature enhanced shell functionality through a multivalent enzyme-based membrane255 that exhibits higher-order structure and function,48 and that possesses multiple subcompartments.256 In the latter report, the authors were able to create host–guest proteinosomes-in-proteinosomes (Fig. 12). Here, the release of encapsulated dextran and DNA from subcompartments was demonstrated. Some of these protein-based capsules represent a distinct class of protocells, which have also been created from inorganic particles in previous reports.
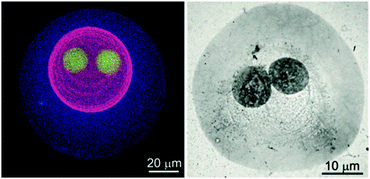 |
| Fig. 12 Proteinosomes-in-proteinosome: (left) proteinosome three levels of organization visualized with different fluorescent markers in a fluorescent microscope (right) TEM micrograph of a proteinosome with two levels of organization. (Reproduced with permission from ref. 256. Copyright 2016, Wiley-VCH Verlag GmbH & Co. KGaA.) | |
3.4 Inorganic protocells
Prior to their work on utilizing proteins as building blocks for the creation of structures with cell-precursive morphologies and functionalities (protocells),257 Mann et al. also published pioneering works on such structures based on inorganic nanoparticles.45,258 Initially, Li et al. encapsulated a wide variety of biomolecules in silica nanoparticle-based capsules. The resulting protocells were then used for in vitro protein synthesis. Beyond this, silica protocells with enhanced membrane functionality were created by grafting a pH-susceptible copolymer onto the membrane, giving rise to electrostatically gated permeability. In contrast to previous emulsion-based colloidal capsules which were usually solely able to inhibit diffusion of larger biomolecules out or into the protocells, these structures were able to preserve this functionality also for smaller molecules. This internal protocell functionality was then investigated for the performance of enzymatic dephosphorylation reactions.44 In a further study, cytoskeletal structural components were further mimicked with inorganic colloidal capsules via the integration of a supramolecular hydrogel within a protocell. The hydrogelation reaction was achieved by encapsulating a gel precursor (N-fluorenylmethylcarbonyl-tyrosine-(O)-phosphate) within the aqueous core of the capsules and a subsequent performance of an ALP-mediated removal of phosphate groups from the precursor.259 The authors also observed the formation of nanofilaments from the hydrogels which were further investigated in a subsequent study.260 A primitive cell-like division process of silica protocells was also invented by Mann and co-workers. Here, colloidal capsules containing a sodium phosphate buffer solution in a continuous oil phase were produced. Upon the addition of tetramethoxysilane (TMOS) a swelling inside the shells was induced due to methanol formation, leading to bud formation on the protocell surface. Auxiliary silica nanoparticles then assembled on the newly formed bud and a second generation micro-compartment was created (Fig. 13).261 Clay-based (montmorillonite) protocells with a temperature-responsive PNIPAM-based membrane were recently used for the establishment of simple cell-like signaling pathways,46 which resembles some similarities to works by Tamate et al. who used temperature responsive microgel particles to induce shape oscillation in protocells.47
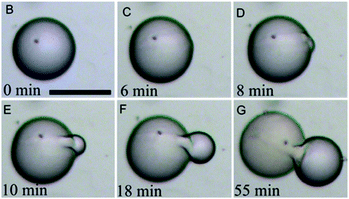 |
| Fig. 13 Self-reproducing colloidal capsule (inorganic protocell) based on silica nanoparticles. (Reproduced with permission from ref. 261. Copyright 2014, Wiley-VCH Verlag GmbH & Co. KGaA.) | |
3.5 Conclusions for micron-sized colloidal capsules
In summary, the use of particles with distinct morphological or physical properties in the shell of colloidal capsules may be effectively utilized to tailor the capsule's properties. However, the analysis of the capsules often tends to be qualitative and has therefore partially earned this field the rather negative tag of ‘pretty picture science’. Numerous standard colloid properties of the capsules are often only marginally discussed and their thorough characterization should be established as good practice in future studies within this field. These include, among others, the polydispersity and long-time colloidal stability of the capsules in relevant media, the interior build-up as well as thickness and number of monolayers of particles in the capsule's shell (e.g. by scanning TEM), membrane permeability, mechanical properties of the capsules (e.g. via AFM analysis), or the surface chemistry of the capsules itself as well as the shell-forming building blocks (e.g. via assays for quantification of functional surface groups).
4. Nano-scaled colloidal capsules
Miniaturization of encapsulation structures plays a pivotal role in their potential transfer from fundamental research objects to competitive products in technical, end-consumer or biological applications. All synthesis routes described in Section 2 have been utilized by various groups for the synthesis of nano-scaled capsules. However, whilst for micron-sized colloidal capsules we found a great majority of emulsion-based structures, for submicron-sized capsules only a few emulsion-based capsules haven been reported so far.
A decrease in capsule size inherently demands the employment of small or ultrasmall (<10 nm) nanoparticle building blocks. This specifically changes some circumstances for colloidal capsules aimed to be formed via the emulsion route. As described above, for the emulsion-route, the adsorption enthalpy of the particles is lowered with a decrease in particle size and tends to be lower compared to thermal particle motion, which might lead to the detachment of particles from the liquid–liquid interfaces of emulsion droplets.78 Additionally, several groups discuss the occurrence of so-called ‘image charge effects’ that prevent hydrophilic particles to absorb at the droplet interface, even though the net adsorption enthalpy is favourable.83,262,263 Furthermore, as recently described by Rotello et al.,16 a decrease of the emulsion droplet/capsule radius (r), which eventually leads to the formation of a nanoemulsion,264 goes along with an increase in Laplace pressure (ΔP) – the pressure difference between inside and outside the droplet – that is exercised onto the droplet interface:
As a result, nanoemulsion droplets tend to coalesce much faster. Hence, in contrast to larger emulsion-based colloidal capsules, where a structural stabilization can be more easily performed after the particles have adsorbed at the emulsion droplet interface, nano-scaled colloidal capsules require some form of accompanying in situ stabilization of the particles at the interface. However, a general theoretical description of the forces that influence the self-assembly of colloids at the nano-scale are more complex in comparison to the micron-scale as the interactions are not linearly additive and classical DLVO theory does not apply.265,266
Only a limited number of reports of emulsion-based colloidal capsules with sizes below 1 μm exist so far, including works by our group. We exploited a synergistic effect stemming from oil-soluble surfactants to decrease the capsule size, combined with a simultaneously occurring shell stabilization. Utilizing ultra-sound homogenization, we created a water-in-oil emulsion, with the water phase containing the shell-forming particles and the continuous oil phase containing oil-soluble surfactants (lipids). The lipids synergistically decreased the surface tension of the oil–water interface leading to nano-scaled droplet sizes and concurrently drove the nanoparticles to adsorb and to agglomerate at the interface. This synthesis route was based on previous observations of the formation of nanoparticle lipid thin films studied by us using interfacial shear rheology86 and by other groups using additional characterization methods.262,267 We performed the capsule synthesis with SiO2, Al2O3 and Al2O3-coated SiO2 core–shell nanoparticles268 (Fig. 14) as well as nanodiamonds35 in combination with either stearic acid or stearyl amine. Utilizing the same approach, we also formed submicron colloidal capsules from rhodamine fluorescent core–shell silica particles.30 Also using silica particles and surfactants, i.e. lecithin or oleylamine, Eskandar et al. prepared submicron-sized Pickering-emulsions. Inverse Pickering-emulsions with sizes below 500 nm and exceptional stability were also prepared by Ziener et al. by employing silica particles and in situ hydrophobizing the particles with oil-soluble surfactants.269 Earlier, Ziener and co-workers investigated another approach for the preparation of emulsion-based colloidal capsules. This was achieved by templating monomer droplets with silica particles followed by a temperature-induced polymerization of the core.17 Similarly, a combination of Pickering-emulsification and solvent displacement technique was performed for the synthesis of colloidal capsules. Here, silica particles were adsorbed on hexane droplets comprising poly(styrene-co-4-vinyl pyridine) copolymers, which phase-separated prior to the polymerization of the capsule's core.270 Khashab et al. adsorbed negatively and positively charged nitrophenylene-doped silica nanoparticles on the droplets of an oil-in-water emulsion leading to electrostatically stabilized Pickering-emulsions. Upon light-irradiation the charge of the positively charged particles could be reversed, leading to a destabilization of the shell and an eventual disassembly of the capsules, which could be combined with a time-dependent cargo release.271
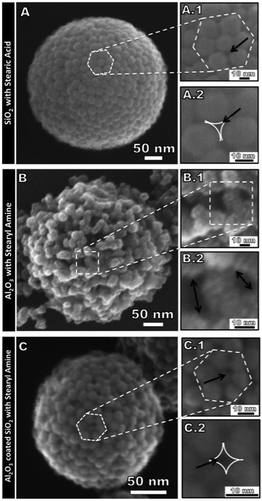 |
| Fig. 14 Submicron-sized colloidal capsules with SiO2, Al2O3, Al2O3-coated SiO2 particles based colloidal shells (Reprinted with permission from ref. 268. Copyright (2013) American Chemical Society.). | |
Another Pickering-emulsion approach combining a set of two types of particles (SiO2 and TiO2) was adopted by Wu and co-workers, where the core of the colloidal capsules was polymerized prior to dispersing the capsules in waterborne polysiloxane.272 In addition to these Pickering-emulsion-based colloidal silica shells, capsules from gold nanoparticles have been reported as well. As already introduced above, Rotello and co-workers used 2 nm sized gold nanoparticles for an oil-in-water emulsion based nanocapsule synthesis. Here, arginine-functionalized gold colloids adsorbed on linoleic acid droplets leading to a primary shell stabilization based on a distinct arginine–carboxylate interaction. Secondly, transferrin was introduced to the system leading to a further lateral stabilization of the colloidal shell through electrostatic attraction (see also Fig. 25 for an illustration of shell stabilization of a follow-up study).16
Non-emulsion based colloidal silica shells were also reported by various groups. In their early works Caruso et al. utilized their templating approach with polyelectrolytes and colloids in combination with silica and other inorganic nanoparticles.19,273–275 Herz et al. templated ZnS particles with fluorescent core–shell silica particles to reduce the fluorescent proximity quenching stemming from the energy transfer of closely neighboring fluorescent particles.29 Wu et al. created PMMA@SiO2 hybrid particles by templating silica sols on monomer templates prior to a polymerization step.276,277 Armes et al. synthesized various colloidal shells by templating silica sols against preformed monomer droplets with a subsequent core polymerization step.71,278–280 The packing patterns of silica and other particles templated against PS particles and polymersomes were analyzed by Bon and co-workers.281,282 Ritter et al. formed capsules from β-cyclodextrin and adamantyl-modified particles in an aqueous solution, which is assumed by the authors to occur due to supramolecular complexation.283 Zhou et al. assembled vesicles by physisorption of triblock copolymers on 15 nm silica particles, which showed surface reconstruction characteristics from a raspberry-like to a brain coral-like topography.284 Additionally, Guan et al. recently described colloidal capsules with varying morphologies using a templating approach.285
4.1 Distinct physical properties of building blocks and resulting nano-sized colloidal capsules
4.1.1 Nano-scaled colloidal capsules assembled from magnetic nanoparticles.
Based on their original templating approach, Caruso et al. adsorbed Fe3O4 nanoparticles and polyelectrolytes on PS-core templates, with that pioneering the field of nano-scaled magnetic colloidal capsules.286 By varying the number of coating layers from one to five, after subsequent etching of the PS core, the diameters of the hollow capsules could be adjusted to be between 650 and 960 nm.287 Maceira and co-workers also used a templating approach with polyelectrolytes, although they templated against SiO2 particles and formed the magnetic colloidal shell (cobalt nanoparticles) by an in situ seed formation process.288 Based on our previously discussed Pickering-emulsion approach in combination with lipids, we likewise synthesized colloidal capsules from iron oxide nanoparticle with submicron sizes. Characterization of the structures with an alternating gradient magnetometer revealed that the capsules maintained their superparamagnetic properties. The capsules could be affected by an external magnetic field (Fig. 15).30 Another emulsion-based method to stabilize the shell of magnetic particle enclosed capsules was employed by Shen et al. who silanized a Fe3O4 Pickering-emulsion. The final structures also maintained their superparamagnetic properties.289
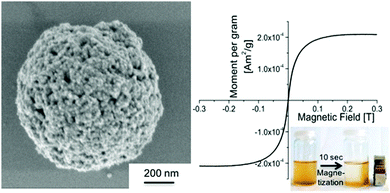 |
| Fig. 15 Submicron-sized colloidal capsule formed from iron oxide nanoparticles featuring superparamagnetic properties. (Reproduced with permission from ref. 30. Copyright 2015, Wiley-VCH Verlag GmbH & Co. KGaA.) | |
We already briefly discussed some magneto-liposome/polymersome structures in Section 2.4. Recent work by Park et al. is worth further noticing, describing the size control of magneto-polymersomes. The authors loaded magneto-polymersomes with iron oxide particles of different sizes through which they were able to control the size of the resulting submicrometer polymersomes. The vesicles were formed by first mixing poly(acrylic acid)–polystyrene block copolymers with iron oxide particles in a dioxane and THF mixture and subsequently adding water to induce vesicle formation. TEM-tomography was utilized for a detailed morphological characterization of the polymersomes (Fig. 16).199 The structures reportedly featured high transverse relaxation rates in analogy to a previous report about magneto-polymersomes by Sandre and co-workers.290
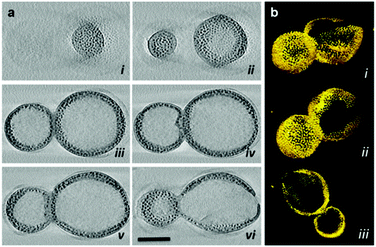 |
| Fig. 16 Magneto-polymersome (a) TEM tomography micrographs and (b) surface renderings (Adapted with permission from ref. 199. Copyright (2014) American Chemical Society.). | |
4.1.2 Nano-scaled colloidal capsules assembled from gold nanoparticles.
In the past years, an especially strong focus on the utilization of gold nanoparticles to study interparticle surface plasmon interactions has emerged.291,292 The surface plasmon resonance of gold nanoparticles is extremely sensitive to changes in the local environment surrounding the particles and to the interparticle distances, making gold particles specifically interesting for nano-scaled colloidal capsule formation.293 Again, based on a hard templating approach with PS particle cores, Caruso et al. were the first to report submicron colloidal capsules formed from Au nanoparticles and with that pioneered the field of plasmonic coupling in colloidal submicron capsules. UV-visible spectra of the capsules displayed a plasmon resonance peak of 655 nm in comparison to the gold nanoparticle dispersion of 517 nm.294
Following up on this report, more templating approaches have been used to further tailor the resulting capsule properties. After earlier reports of densely13 nanoparticle-coated micron-sized and loosely295 coated submicron-sized microgels, Karg et al. reported a high density colloidal gold shell adsorbed on a thermoresponsive poly-(NIPAM-co-allylacetic acid) microgel. Based on electrostatic interactions a swollen microgel template was covered with PSS/PAH coated gold nanorods (57 nm in length and 15 nm in diameter). Upon a temperature increase the microgel template shrunk from approx. 700 nm at 15 °C to 270 nm at 60 °C, leading to dense colloidal particle surface coverage. The decrease in microgel size inherently led to a decrease of the separation distances of the nanorods, causing a red-shift of the UV-vis spectra band induced by surface plasmon interaction (Fig. 17).37 Another report utilizing gold colloids and a hard template without the use of polyelectrolytes was recently made by Landon et al. designing ‘hollow golf nano balls’. The authors first attached 100 nm PS particles to a larger SiO2 template and subsequently adsorbed 3 nm gold particles on the SiO2@PS template. Followed by a plating process of the gold particles and removal of the SiO2@PS template resulted in the formation of a partially fused gold colloid surface with a morphology resembling a golf ball.296 Another distinct linking approach of a colloidal particle gold shell template on silica particles was reported by Liu and co-workers. The authors adsorbed negatively charged bis(p-sulfonatophenyl)phenyl phosphine capped 14 nm gold particles on an aminated SiO2 template. A subsequent treatment of the structures with Ag+ led to covalent interconnections between the gold particles, which was performed prior to HF etching of the silica core.14 Besides the works of Rotello et al., which we will discuss in more detail in Section 5, only a few reports of emulsion-based submicron-sized colloidal gold particle shells could be found, including a report by Tian et al. who assembled amphiphilic gold particles on the droplet interface of a toluene-in-water emulsion.297
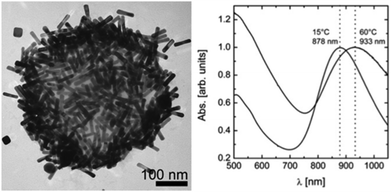 |
| Fig. 17 Left: Micrograph of a gold nanorod-covered PNIPAm microgel particle; right: UV-vis spectra of a swollen (15 °C) and collapsed microgel (60 °C) (Adapted with permission from ref. 37. Copyright (2009) American Chemical Society.). | |
4.1.2.1 Nano-scaled colloidal capsules assembled from polymer-grafted gold nanoparticles.
As already mentioned above, a great number of reports of capsules built from colloidal gold particles are based on the amphiphilicity-driven self-assembly of polymer-brush-conjugated gold particles. In advance to the first works on the vesicle forming capabilities of gold colloids with a mixed homopolymer architecture, Duan et al. investigated the adsorption kinetics of PEG and PMMA conjugated nanoparticles at the oil–water interface and were able to reversibly tune the assembly of the particles at the interface by the addition of different solvents to the system.298 The nanoparticles were synthesized via a dual grafting-“To” and -“From” route, by first simultaneously bonding the hydrophilic polymers to the particle, in combination with an initiator species for a subsequent grafting-“From” step performed by atomic transfer radical polymerization (ATRP). On a side-note, polymer brush growth from various types of nanoparticles was recently reviewed by Böker and co-workers.299
Based on 14 nm gold particles grafted with a dual homopolymer architecture consisting of PEG and PMMA brushes (Au@PEG/PMMA) Song et al. created approx. 200 nm sized nanoparticle vesicles via a film rehydration method (Fig. 18). UV-vis spectra showed a 30 nm red shift of the vesicles in comparison to the individually dispersed Au@PEG/PMMA particles, indicating a surface plasmon coupling of the gold particles. The authors also studied the pH-responsive disassembly of the vesicles by introducing 25% 4-vinylpyridine (4VP) into the PMMA chain, allowing a controlled degradation of the vesicles upon shifting the pH from 7 to 5.49 The intracellular drug delivery potential of these Au@PEG/PMMAVP152 and photo-regulated Au@PEG/PNBA153 vesicles were investigated in follow-up studies, as reviewed in Section 5. The vesicles were further advanced by the substitution of the spherical particles with nanorod gold colloids. By changing the initiator species, Song et al. were able to conduct an organocatalytic surface-initiated ring-opening polymerization of lactic acid to retrieve PEG and polylactide mediated gold nanorods (AuNR@PEG/PLA). After vesicle formation, the PLA allowed for an enzymatic disruption of the shell via treatment with proteinase K as well as a rupturing by heating the vesicles, which was induced by a 808 nm laser.154 Recently, Song et al. simplified the gold particle functionalization by solely applying a grafting-“To” step for the fixation of mixed homopolymers on the particles. Individual PEG and PLGA polymer-chains with thiol end-capping were simultaneously reacted with CTAB-stabilized gold nanorods, eliminating the need for the rather laborious combination of grafting-“To” and grafting-“From” steps. The resulting Au@PEG/PLGA particles could be combined with reduced graphene oxide for an w/o/w-based synthesis of approx. 65 nm sized NP vesicles.155 In a follow-up study, also based on an emulsion-route and employing polyvinyl alcohol as an emulsion stabilizer, the sizes of the Au@PEG/PLGA based vesicles were adjusted to ∼60 nm.52 Deng an co-workers applied a similar approach and conjugated 14 nm Au NP with pre-synthesized PCL-SH and PMEO2MA-SH grafts.300 The further miniaturization of these NP vesicles with the combination of biodegradable polymers makes these structures of specific interest for biomedical applications which will be discussed in more detail in Section 5.
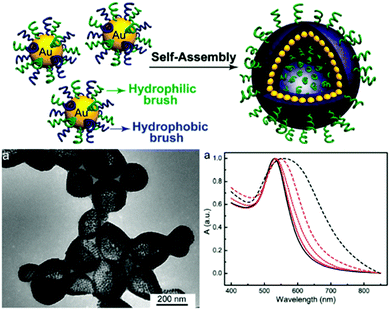 |
| Fig. 18 (top) Mixed PEG-PMMA grafting architecture on gold nanoparticles and resulting nanoparticle vesicle; (bottom-left) TEM micrograph of vesicles; (bottom-right) UV-vis spectra of Au@PEG/PMMA dispersion and respective colloidal capsules (native Au@PEG/PMMA dispersion in CHCl3 (black solid line), in H2O: vesicles of Au@PEG/PMMA (black dashed line), Au@PEG/PMMAVP with 25% 4VP (red solid line), vesicles at pH 7.0 (red dashed line), disassembled vesicles (red dot line)) (Adapted with permission from ref. 49. Copyright (2011) American Chemical Society.). | |
As mentioned in Section 2.3, Nie et al. utilized a BCP polymer-brush architecture for colloidal capsule synthesis. In their initial work, the authors reported a strong dependence of the possibility to form NP vesicles on the hydrophobic block length and particle size. Larger particles featuring a diameter of 8 nm or larger only formed vesicles if the PS block length had a mass of 10
000 g mol−1 or more. In other words, vesicle assembly can only be achieved if the chain length of the polymer is at least in the size range of the nanoparticle diameter. The resulting vesicles featured distinct interparticle spacings between the gold particles which are determined by the polymer block length (Fig. 19).15 These and further vesicles based on nanoflower-shaped Au particles145 influenced the resulting UV-vis absorption spectra. Au NP designed with different lengths of the PS block were further probed for the assembly in THF:water mixtures with different volumetric ratios.144 In another study, the photosensitizer chlorine e6 (Ce6) was loaded into PEO45-b-PS245 grafted Au NP Vesicles with final sizes of ∼280 nm. Furthermore, NP vesicles with enhanced biocompatibility featuring PCL as the hydrophobic block and a high photothermal conversion efficiency were assembled from 26 nm sized Au NPs grafted with PEO45-b-PCL270 BCPs. The resulting vesicles featured narrow size distributions of 192.6 ± 11.8 nm or 207.3 ± 15.7 nm, depending on the Au@PEO-b-PCL concentration at the start of the synthesis. Degradation of the vesicles could be observed by either increasing the temperature or after a prolonged period of time of 8 weeks at a constant temperature of 37 °C.50 The surfactant-like characteristics of amphiphilic polymer-tethered nanoparticles were recently reviewed by Zhang and Zhao.301
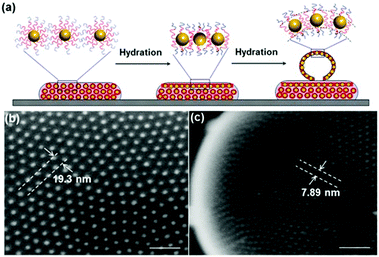 |
| Fig. 19 (a) Scheme of the association of the hydrophobic block of BCPs during vesicle self-assembly; (b and c) SEM images of discrete spacings between AuNP on vesicle surface. scale bars, 100 nm. (Copied with permission from ref. 15. Copyright (2012) American Chemical Society.) | |
Vesicles from block-oligomer-tethered gold nanoparticles with shorter hydrophilic and hydrophobic units were reported by Niikura and co-workers. Here, gold nanoparticles with a citric acid coating were functionalized with a ligand of intermediate length, comprising an oligo(ethylene glycol) (OEG) group, a fluorinated tetraethylene glycol (FG) group, and a 11-carbon alkyl subunit. The resulting 60 nm sized vesicles exhibited surface plasmon coupling characteristics, which was expressed in a 30 nm red shift in the UV-vis adsorption spectra.302 In a follow-up study, the sub-100 nm vesicles were probed for drug-encapsulation and light-triggered release applications.303 An unprecedented ‘entropy-driven size segregation effect’ was reported by Niikura et al. when combining citric-acid coated gold nanoparticles with different sizes with a glucose-terminated FG-11-carbon alkyl ligand. The particles of different sizes first aggregated and then transferred to a yolk/shell build-up (Fig. 20).304 Another phase separation effect was also reported by Nie et al., observed during the coassembly of BCP-tethered Au NPs with free BCPs, leading to Janus-like colloidal shells. Au NP tethered with BCPs with different PS and PEO block lengths were concurrently assembled with ‘free’ BCPs, which also comprised different PS and PEO lengths. The Janus-like vesicles included spherical, hemispherical and disk-like shapes (Fig. 21).201 Rasch et al. observed an analogous phase separation effect in their nanoparticle–liposome hybrids utilizing dodecanethiol-coated Au colloids, which we already briefly discussed in Section 2.4. The authors reported the assembly of spherical Janus-like nanoparticle-loaded liposomes based on a dialysis route (Fig. 22A and B). Here, the formation of a Janus-like vesicle morphology is associated with a clustering of the hydrophobic particles in the lipid bilayer. When the particles are confined, the bilayer must first ‘unzip’ which creates small gaps around the particles, which are eventually reduced upon clustering of particles.186 Other liposomes with gold nanoparticles that were packed rather sparsely inside the bilayer were reported by An and co-workers. Here, the liposomes were probed for a photo-induced drug release by local membrane heating which changed the membrane permeability.305 A different approach was recently reported by Nakamura et al. who adsorbed gold nanoparticles on a vesicle based on fullerene amphiphiles (R5C60−K+). Au NP approx. sized 3.5 nm and comprising (11-mercaptoundecyl)tetra(ethylene glycol) ligands were conjugated to potassium ions on the surface of the fullerenes. The Au NP served a seed and could be further grown to sizes of approx. 7 nm leading to an increased surface coverage.306
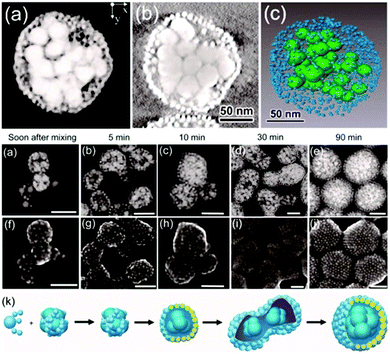 |
| Fig. 20 Egg/yolk like size-segregated core–shell Au nanoparticle vesicles. Top: STEM tomography images of a 5 nm NP based shell and 30 nm Np based core (a) 3D reconstruction; (b) sliced XY image; (c) 3D representation. Bottom: Time evolution segregation of differently sized Au nanoparticles (Adapted with permission from ref. 304. Copyright (2016) American Chemical Society.). | |
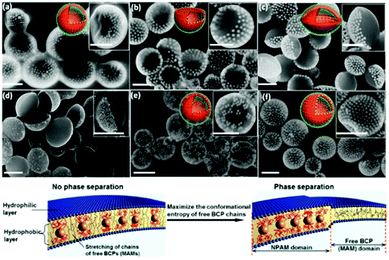 |
| Fig. 21 Top: SEM micrographs of Janus-like nanoparticle vesicles observed in the concurrent assembly of PEO-b-PS BCP tethered Au NP with “free“ PEO-b-PS BCPs with (a) spherical, (b) hemispherical, (c and d) disk-like shapes; and non-Janus-like vesicles with (e) patchy and (f) heterogenous morphology. Bottom: Representation of the phase separation effect within the free BCP bilayer attributed to an entropy-driven attraction of the BCP-tethered Au Np (Adapted with permission from ref. 201. Copyright (2014) American Chemical Society.). | |
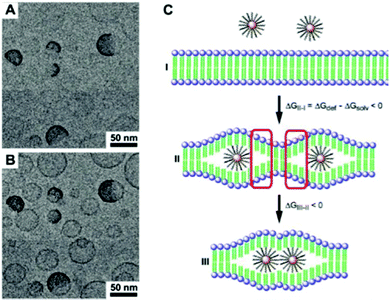 |
| Fig. 22 (A and B) TEM images of spherical Janus-like liposome–nanoparticle hybrids. (C) The gold colloids are confined in the hydrophobic part of the liposome by first “unzipping” the bilayer membrane and secondly clustering to reduce the sizes of so-called “strained regions”. (Adapted with permission from ref. 186. Copyright (2010) American Chemical Society.) | |
4.1.3 Nano-scaled colloidal capsules assembled from multiple modular building blocks.
Based on our above discussed lipid-employing emulsion route for concurrent shell stabilization and reduction of capsule size, we reported the co-assembly of different nanoparticle types to create multivalent submicron-sized capsules. We used fluorophore-doped (rhodamine B) silica and superparamagnetic iron oxide nanoparticles in combination with stearic acid for our water-in-oil submicron capsule synthesis. The resulting colloidal shells maintained the distinct physical properties of the modular building blocks (Fig. 23).30 Similarly, so-called supernanoparticles featuring magneto-fluorescent properties were described by Chen et al. who were able to create core–shell clusters featuring a core of magnetic nanoparticles and a shell with a monolayer of quantum dots.31
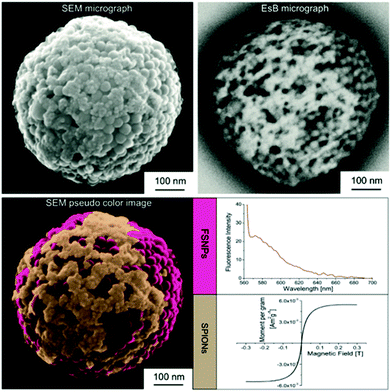 |
| Fig. 23 Bifunctional submicron colloidal capsule concurrently assembled from fluorescent silica and iron oxide particles, comprising fluorescent and superparamagnetic properties. (Reproduced with permission ref. 30. Copyright 2015, Wiley-VCH Verlag GmbH & Co. KGaA.) | |
Rotello et al. synthesized multivalent colloidal capsules based on a delicate interplay of electrostatically and covalently interconnected modular building blocks adsorbed on fatty acid templates dispersed in water. The so-called nanoparticle-stabilized capsules were used for fluorescence resonance energy transfer (FRET) studies, by the incorporation of quantum dots (QD) and fluorescent proteins in the capsule's shell. Green-emitting QDs (CdSe/ZnS core–shell particles) were first surface-grafted with dihydrolipoic acid and then functionalized with a polyhistidine-conjugated red fluorescent protein via metal affinity coordination chemistry. These protein–QD conjugates were then adsorbed onto the surface of Au NP containing fatty acid droplets (linoleic acid and decanoic acid) leading to final capsule sizes of 120 ± 50 nm. The capsules further featured stimuli-responsive release of the hexahistidine-tagged red fluorescent protein which will be further discussed in Section 5.2.307 As briefly mentioned in Section 2.4.2 Nie et al. combined free PS107-b-PAA4 BCPs with 20, 30, or 50 nm-sized PS490-b-PEO45-grafted Au NP and 15 or 25 nm-sized oleic acid-capped Fe3O4 NP for the synthesis of multivalent janus vesicles which featured sizes of approx. 570 nm. The authors reported the formation of vesicles with either spherical or hemispherical morphology, similar to their previously described structures illustrated in Fig. 21. These so-called ‘magneto plasmonic janus vesicles’ exhibited strong near-infrared absorption, as well as an enhanced transverse relaxation (T2) contrast effect due to the dense confinement of the particles in the vesicle membrane. The authors examined the structures as light- and magnetic field-responsive containers for triggered release of a model drug and as contrast agents for different bioimaging techniques (see Section 5.3 for more details on their in vivo experiments).202
4.2 Nano-scaled colloidal capsules assembled from proteins or bionanoparticles as building blocks
In 1999, Caruso and Möhwald published the first work of protein (BSA and IgG) and polymer-templated PS submicron-sized particles,308 followed by further reports utilizing different enzymes, i.e. horseradish peroxidase, β-glucosidase, or urease for the templating process.309–312 Following these initial reports and their above described template-based synthesis of micron-sized capsules with a protein shell, Caruso and Mertz et al. further adjusted their route for the synthesis of capsules with diameters between 50 and 150 nm with a human serum albumin (HSA) shell. The HSA proteins were templated against siRNA loaded isobutyramide-mediated mesoporous silica particles (MSNP) (Fig. 24) and subsequently the MSNP core could be removed by incubating the structures in a buffered HF solution.129 Viruses, which can be interpreted as protein-based bionanoparticles, were used by Wang et al. in combination with poly(4-vinyl-pyridine) (P4VP) for the synthesis of colloidal capsules. P4VP in DMF was slowly added to an aqueous solution of spherically shaped cowpea mosaic viruses (CPMV) or rod-like tobacco mosaic viruses (TMV), leading to a solvent change induced assembly of close-packed virus-enclosed capsules.313
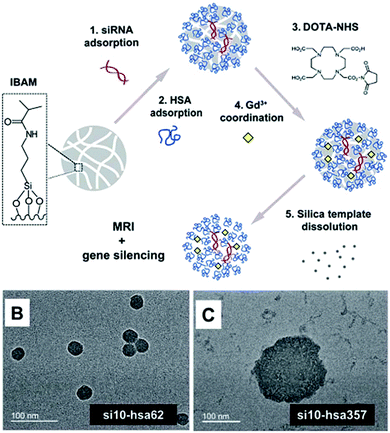 |
| Fig. 24 Top: Synthesis route of gadolinium-mediated and siRNA loaded human serum albumin capsules; bottom: TEM micrographs of differently sized HSA capsules. (From ref. 129. Reproduced with permission from The Royal Society of Chemistry.) | |
4.3 Conclusions for nano-scaled colloidal capsules
In the last decade a number of novel and promising nano-scaled colloidal capsules have been reported. However, similar conclusions for nano-scaled colloidal capsules hold true as the ones briefly discussed above for micron-sized capsules (Section 3.5). Here, likewise, a good practice of the characterization of the capsules needs to be established, even though visualization of the nano-scaled capsules can so far only be performed ex situ after their preparation for electron microscopy studies. Additionally, a complete mechanistic understanding of the varying assembly phenomena that occur at the nano-scale remain not fully investigated; such as the kinetics of formation of colloid thin-films on nano-emulsion droplets and their mechanical and rheological properties, deeper knowledge of the delicate interplay of colloid size, length of the hydrophilic and hydrophobic units, grafting density, and the resulting tendency of polymer-grafted particles to form capsules with defined sizes, notwithstanding the often unintuitive and poorly understood colloidal behaviour of small and ultrasmall nanoparticles.265 Detailed knowledge of these and other factors could allow for the phrasing of simple design rules which could then lead this field closer to a knowledge-based design of these structures. Furthermore, the establishment of a gold standard synthesis route that would allow a reproducible formation of highly monodisperse samples with a high yield, analogous to the now ubiquitous microfluidic based synthesis of micron-sized capsules, is needed to advance nano-scaled colloidal capsules into a mature field of science in the near future.
5. Colloidal capsules for biological applications
A great number of the above described colloidal capsules are developed to be used in bionanotechnology, like biosensing, bioimaging or targeted drug delivery. With silica314–317 (e.g. C·spec®), quantum dots318,319 (e.g. EviTag®), gold320 (e.g. Aurimmune, AuroVist™), iron oxide321–324 (e.g. Feraheme™, NanoTherm®, Combidex®, Feridex®/Endorem®, Resovist®/Ferucarbotran) and other (ultrasmall325) nanoparticles either being in (pre-)clinical trials or FDA-approved and (temporarily) in clinical use, utilization of these material classes, as modular building blocks for colloidal capsule self-assembly, could open new design routes in the synthesis of multivalent nanomedical agents.326 First in vitro studies in different cell lines and in vivo experiments in rodent models have very recently been performed with colloidal capsules and represent promising approaches and possible competitors to e.g. amphiphile-based vesicles. Numerous guidelines and considerations need to be taken into account for drug-carrier design, which makes the synthesis of colloidal capsules formed from the above mentioned inorganic particles significantly more complex in comparison to their organic vesicle counterparts, i.e. liposomes and polymersomes. These considerations include a thorough and standardized nanosafety assessment of the individual nanoparticle building blocks and the assemblies as a whole.327–329 In many of the studies discussed in the following paragraphs, considerations for the design of nanomedical agents have been applied in the synthesis of colloidal capsules for in vivo experiments in varying degrees. Aspects of the biological fate of colloidal capsules have been characterized as well in some studies. However, this novel field of bionanotechnology is far from presenting a unified approach towards nanosafety or biomedical design rules which would greatly facilitate the development of advanced nanocarrier systems.
Nevertheless, the self-assembly of nanoparticles to colloidal capsules enables the synthesis of novel nanoprobes whose functionalities can be tailored through the collective properties of the shell-forming particles. The integration of superparamagnetic, plasmonic, fluorescent or further functional colloids with distinct physical properties into the shell of colloidal capsules allows the creation of synergies for applications such as multimodal imaging, photo-/hyperthermal treatments, or targeted drug delivery.326 Furthermore, such capsules may solve some of the problems larger individual inorganic nanoparticles face in bionanotechnology. The potential to design colloidal capsules with the ability to extra- or intra-cellularly erode into their individual building blocks and then follow distinct clearance pathways330,331 (e.g. renal clearance) imparts them with a great advantage over non-degradable larger inorganic nanoparticles which the human organism is not capable to metabolize and consequently cause great concern regarding long-term toxicity.332 Additionally, by using ultrasmall nanoparticles to create colloidal capsules with diameters of approx. 100 nm or smaller they can synergistically inhibit accumulation within the mononuclear phagocyte system and passively target tumors by utilizing the enhanced permeability and retention (EPR) effect.333 Another benefit of the degradable shell of colloidal capsules is their potential ability to deliver active pharmaceutical agents (i.e. chemotherapeutics, siRNA, etc.) directly to the cytosol by membrane fusion and by avoiding endosomal sequestration. Hence, precise combinations of size, surface moieties and controlled degradation behavior into their shell-forming individual building blocks with complete clearance will be central for the potential bench-to-bedside translation of colloidal capsules for parenteral/intravenous administration.
As briefly described above, gold NP are of high interest for colloidal capsule formation as the architectural build-up of the shell can have significant impact on their surface plasmon resonance (SPR), which can be tailored through particle size and interparticle spacing. Interparticle plasmonic coupling results in a shift of the SPR band towards longer wavelengths (red shift) of the near-infrared range (NIR). This is highly desirable for in vivo applications, as NIR light is attenuated less by biological tissues than visible light.334 This can be efficiently exploited for imaging and therapy applications as plasmonic (supra)particles convert light strongly at SPR wavelengths.293 As discussed throughout the following sections, colloidal capsules assembled from Au NP can specifically be used in photothermal and photodynamic therapy,335 photothermal and photoacoustic imaging,336 as well as remote controlled release of encapsulated drugs.326 Similarly, colloidal capsules and other supraparticles assembled from superparamagnetic nanoparticles can potentially be tailored to feature distinct magnetic properties that distinguish them from larger individual iron oxide nanoparticles. Here, high r2 transverse relaxivity values to potentially enhance the image contrast in T2-weighted magnetic resonance imaging (MRI), a high specific absorption rate (SAR) for heat generation, and high magnetic responsiveness to allow such colloidal structures to be moved via an external magnetic field, are desired.54 These properties would allow colloidal capsules formed from superparamagnetic colloids to be utilized in applications such as magnetic fluid hyperthermia337 and hyperthermic drug release, magnetic particle imaging,338,339 and magnetic resonance imaging.340
We will first briefly review micron-sized colloidal capsules that were designed for biological applications. Subsequently, in vitro and in vivo studies will be reviewed in detail, describing the unique properties of colloidal capsules within this field. Finally, we will close this section with a critical discussion on whether the here described capsules should be considered for in vivo applications in the near future.
5.1 Micron-sized colloidal capsules for biological applications
A certain number of micron-sized colloidal capsules have been reported which do not have to deal with the hassles that are faced in the design of nanocarriers as they are almost solely aiming at non-human ex vivo applications. For instance, micron-sized colloidal capsules have been investigated to retain enzyme activity for improved enzymatic catalytic performance, including reports for amylase,341 laccase,342 CalB,40 lipase A, or benzaldehyde lyase.22 Furthermore, colloidal capsules were investigated to incorporate various different hydrophobic cores.343
For example, Porta and Kros examined mesoporous silica nanoparticle-based capsules with a polyacrylamide core for the local delivery of hydrophobic all-trans retinoic acid (ATRA), also called tretinoin, in zebrafish. The large colloidal capsules with diameters of approx. 100 μm could be handled with tweezers and were implanted into the caudal fin of the zebrafish. The authors observed a delay in tail regeneration from the zebra fish due to the release of ATRA from the capsules.344
5.2
In vitro studies of nano-scaled colloidal capsules
We will start discussing nano-scaled colloidal capsules for bionanotechnology with reports on in vitro studies. As mentioned above, Rotello and co-workers have published a series of reports of colloidal capsules for biological applications. In their initial report, the authors encapsulated paclitaxel in the hydrophobic linoleic acid core of transferrin and arginine–Au NP stabilized nanocapsules. The drug-delivery capabilities of these paclitaxel-loaded capsules were probed on HeLa cells and proved to be effective by exhibiting a half-maximal inhibitory concentration (IC50) of 13.5 ng mL−1 after 24 h of incubation. Control experiments showed no carrier toxicity.16 Nanocapsules formed from a combination of His-Lys-Arg-Lys-capped gold nanoparticles (“Au_HKRK” NP), histidine6-tagged red fluorescent protein “mCherry” and dihydrolipoic acid-capped CdSe/ZnS core–shell particles (“DHLA_QD”) were used for cellular studies, as well. The emission–adsorption overlap of the QD and mCherry spectra enabled FRET studies. The nanocapsules formed from the aforementioned components were incubated with HeLa cells and were taken up by the respective cells via endocytosis. Here, histidine and chloroquine could be utilized as disrupting and releasing agents of the mCherry proteins from the endosomes/lysosomes into the cytosol.307 A process resembling cell membrane fusion for the delivery of proteins and small molecule drugs was investigated in several studies as well by Rotello and co-workers. Au NP-stabilized nanocapsules were utilized for the delivery of protein (GFP, green fluorescent protein) and enzyme (caspase-3) into the cytosol of HeLa cells (Fig. 25). With GFP being a protein imaging agent and caspase-3 a therapeutic enzyme, the protein stabilized NP capsules exhibited multivalent functionality. Caspase-3 was reported to be effectively delivered, leading to the induction of apoptosis, whilst the GFP allowed for a monitoring of its intracellular delivery.21 In another report, again avoiding endosomal sequestration, the proposed cell-membrane fusion process was used for the delivery of small interfering RNA (siRNA) directly to the cytosol, which can be favorable over endocytic routes which were previously described for other nanocarriers. Electrostatically stabilized complexes consisting of siRNA and arginine-functionalized Au NP were again confined on the surface of linoleic acid oil-in-water emulsion droplets. The transfected siRNA allowed for a very high knockdown of green fluorescence protein (deGFP) in deGFP-HEK293 cells. Furthermore, the delivery of a different siRNA type allowed for silencing of polo-like kinase 1 (PLK1), a kinase enzyme, possessing cell cycle regulation properties and often overexpressed in tumor cells. The siRNA was efficiently protected against nuclease degradation. The membrane fusion mediated cytosolic release of siRNA was evidenced in various control experiments.51 In a subsequent report, Rotello et al. developed a novel method to monitor the trafficking of proteins from nanocapsules to the nucleus. Different nuclear localization signal (NLS) sequences were reportedly used to track the transfer to the nucleus. Enhanced green fluorescent proteins (eGFP) were utilized to function as a fluorescent reporter protein, which was again delivered via the membrane-fusion route (Fig. 26a). Among the different NLS sequences, listed in Fig. 26b, NLSc-Myc-eGFP exhibited the most significant nuclear accumulation ability (Fig. 26c and d). Furthermore, the cytosol delivery of NLSc-Myc-eGFP was monitored via live cell video imaging, revealing a significant delivery to the cytosol after 60 s and laser scanning confocal microscopy reportedly showed the establishment within the nucleus after 6 min.345 The co-delivery of nanocapsules containing both a proapoptotic protein and paclitaxel as a antimitotic agent was reported as well. Here, paclitaxel was first incorporated in linoleic acid emulsion droplets, followed by the adsorption of arginine-conjugated Au NP on the droplet surface and by an adsorption of negatively charged proteins on the surface of the nanocapsules. The utilized proteins, transferrin and CASP3, were used as non-active control and therapeutic agents. HeLa cell viability was measured by incubation with “NPSC-PTX-CASP3” nanocapsules. These dual-cargo containing nanocapsules exhibited substantially higher efficiency to induce apoptosis in comparison to free paclitaxel and unloaded capsules, consisting of Au NP with either paclitaxel or one of the proteins.346 Dual-cargo siRNA and tamoxifen loaded colloidal capsules347 as well as colloidal capsules for the direct cytosolic delivery of larger proteins, again avoiding endosomal entrapment,348 were also investigated by Rotello et al. in other studies. A recent report by Rotello et al. of dendrimer–Au NP based colloidal capsules will be discussed in the following in vivo section.
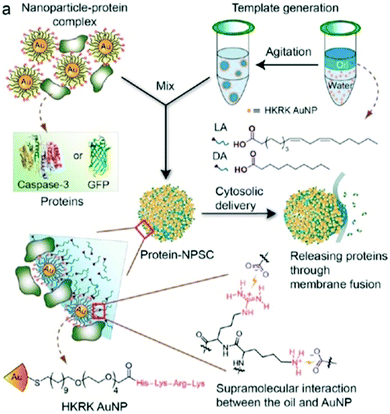 |
| Fig. 25 Scheme of Au NP and protein stabilized colloidal nano-capsules, which were utilized for a membrane fusion based release of proteins directly into a cell's cytosol. (Adapted with permission from ref. 21. Copyright (2013) American Chemical Society.) | |
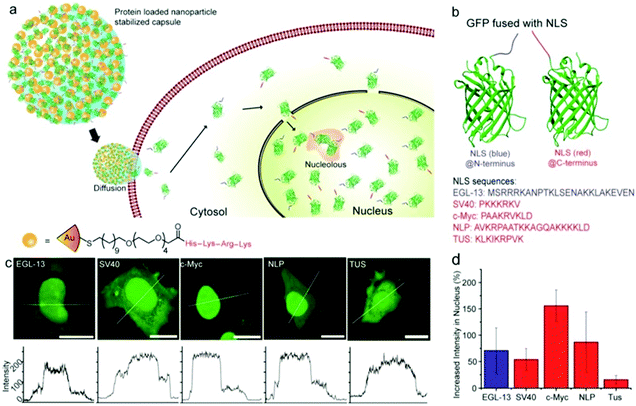 |
| Fig. 26 (a) Scheme of Au NP and eGFP stabilized nanocapsules and their membrane fusion based Protein release; (b) eGFP structure with nuclear localization signal sequences; (c) different cell images fused with eGFP (scale bar: 20 μm); (d) increase of nuclear intensities of different NLS-eGFPs (Copied with permission from ref. 346. Copyright (2015) American Chemical Society.). | |
Song et al. also performed various in vitro studies with colloidal capsules formed from Au nanoparticles with a mixed homopolymer architecture. These colloidal capsules with enhanced biocompatibility were synthesized via conjugation with a cell-membrane targeting antibody (HER2 protein), and a pH-susceptible dissolution trigger, that makes use of the acidic environment of intracellular compartments (Fig. 27). The nanoparticle vesicles formed from PEG- and PMMAVP-grafted 14 nm gold particles could be loaded with doxorubicin and were aimed at drug-delivery via an endocytic pathway. Additionally, the capsules were conjugated with a Raman reporter (BGLA), which enabled the identification of the disassembly of the capsules by monitoring the surface enhanced Raman scattering (SERS) signal via Raman spectroscopy. The authors successfully targeted HER2-positive SKBR-3 breast cancer cells with the antibody-tagged capsules, whilst this receptor-mediated targeting was evidenced in control experiments with HER2-negative MCF-7 breast cancer cells, which showed no specific binding of the capsules. Additionally, doxorubicin, deprotonated with triethylamine, was encapsulated by first forming a hybrid thin-film of doxorubicin and Au@PEG/PMMAVP4 particles on the wall of a small vial, and then rehydrating the film and by that inducing the nanoparticle vesicle formation. The release of doxorubicin from the capsules showed a strong pH-dependence.152 Very similar nanoparticle vesicles featuring a photo-responsive disassembly trigger were synthesized by utilizing poly(2-nitrobenzyl acrylate) (PNBA) as the hydrophobic brush which can be reacted to water-soluble poly(acrylic acid) after light irradiation, inducing capsule erosion to single particles. In accordance to the aforementioned Au@PEG/PMMAVP4 based vesicles, the capsules also featured a plasmon coupling red-shift, which could be further controlled by varying the PEG/PNBA ratio to 1
:
1, 1
:
2, and 1
:
4, leading to localized surface plasmonic resonance peaks of 550, 630, and 745 nm respectively. Folate was conjugated to the capsules as well, to serve as a moiety to target the folate receptor, which is overexpressed in numerous cancer types, including MDA-MB-435 breast cancer cells. In comparison to nanoparticle vesicles without folate ligands, the conjugated vesicles reportedly showed greatly enhanced binding to the cells, which was qualitatively observed by the authors via dark-field microscopy imaging. The encapsulation and release of doxorubicin was also investigated for the capsules on MDA-MB-435 cells.153 Nanoparticle vesicles from PLA and PEG conjugated gold nanorods were also investigated by Song and co-workers. The Raman reporter carrying colloidal capsules featured distinct SERS signals, potentially enabling their use for Raman imaging and spectroscopy. Proteinase K, a protein used for the enzymatic degradation of PLA, was used for the degradation and controlled erosion of the PLA capsules, leading to the release of encapsulated doxorubicin. The capsules were further investigated in in vitro studies by demonstrating the targeting of EpCAM-positive Hep3B cancer cells with monoclonal antibodies, in comparison to antibody-free capsules and EpCAM-negative PLC/PRF/5 cells. The capsules were thoroughly characterized via dark-field and fluorescence imaging, inductively coupled plasma mass spectroscopy, and Raman spectroscopy (Fig. 28). The nanocarriers were also investigated as potential structures for theranostic nanomedicine by utilizing their chemo-photothermal properties. After antibody-based cell targeting, the capsules were heated and triggered to release encapsulated doxorubicin via laser-light irridation.154 Further nanoparticle vesicles from polymer-grafted gold colloids from Song et al. as well as from Nie et al., will be discussed in the subsequent in vivo studies section.
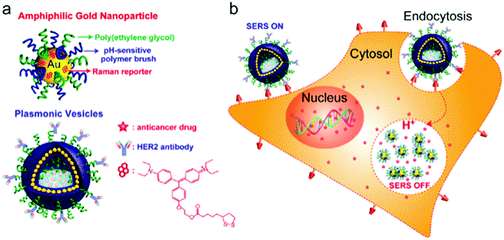 |
| Fig. 27 (a) Scheme NP Vesicle formed from PEG/PMMAVP@AuNPs conjugated with a Raman reporter, HER2 antibody and loaded with DOX; (b) scheme of cellular internalization and erosion process of the vesicles (Copied with permission from ref. 152. Copyright (2012) American Chemical Society.). | |
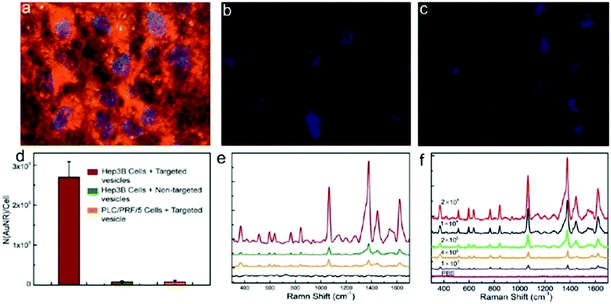 |
| Fig. 28 Hep3B cells cultured with (a) targeted, (b) native and (c) live PLC/PRF/5 cells all incubated with Au@PEG/PLA based colloidal capsules. (d) Vesicles taken up the cells; (e) SERS signals and (f) SERS spectra of Hep3B cells cultured with different vesicles. (Copied with permission from ref. 154. Copyright (2013) American Chemical Society.) | |
Nanoparticle membrane loaded liposomes and polymersomes have also been investigated intensely in in vitro studies. Wei et al. for example used biocompatible PEG–PLA BCPs in the combination with hydrophobic Fe3O4 particles for the formation of nanoparticle loaded polymersomes. By conjugating the polymersomes with a secondary antibody, the structures gained immunolabeling properties, which were probed by the authors on a prostate specific antigen, potentially allowing for their use for the detection of different cancer biomarkers. The polymersome immunosensor's effectiveness was investigated for the detection of different prostate-specific antigen (PSA) concentrations (Fig. 29) and compared well an established enzyme-linked immunosorbent assay (ELISA) method.195 Based on ultra-small superparamagnetic iron oxide nanoparticles (USPION) bilayer loaded poly(trimethylene carbonate)-b-poly(L-glutamic acid) (PTMC-b-PGA) polymersomes, Lecommandoux et al. created doxorubicin loaded vesicles, which may also serve as magnetic resonance imaging (MRI) contrast agents. The nanoparticle–polymersome hybrid's morphological properties were visualized via (cryo-)TEM (Fig. 30 – top-left), AFM and small angle neutron scattering (SANS), while the magnetic properties were analyzed via magnetophoresis and with a magnetometer. Furthermore, MRI properties were investigated by measuring the T1 and T2 relaxation times of the Fe3O4 particles in the bilayer. After loading the polymersomes with doxorubicin, the drug could be released in vitro from the vesicles by heating the UPSIONs via an oscillating magnetic field.196 In a follow-up study, Lecommandoux et al. investigated the ability of doxorubicin release after the internalization of the magneto-polymersomes in HeLa cells.197 Besides this, liposomes decorated with a magnetic-hydroxyapatite layer were investigated by Liu et al. for ultrasound-triggered drug release.349 Similarly, and as briefly mentioned in Section 2.4.1, Amstad et al.191 and Qui et al.193 utilized iron oxide particles loaded into the bilayer of liposomes, and were able to control the membrane permeability also via heating of the iron oxide particles within the bilayer. Both groups used calcein as a model drug to study the release from the magneto-liposomes. Design strategies and functional properties of smart vesicles, including nanoparticle equipped polymersomes, were recently reviewed by Lecommandoux and co-workers.350
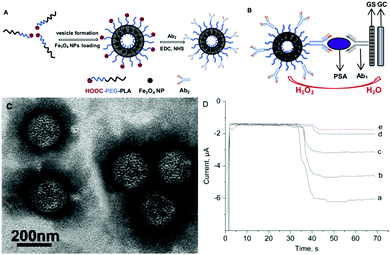 |
| Fig. 29 (A) Scheme of loading process of magneto-polymersomes and (B) immunosensor capabilities; (C) TEM micrograph; (D) immunosensor effectiveness for the detection of PSA (Reprinted from ref. 195, Copyright (2010), with permission from Elsevier.). | |
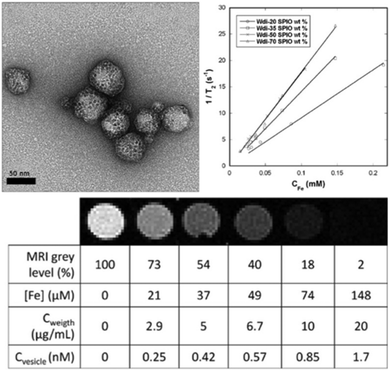 |
| Fig. 30 (top-left) TEM micrograph of PTMC-b-PGA based polymersomes loaded with iron oxide particles; (top-right) transverse relaxation rates vs. iron conc. of magneto-polymersomes loaded with varying iron oxide weight percentages; (bottom) T2-weighted MRI images and values for concentrations of iron ions in different samples. (Adapted with permission from ref. 196. Copyright (2013) American Chemical Society.) | |
Further in vitro studies for the use of capsules based on protein building blocks, were performed by Mertz and co-workers. Here, as briefly mentioned above, HSA-based capsules were formed on mesoporous silica templates (see Fig. 24 in Section 4.2) and modified with macrocyclic gadolinium (DOTA–Gd) complexes and were investigated for their potential use as MRI contrast agent. T1-weighted MR imaging showed MRI contrast enhancements for HSA capsules modified with and without siRNA (Fig. 31 – left A and B), which compared well to regulatory approved Gd-based MRI contrast agents. Furthermore, the authors reported investigations on the gene silencing abilities of the HSA capsules, which were probed on A549luc lung carcinoma cell lines via quantification of luciferase activity. Depending on the initial siRNA amount throughout the HSA capsules, the gene reporter expression within the A549luc cells could be inhibited up to 80% without Gd-modification and up to 50% with Gd-modification.129
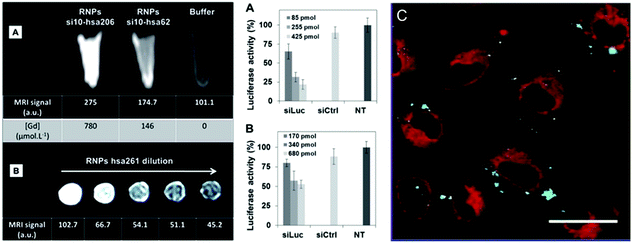 |
| Fig. 31 Left (A) T1-weighted MRI images of HSA capsules modified with Gd and siRNA (B) T1-weighted MRI images of HSA capsules modified solely with Gd. Biological in vitro tests. Right luciferase activity indicating the gene silencing ability of the siRNA/HSA capsules (A) without and (B) with Gd. (C) Images of A549 cells incubated with Gd and siRNA HSA capsules. (From ref. 129. Reproduced with permission from The Royal Society of Chemistry.) | |
5.3
In vivo studies of nano-scaled colloidal capsules
Recently, initial preclinical studies to assess a potential future bench-to-bedside translation of colloidal capsules were performed in in vivo experiments in various rodent models. As mentioned above, Nie et al.27,150,326 and Song et al.26,151 have both recently published reviews about colloidal capsules from polymer-grafted Au particles, including summaries about recent in vivo studies. We recommend these reviews for secondary references and will only marginally discuss the therein reviewed reports. We will start the discussion on in vivo studies with recent reports from Song and co-workers, who utilized the EPR effect for passive tumor targeting. Here, for the formation of colloidal capsules, the authors used 8 × 2 nm-sized gold nanorods, which, due to their small size, potentially possess renal clearance capabilities.331 The resulting capsules featured sizes between 60 and 100 nm, which is theoretically small enough to prevent an accumulation in the reticuloendothelial system, which usually occurs for such structures featuring sizes above 100 nm. The size of these colloidal capsules stand in good accordance to a recent liposome-based study for the ideal size of 50 nm for vesicles with the highest tumor tissue retention.351 The nanorods were functionalized with PLGA and PEG grafts to form emulsion-based biocompatible colloidal capsules. As PLGA is prone to degrade in an aqueous environment through de-esterification, the capsules were able to nearly completely dissociate to their individual units after 11 days. Resulting from the efficient plasmonic coupling of the nanorods, the vesicles were also investigated for their potential use as photothermal therapy (PTT) vehicles through laser-induced heating, and could further be monitored in vivo via photoacoustic (PA) imaging as well as with positron emission tomography (PET) after 64Cu-labeling of the vesicles (Fig. 32). In vitro experiments were performed on U87MG human glioma cell lines and all in vivo experiments were conducted using glioblastomar carrying U87MG mice. After intraveneous injection of the vesicles, control experiments, using photoacoutic imaging, vesicles showed a higher accumulation in the tumor region in comparison to the individual vesicle-forming gold nanorods. PET imaging revealed a vesicle uptake in the tumors of approx. 9.5% ID g−1 after 24 h and a blood half-life of approx. 18 h of vesicles, and the authors conclusively report a major clearance of the vesicles after 10 days of their administration. However, it needs to be noted, that after 10 days, vesicle ID g−1 values still reached at least 2.5% in most organs, and a critical value of 5% in the liver. Furthermore, the authors studied photothermal therapy efficiency on xenografted tumor-bearing mice, and were able to reach heat-induced tissue damage within the vesicle containing tumors. However, all tumor-bearing mice reportedly died after 40 to 50 days, due to reoccurrence of the tumor.52
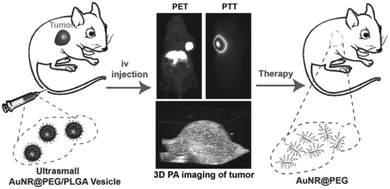 |
| Fig. 32 Scheme of vesicle administration to mice and their subsequent erosion to individual building blocks after PET and PA imaging was applied (Reproduced with permission from ref. 52. Copyright 2015, Wiley-VCH Verlag GmbH & Co. KGaA.). | |
In a similar recent study by Song et al., Au-nanorod@PEG/PLGA based vesicles were further equipped with doxorubicin-loaded and PEGylated reduced graphene oxide. Here, the interplay of the plasmonic Au nanorod capsules and the reduced graphene oxide led to enhanced photothermal performance of this hybrid vesicle-like structure. The vesicles featured sizes of approx. 65 nm and in accordance to the study above, the vesicles impact could be monitored via PA imaging and via PET imaging after 64Cu-labeling. Fig. 33a shows 2D and 3D ultrasound photoacoustic images of U87MG tumor-bearing mice, and Fig. 33b the different PA intensities of the vesicles with and without graphene oxide. Vesicles labeled with 64Cu allowed for PET imaging, reportedly showing a blood half-life of approx. 19.6 h of the vesicles (Fig. 33c) and a corresponding tumor uptake which increased from 0.79% after 1 h to approx. 9.7% ID g−1 after 24 h (Fig. 33d and e). Furthermore, using 808 nm, 0.25 W cm−2 laser light irradiation, the authors reported to be able to utilize the photothermal abilities of the vesicles to increase the tumor temperature, causing the vesicles to disrupt and leading to a controlled release of the encapsulated doxorubicin. These chemo-photothermal therapy properties of vesicles were assessed using thermographic imaging and monitoring the temperature change in the tumor region (Fig. 34a and b). The authors observed no difference in therapeutic efficiency for vesicles with and without doxorubicin for the first 5 days. Over a longer time span, the tumor growth in mice treated with doxorubicin loaded vesicles was delayed in comparison to DOX-free vesicles. This led to an increased life-span of the Au-vesicle-dox treated mice (>40 d) in comparison to free DOX and native Au-vesicles (20–26 d) (Fig. 34d). Additionally, tissue sections of differently treated tumors showed most severe cancer necrosis and least cancer cells for the DOX-loaded Au-vesicles in comparison to the other samples (Fig. 34e).155 In conclusion, these two studies by Song et al. represent seminal approaches for further in vivo studies of similar kind, where good practice is applied and guidelines for the synthesis for nanomedicine structures are followed. Their use of gold nanorods as the shell-forming building blocks, which potentially possess favourable clearance properties in comparison to larger particles or spherical gold colloids, builds a fundament for future studies in this field. However, besides the beneficial short-term therapeutic effects the potential negative long-term consequences of the gold nanoparticles should be investigated in more detail in future studies.352,353
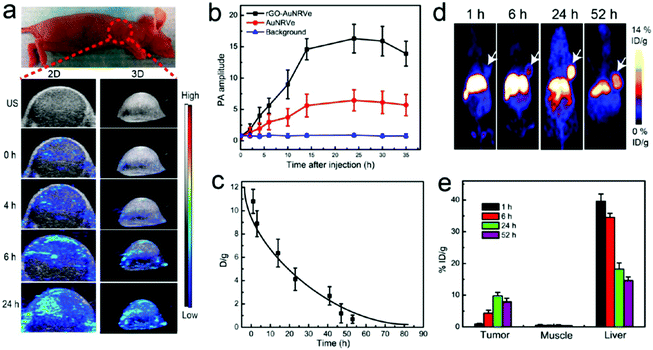 |
| Fig. 33 (a) In vivo images acquired via ultrasound and photoacoustic measurements; (b) PA values of tumor region; (c) biodistribution of 64Cu labeled colloidal capsules; (d) coronal PET images after administration of vesicles; (e) biodistribution of vesicles in different organs (Adapted with permission from ref. 155. Copyright (2015) American Chemical Society.). | |
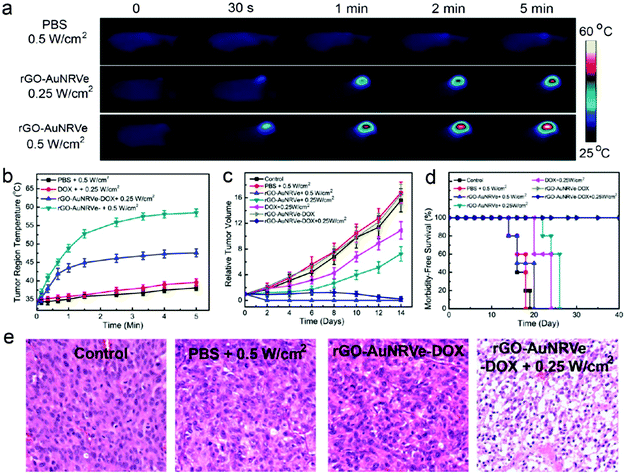 |
| Fig. 34 (a) Thermal images of the tumor region injected with Au NP vesicles and (b) resulting temperature change after irradiation with a 808 nm laser. (c) Relative tumor volume of the tumor-bearing mice and (d) comparison of survival curves of mice receiving varying treatments. (e) Comparison of tumor tissue sections, showing a more severe cancer necrosis and fewer cancer cells for treatment with DOX-loaded Au NP vesicles. (Adapted with permission from ref. 155. Copyright (2015) American Chemical Society.) | |
Nie et al. also performed in vivo experiments utilizing vesicles formed from BCP-tethered gold colloids. In initial in vivo experiments Nie et al. used a xenograft model of mice bearing 4T1 tumors. Vesicles formed from PEG–PS–BCP coated 40 nm sized Au NP were intratumorally injected and heated via 808 nm laser irradiation. The authors reported good therapeutic efficacy, as the tumors were ablated and showed no reoccurrence.144 Following this report, Nie and co-workers synthesized Au NP based vesicles, encapsulating a photosensitizer chlorine e6 (Ce6) within their hollow interior (Fig. 35 – left). The vesicles were assembled from PEO45-b-PS245 mediated 26 nm sized Au core-NP and featured average diameters of approx. 280 nm. The vesicles possessed intrinsic fluorescent, thermal, and photoacoustic properties which could reportedly be utilized for photothermal treatment by heating the vesicular Au NP membrane and photodynamic therapy (PDT)354 by using the singlet oxygen (1O2) species produced by the Ce6 photosensitizer upon light irradiation, to treat malignant cells. In vitro in a MDA-MB-435 human breast cancer cell line, the authors were able to dissociate the Au NP vesicles through photothermal activation, causing the Ce6 to be released. This was quantified by measuring the fluorescent signal of singlet oxygen sensor green (SOSG), which is reportedly altered by reacting with the singlet oxygen created by the Ce6. The vesicles were taken up by the cells via an endocytic-pathway and were mainly found within lysosomes of the cells. Furthermore, the cell experiments showed decreased cell viability for cells incubated with Ce6-functionalized Au NP vesicles and treated via PDT/PTT in comparison to free Ce6 and unloaded Au NP vesicles. For in vivo experiments, the authors used mice with MDA-MB-435 breast cancer xenografts, and Ce6 loaded vesicles were again intratumorally administered. The authors then used in vivo fluorescence, thermal, and photoacoustic imaging to track the tumor (Fig. 35a, b and d). The PTT effectiveness was investigated by increasing the temperature of the tumor to above 42 °C via laser irradiation (Fig. 35c), whilst tissue in close proximity to the tumor was barely affected. The Ce6 mediated Au NP vesicles exhibited the highest therapeutic efficacy in comparison to control experiments, indicating promising theranostic abilities of these structures.159
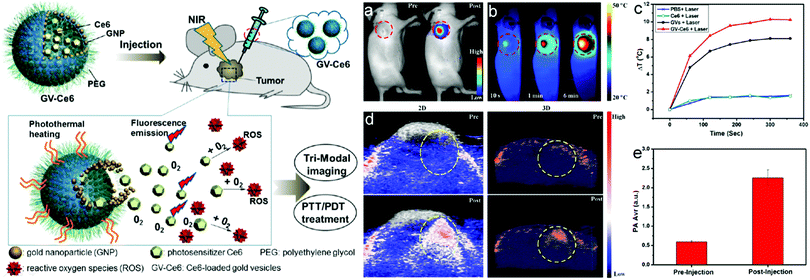 |
| Fig. 35 Left: Scheme of vesicle injection and Ce6 release; right: (a) NIR fluorescence and (b) thermal images of a tumor-bearing mouse, (c) tumor heat response to laser irradiation; (d) photoacoustic images and; (e) intensities of tumor tissues (Adapted with permission from ref. 159. Copyright (2015) American Chemical Society.). | |
In another report from Nie and co-workers, the PS block was substituted with polycaprolactone (PCL), to increase the biocompatibility of the used BCPs and the resulting vesicles. PCL reportedly features a melting of approx. 60 °C enabling its degradation through thermal heating of the Au NP vesicles. The vesicles featured a strong plasmonic coupling effect, shifting the LSPR peaks to NIR wavelengths. This shift was again used for in vivo PTT experiments in combination with rapid clearance of the individual Au NP after vesicle dissociation. The in vivo experiments were again performed by intratumorally injecting the vesicles in mice bearing MDA-MB-435 tumor xenografts. Experimental methodology was applied in accordance to the above discussed paper by Song and co-workers. PA and PT imaging was applied and the PTT effectiveness was assessed for Au NP vesicles. Tumor growth could be efficiently slowed and no reoccurrence was observed in mice treated with vesicles, in comparison to a control group. The overall therapeutic efficacy was the highest for the PCL based vesicles, in comparison to free gold nanorods or PS based vesicles. The life span of the mice treated with PCL vesicles was greater than 30 days, whilst the average life span of the other groups was between 14 and 20 days. Due to their size, the vesicles were mainly taken up by the RES system, i.e. mainly in the liver and spleen, which were cleared 8 days post injection.50 Nie et al. were also able to further tailor the build-up of nanoparticles within a Au NP-PS-b-PEO vesicle membrane, leading to string-like arrangements. These NP ‘chain vesicles’ posses an eightfold increased PA imaging efficiency over non-chained vesicles and also allow for a NIR induced release of an encapsulated model drug (Rhodamine B). Nude mice were subcutaneously injected with the vesicles and irradiated with NIR laser light. The increased efficiency of the chain vesicles was then visualized via PA imaging.162 Further in vivo studies were performed by Nie et al. with magnetic and plasmonic janus vesicles comprising a densely loaded membrane of gold NP and Fe3O4 NP. Due to plasmonic coupling and ordering of the particles, the structures featured shifted NIR peaks towards higher wavelengths and also exhibited an enhanced transverse relaxation effect, allowing the structures to be utilized as contrast agents in PA and T2-weighted MR imaging. The authors also demonstrated the controlled destruction of the structures triggered by NIR light in combination with a controlled release of a model drug through a magnetic field. For the in vivo studies U87MG tumor-bearing athymic nude mice were intravenously injected with hemispherical janus. Targeting towards the tumors was performed with an external magnet and identified via MR and PA imaging.202
The possibility to combine various types of materials with exploitable properties to create synergies for combined drug targeting and bioimaging is one of the central characteristic colloidal capsules possess and which sets them apart from most other vesicles and nanocapsules. The studies provided by Nie et al. describe pioneering works for the use of multifunctional colloidal capsules for bionanotechnology, which aim to utilize the full potentials of the colloidal capsule building block system. In future studies, it would be particularly interesting to further miniaturize the capsules towards diameters below 100 nm for the utilization of size-depended clearance pathways330,355 after capsule erosion.
In a report from another group, Deng et al. applied a grafting-TO approach, similar to previously reported works from Song et al., to functionalize 14 nm Au NP with hydrophilic poly-2-(2-methoxyethoxy) ethyl methacrylate (PMEO2MA) and hydrophobic PCL polymer grafts. The authors also observed a shift of the LSPR to (near) infrared wavelengths. PT imaging was applied for in vivo experiments of female BALB/c nude mice which, following subcutaneous MCF-7 cell injection, beard MCF-7 tumors. Au NP based vesicles were intratumorally injected into the mice and could be visualized via PT imaging. Enhanced PTT characteristics were observed for larger Au NP vesicles, allowing for ablation of the tumors leading to complete tumor regression. The vesicles were also investigated by the authors as X-ray CT contrast agents, owing to their high absorption coefficient. The contrast of the tumor tissue could be greatly enhanced post injection of the Au NP vesicles (Fig. 36A and B) and subsequently allowed for three-dimensional visualization of the tumor (Fig. 36C).300
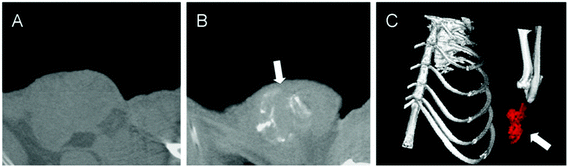 |
| Fig. 36 (A) X-ray computed tomography images of tumor-bearing mice prior, (B) after administration of gold colloid assemblies with diameters of approx. 500 nm leading to an increased Hounsfield Unit value, and (C) 3D CT image of tumor tissue (marked in red) (from ref. 300. Copied with permission from Ivyspring International Publisher (2014)). | |
Rotello and co-workers used dendrimers to stabilize the shell of paclitaxel-loaded colloidal nanocapsules, which were formed from 2 nm-sized Au core particles. The authors used poly(amido amine) (PAMAM) dendrimers in combination with dithiocarbamate (DTC) chemistry for the cross-linking of the shell. B16F10 skin melanoma cell lines were incubated with the capsules to investigate their impact on cell viability. The cell lines were monitored via flow cytometry and ICP-MS analysis and reportedly showed good cellular uptake. Unloaded capsules showed low cytotoxicity and paclitaxel loaded capsules were more effective in decreasing cell viability in comparison to free paclitaxel. For in vivo studies, the authors used female C57BL6 mice with ages of 8–10 weeks, and weights between 20 and 25 g. B16F10 cells were injected into the mice, which were used for the studies after tumor sizes reached 100 mm3. Thereafter, 100 nm-sized paclitaxel-loaded capsules were administered intravenously and accumulated in various organs, as well as in the tumors, which the authors attributed to the passive targeting capabilities of the capsules, caused by the EPR effect. The authors observed notable decrease of tumor growth in mice treated with paclitaxel-loaded capsules, in comparison to paclitaxel-free capsules.356 For future studies, the elucidation of intra-organ biodistribution as well as clearance properties of the individual particles of the eroded capsules would be of significant interest.
In addition to these Au NP based in vivo studies, other groups have performed initial works in rodent models with iron oxide based colloidal capsules. These works focused on the sparely and closely packed integration of iron oxide particles in the shell of polymeric nano-357 and microbubbles.358,359 These gas-filled structures which feature a polymer shell, are being extensively explored as contrast agents in ultrasonography, magnetic resonance imaging and in single photon emission computer tomography (SPECT) imaging. The structures usually feature sizes on the border of the micro–nano-scale, with diameters above or slightly below 1 μm. Brismar et al. reported initial work in this field by investigating magnetic nanoparticle coated microbubbles for MRI and computer tomography. The structures were prepared by loading 8–10 nm sized aminated SPIONs into the shell or onto the surface of poly(vinyl alcohol) and chitosan based microbubbles. The resulting structures featured sizes of approx. 3.8 μm and exhibited superparamagnetic properties, which was evidenced using vibrating sample magnetometer measurements. The authors performed preliminary proof-of-concept experiments for the use of the structures as visualizing agents in magnetic resonance imaging by administrating the structures to rats.360 In a follow-up report, microbubbles with enhanced multimodality imaging properties were investigated in male Sprague Dawley rats.361 Recently, Duan et al. templated γ-Fe2O3 NP against PVA/PLLA microbubbles and investigated the structures as potential agents in magnetic resonance/ultrasound (MR/US) imaging. The authors were able to tailor the T2 MR contrast by increasing the amount of particles templated on the surface. In vivo studies were performed with tumor bearing female BALB/C nude mice which were beforehand injected with COLO-205 cells. MRI imaging was then applied by the authors after intratumoral injection of the SPION templated microbubbles, which resulted in a decrease of the T2 signal, overall improving the MR imaging results in comparison to the state prior to injection.362 Similar structures with decreased sizes of approx. 180–200 nm, i.e. SPION mediated nanobubbles, were reported by Huang and co-workers. The nanobubbles were prepared from perfluoropentane and PAA–F127 thermosensitive polymers and approx. 5 nm-sized SPIONs.28
5.4 Conclusions for colloidal capsules for biological applications
The above described initial experiments represent promising first approaches for the use of nano-scaled colloidal capsules in nanomedicine. However, besides the positive short-term effects these novel nano-pharmaceutical agents show, the mid- and long-term biological fate of the particles, i.e. biodistribution and clearance, effects on cell cycling and cell signaling processes, etc., remain greatly unknown. A complete understanding of the individual shell-forming nanoparticles needs to be fully established in future studies before their use in biological applications should be considered.
Generally, the huge and still growing field of nanomedicine363 with its claim for novel ways to cure diseases has recently raised critical voices and has led to significant debate,364–369 questioning whether this field will anytime soon return the credit it received in advance. Numerous aspects such as EPA law, FDA law, ethics, environmental health, safety and toxicity370 along with standardization need to be taken into account for the above nanoparticle types to be considered for in-human use over organic components such as amphiphiles, peptides, proteins or FDA-approved biocompatible polymers (i.e. PLGA or PCL). A multitude of factors need to be considered for nanocarrier design,371 including particle/capsule size and morphology,351,372–374 surface charge,375 particle/capsule hardness, potential capsule deformability, complete renal clearance capabilities,319 active/passive targeting (EPR effect),333 precise design for biological barrier interaction,366 pathways for cellular uptake,376 and surface functionalization with e.g. PEG377 or PEEP378 for tailored blood half-life (stealthing). Furthermore, a pivotal role is attributed to the formation of a protein corona379–381 on nanoparticles in biological media which can lead to loss of the receptor targeting ability of protein, antibody or other biomolecule functionalizations on nanoparticles/nanocapsules and which can facilitate rapid macrophage uptake and clearance of the biofunctional colloids.380 Here, ultrasmall nanoparticles with a zwitterionic coating382 or certain moieties with tunable hydrophobicity383 are of increasing interest as they have shown to inhibit biomolecular corona formation effectively and should be considered for new colloidal capsule studies that aim at in vivo applications. However, various pharmacokinetic and biodistribution properties, such as intracellular trafficking,384 organelle targeting, or suborgan biodistribution385 of (ultrasmall) nanoparticles with distinct surface functionalizations, are still greatly unexplored.325 These and other short and long-term352 nanotoxicological386 factors demand for highly specific designs of studies by all researchers in this field.327,387 Also, since humans, by nature, are not capable of metabolizing most types of synthetic nanoparticles, the full clearance, e.g. by the renal route,319,330,331 of non-biodegradable particles are an absolute prerequisite for a potential in-human use, especially in consideration of the discontinuation of the clinical use of some previous nanoparticle agents323 (i.e. Ferucarbotran, Ferumoxstran, Ferumoxides). With this said, it will be exciting to see if a general rationale as well as feasible synthetic routes will be found to add colloidal capsules formed from the above described material classes as an alternative to already approved363 nanomedicial agents formed from natural amphiphile molecules or protein building blocks, like, Doxil® (doxorubicin HCl liposome injection), DaunoXome® (daunorubicin citrate liposome injection) or Abraxane® (albumin-bound paclitaxel). Additionally, the reports of more and more pre-clinical systems tailored for drug-delivery applications of nanocarriers emphasize the need for other nanomedical agents than just tumors388 in the future, e.g. for cancer immunotherapy389 or the treatment of endothelial disorders390 and pulmonary diseases.391
6. Conclusions and outlook
Great advancements have been achieved in the development of colloidal capsules in the last two decades, including the establishment of a reproducible synthesis route for the fabrication of monodisperse micron-sized capsules, the miniaturization of the capsules combined with the integration of distinct functionalities, and the synthesis of biocompatible nano-scaled capsules with tailored erosion properties as novel nano-pharmaceutical agents. We have reviewed the initial reports and recent literature on colloidal capsules. By reviewing the above described synthesis routes, i.e. (1) interfacial colloidal particle self-assembly on emulsion droplets; (2) colloidal templating against hard or soft particles; (3) solvent change induced assembly of polymer-grafted amphiphilic colloids; and (4) dense membrane nanoparticle-loading of liposomes/polymersomes, we aim at unifying the discussion on colloidal capsules and with that, facilitate communication and further progress in this nascent research field.
However, some critical aspects also have to be noted. The characterization of the capsules is often incomplete as repeatedly just one or a few of the following capsule properties are provided in detail, i.e.; colloidal stability in various media, erosion kinetics of the capsules, size and polydispersity, topological (i.e. membrane permeability), shell (i.e. degree of order of particles), and internal structural build-up, mechanical stability in liquid media and dried state, as well as chemical composition (i.e. functional groups or ligands). With biomedical applications in mind, it is especially critical to study these material properties in biological environments, as the presence of components from the media/blood, like blood cells, proteins, and salts can drastically alter colloidal stability, surface properties and degradability of the colloidal capsules. As a result, with many reported colloidal capsule systems it is not clear if the production of these capsules can be scaled to allow further development of the capsule system in a biological context.
Furthermore, while the mechanisms of the adsorption and desorption of colloidal particles at planar interfaces are well studied the understanding of the behaviour of nanoparticles at the interface of nano-emulsions is far from complete. A recent study on the organization of colloids at the interface of micron-sized emulsion droplets might be a starting point for future studies.81 van der Waals, electrostatic double-layer, solvation, and hydrodynamic drag forces as well as Brownian motion, are the driving forces within self-assembly processes,266,392 though little efforts have been undertaken in most studies to characterize these forces within a respective colloidal capsule synthesis route. A deeper understanding of the pair-wise and multi-component interactions (i.e. colloid–colloid, colloid–solvent, colloid–salts and, if present, colloid–template) could provide a better general prediction of the structural outcome of capsules prior to their synthesis. This calls for the development of novel measurement techniques that can monitor the mentioned properties in situ and at the nanoscale as well as fundamental research on thin films at fluid interfaces and the non-trivial interactions during the colloidal assembly of multicomponent nanoparticles. For instance, the recent development of liquid cells for in situ liquid TEM may allow the visualization and improved understanding of some colloidal capsule formation and other nanoparticle self-assembly processes in the future.393,394 Naturally, these experimental techniques should be supplemented with computer simulations at various scales (atomistic modeling of interfaces, coarse grain models for particle interactions, etc.) which can provide useful theoretical predictions and support the rational design of colloidal capsules.
Hence, plenty of hard work remains for advancing the broad field of colloidal capsule research which is currently one of the most exciting fields in colloid chemistry. We are looking forward to witness the development of many more sophisticated structures that surpass simple colloidal particles, other nano- and micro-capsules, and vesicles by featuring a range of tailor-made properties for specific advanced applications.
Acknowledgements
We are grateful for funding by the German Research Foundation (DFG) by the grants MA4795/5-1 and /5-2.
References
- R. Gref, Y. Minamitake, M. T. Peracchia, V. Trubetskoy, V. Torchilin and R. Langer, Science, 1994, 263, 1600–1603 CAS.
- S. Gouin, Trends Food Sci. Technol., 2004, 15, 330–347 CrossRef CAS.
- C. S. Peyratout and L. Dähne, Angew. Chem., Int. Ed., 2004, 43, 3762–3783 CrossRef CAS PubMed.
- R. Akiyama and S. Kobayashi, Chem. Rev., 2009, 109, 594–642 CrossRef CAS PubMed.
- G. Nelson, Int. J. Pharm., 2002, 242, 55–62 CrossRef CAS PubMed.
- G. Gregoriadis, FEBS Lett., 1973, 36, 292–296 CrossRef CAS PubMed.
- V. P. Torchilin, Nat. Rev. Drug Discovery, 2005, 4, 145–160 CrossRef CAS PubMed.
- W. T. Al-Jamal and K. Kostarelos, Acc. Chem. Res., 2011, 44, 1094–1104 CrossRef CAS PubMed.
- L. Zhang and A. Eisenberg, Science, 1995, 268, 1728–1731 CAS.
- B. M. Discher, Y.-Y. Won, D. S. Ege, J. C.-M. Lee, F. S. Bates, D. E. Discher and D. A. Hammer, Science, 1999, 284, 1143–1146 CrossRef CAS PubMed.
- D. E. Discher and A. Eisenberg, Science, 2002, 297, 967–973 CrossRef CAS PubMed.
- A. D. Dinsmore, M. F. Hsu, M. G. Nikolaides, M. Marquez, A. R. Bausch and D. A. Weitz, Science, 2002, 298, 1006–1009 CrossRef CAS PubMed.
- J.-W. Kim, A. Fernández-Nieves, N. Dan, A. S. Utada, M. Marquez and D. A. Weitz, Nano Lett., 2007, 7, 2876–2880 CrossRef CAS PubMed.
- M. Liu, Q. Tian, Y. Li, B. You, A. Xu and Z. Deng, Langmuir, 2015, 31, 4589–4592 CrossRef CAS PubMed.
- J. He, Y. Liu, T. Babu, Z. Wei and Z. Nie, J. Am. Chem. Soc., 2012, 134, 11342–11345 CrossRef CAS PubMed.
- X.-C. Yang, B. Samanta, S. S. Agasti, Y. Jeong, Z.-J. Zhu, S. Rana, O. R. Miranda and V. M. Rotello, Angew. Chem., Int. Ed., 2011, 50, 477–481 CrossRef CAS PubMed.
- Z. Cao, A. Schrade, K. Landfester and U. Ziener, J. Polym. Sci., Part A: Polym. Chem., 2011, 49, 2382–2394 CrossRef CAS.
- D. Patra, A. Sanyal and V. M. Rotello, Chem. – Asian J., 2010, 5, 2442–2453 CrossRef CAS PubMed.
- F. Caruso, R. A. Caruso and H. Möhwald, Science, 1998, 282, 1111–1114 CrossRef CAS PubMed.
- Z. Nie, D. Fava, E. Kumacheva, S. Zou, G. C. Walker and M. Rubinstein, Nat. Mater., 2007, 6, 609–614 CrossRef CAS PubMed.
- R. Tang, C. S. Kim, D. J. Solfiell, S. Rana, R. Mout, E. M. Velázquez-Delgado, A. Chompoosor, Y. Jeong, B. Yan, Z.-J. Zhu, C. Kim, J. A. Hardy and V. M. Rotello, ACS Nano, 2013, 7, 6667–6673 CrossRef CAS PubMed.
- C. Wu, S. Bai, M. B. Ansorge-Schumacher and D. Wang, Adv. Mater., 2011, 23, 5694–5699 CrossRef CAS PubMed.
- A.-M. Cao, J.-S. Hu, H.-P. Liang and L.-J. Wan, Angew. Chem., Int. Ed., 2005, 44, 4391–4395 CrossRef CAS PubMed.
- A. Rogach, A. Susha, F. Caruso, G. Sukhorukov, A. Kornowski, S. Kershaw, H. Möhwald, A. Eychmüller and H. Weller, Adv. Mater., 2000, 12, 333–337 CrossRef CAS.
- N. Vogel, S. Utech, G. T. England, T. Shirman, K. R. Phillips, N. Koay, I. B. Burgess, M. Kolle, D. A. Weitz and J. Aizenberg, Proc. Natl. Acad. Sci. U. S. A., 2015, 112, 10845–10850 CrossRef CAS PubMed.
- J. Song, P. Huang, H. Duan and X. Chen, Acc. Chem. Res., 2015, 48, 2506–2515 CrossRef CAS PubMed.
- Y. Liu, Y. Liu, J.-J. Yin and Z. Nie, Macromol. Rapid Commun., 2015, 36, 711–725 CrossRef CAS PubMed.
- H.-Y. Huang, S.-H. Hu, S.-Y. Hung, C.-S. Chiang, H.-L. Liu, T.-L. Chiu, H.-Y. Lai, Y.-Y. Chen and S.-Y. Chen, J. Controlled Release, 2013, 172, 118–127 CrossRef CAS PubMed.
-
E. Herz, A. Burns, S. Lee, P. Sengupta, D. Bonner, H. Ow, C. Liddell, B. Baird and U. Wiesner, Fluorescent core-shell silica nanoparticles: an alternative radiative materials platform, 2006, vol. 6096, p. 609605 Search PubMed.
- T. Bollhorst, S. Shahabi, K. Wörz, C. Petters, R. Dringen, M. Maas and K. Rezwan, Angew. Chem., Int. Ed., 2015, 54, 118–123 CrossRef CAS PubMed.
- O. Chen, L. Riedemann, F. Etoc, H. Herrmann, M. Coppey, M. Barch, C. T. Farrar, J. Zhao, O. T. Bruns, H. Wei, P. Guo, J. Cui, R. Jensen, Y. Chen, D. K. Harris, J. M. Cordero, Z. Wang, A. Jasanoff, D. Fukumura, R. Reimer, M. Dahan, R. K. Jain and M. G. Bawendi, Nat. Commun., 2014, 5, 5093 CrossRef CAS PubMed.
- M. Pang, A. J. Cairns, Y. Liu, Y. Belmabkhout, H. C. Zeng and M. Eddaoudi, J. Am. Chem. Soc., 2013, 135, 10234–10237 CrossRef CAS PubMed.
- C. Huo, M. Li, X. Huang, H. Yang and S. Mann, Langmuir, 2014, 30, 15047–15052 CrossRef CAS PubMed.
- W. Cao, R. Huang, W. Qi, R. Su and Z. He, ACS Appl. Mater. Interfaces, 2015, 7, 465–473 CAS.
- M. Maas, T. Bollhorst, R. N. Zare and K. Rezwan, Part. Part. Syst. Charact., 2014, 31, 1067–1071 CrossRef CAS.
- V. N. Paunov and M. in het Panhuis, Nanotechnology, 2005, 16, 1522 CrossRef CAS.
- M. Karg, Y. Lu, E. Carbó-Argibay, I. Pastoriza-Santos, J. Pérez-Juste, L. M. Liz-Marzán and T. Hellweg, Langmuir, 2009, 25, 3163–3167 CrossRef CAS PubMed.
- P. F. Noble, O. J. Cayre, R. G. Alargova, O. D. Velev and V. N. Paunov, J. Am. Chem. Soc., 2004, 126, 8092–8093 CrossRef CAS PubMed.
- K. L. Thompson, P. Chambon, R. Verber and S. P. Armes, J. Am. Chem. Soc., 2012, 134, 12450–12453 CrossRef CAS PubMed.
- Z. Wang, M. C. M. van Oers, F. P. J. T. Rutjes and J. C. M. van Hest, Angew. Chem., Int. Ed., 2012, 51, 10746–10750 CrossRef CAS PubMed.
- R. Chandrawati, L. Hosta-Rigau, D. Vanderstraaten, S. A. Lokuliyana, B. Städler, F. Albericio and F. Caruso, ACS Nano, 2010, 4, 1351–1361 CrossRef CAS PubMed.
- J. T. Russell, Y. Lin, A. Böker, L. Su, P. Carl, H. Zettl, J. He, K. Sill, R. Tangirala, T. Emrick, K. Littrell, P. Thiyagarajan, D. Cookson, A. Fery, Q. Wang and T. P. Russell, Angew. Chem., Int. Ed., 2005, 44, 2420–2426 CrossRef CAS PubMed.
- X. Huang, M. Li, D. C. Green, D. S. Williams, A. J. Patil and S. Mann, Nat. Commun., 2013, 4, 2239 Search PubMed.
- M. Li, R. L. Harbron, J. V. M. Weaver, B. P. Binks and S. Mann, Nat. Chem., 2013, 5, 529–536 CrossRef CAS PubMed.
- M. Li, X. Huang, T.-Y. D. Tang and S. Mann, Curr. Opin. Chem. Biol., 2014, 22, 1–11 CrossRef CAS PubMed.
- S. Sun, M. Li, F. Dong, S. Wang, L. Tian and S. Mann, Small, 2016, 12, 1920–1927 CrossRef CAS PubMed.
- R. Tamate, T. Ueki and R. Yoshida, Angew. Chem., Int. Ed., 2016, 55, 5179–5183 CrossRef CAS PubMed.
- X. Huang, A. J. Patil, M. Li and S. Mann, J. Am. Chem. Soc., 2014, 136, 9225–9234 CrossRef CAS PubMed.
- J. Song, L. Cheng, A. Liu, J. Yin, M. Kuang and H. Duan, J. Am. Chem. Soc., 2011, 133, 10760–10763 CrossRef CAS PubMed.
- P. Huang, J. Lin, W. Li, P. Rong, Z. Wang, S. Wang, X. Wang, X. Sun, M. Aronova, G. Niu, R. D. Leapman, Z. Nie and X. Chen, Angew. Chem., Int. Ed., 2013, 52, 13958–13964 CrossRef CAS PubMed.
- Y. Jiang, R. Tang, B. Duncan, Z. Jiang, B. Yan, R. Mout and V. M. Rotello, Angew. Chem., Int. Ed., 2015, 54, 506–510 CAS.
- J. Song, X. Yang, O. Jacobson, P. Huang, X. Sun, L. Lin, X. Yan, G. Niu, Q. Ma and X. Chen, Adv. Mater., 2015, 27, 4910–4917 CrossRef CAS PubMed.
- Z. Lu and Y. Yin, Chem. Soc. Rev., 2012, 41, 6874–6887 RSC.
- J. K. Stolarczyk, A. Deak and D. F. Brougham, Adv. Mater., 2016, 28, 5400–5424 CrossRef CAS PubMed.
- O. D. Velev, K. Furusawa and K. Nagayama, Langmuir, 1996, 12, 2374–2384 CrossRef CAS.
- O. D. Velev, K. Furusawa and K. Nagayama, Langmuir, 1996, 12, 2385–2391 CrossRef CAS.
- O. D. Velev and K. Nagayama, Langmuir, 1997, 13, 1856–1859 CrossRef CAS.
- S. U. Pickering, J. Chem. Soc., Trans., 1907, 91, 2001–2021 RSC.
- W. Ramsden, Proc. R. Soc. London, 1903, 72, 156–164 CrossRef CAS.
- F. J. Rossier-Miranda, C. G. P. H. Schroën and R. M. Boom, Colloids Surf., A, 2009, 343, 43–49 CrossRef CAS.
- J. Wu and G.-H. Ma, Small, 2016, 12, 4633–4648 CrossRef CAS PubMed.
- K. L. Thompson, M. Williams and S. P. Armes, J. Colloid Interface Sci., 2015, 447, 217–228 CrossRef CAS PubMed.
- H. N. Yow and A. F. Routh, Langmuir, 2008, 25, 159–166 CrossRef PubMed.
- S. Laïb and A. F. Routh, J. Colloid Interface Sci., 2008, 317, 121–129 CrossRef PubMed.
- O. J. Cayre, P. F. Noble and V. N. Paunov, J. Mater. Chem., 2004, 14, 3351 RSC.
- C. Wang, H. Liu, Q. Gao, X. Liu and Z. Tong, ChemPhysChem, 2007, 8, 1157–1160 CrossRef CAS PubMed.
- H. Duan, D. Wang, N. S. Sobal, M. Giersig, D. G. Kurth and H. Möhwald, Nano Lett., 2005, 5, 949–952 CrossRef CAS PubMed.
- K. L. Thompson, S. P. Armes, J. R. Howse, S. Ebbens, I. Ahmad, J. H. Zaidi, D. W. York and J. A. Burdis, Macromolecules, 2010, 43, 10466–10474 CrossRef CAS.
- P. Arumugam, D. Patra, B. Samanta, S. S. Agasti, C. Subramani and V. M. Rotello, J. Am. Chem. Soc., 2008, 130, 10046–10047 CrossRef CAS PubMed.
- A. Walsh, K. L. Thompson, S. P. Armes and D. W. York, Langmuir, 2010, 26, 18039–18048 CrossRef CAS PubMed.
- A. Schmid, J. Tonnar and S. P. Armes, Adv. Mater., 2008, 20, 3331–3336 CrossRef CAS.
- Y. Chen, C. Wang, J. Chen, X. Liu and Z. Tong, J. Polym. Sci., Part A: Polym. Chem., 2009, 47, 1354–1367 CrossRef CAS.
- S. A. F. Bon, S. Cauvin and P. J. Colver, Soft Matter, 2007, 3, 194–199 RSC.
- O. J. Cayre and S. Biggs, J. Mater. Chem., 2009, 19, 2724 RSC.
-
Colloidal Particles at Liquid Interfaces, ed. B. P. Binks and T. S. Horozov, Cambridge University Press, Cambridge, New York, 1st edn, 2006 Search PubMed.
- P. Pieranski, Phys. Rev. Lett., 1980, 45, 569–572 CrossRef CAS.
- B. P. Binks and J. H. Clint, Langmuir, 2002, 18, 1270–1273 CrossRef CAS.
- Y. Lin, H. Skaff, T. Emrick, A. D. Dinsmore and T. P. Russell, Science, 2003, 299, 226–229 CrossRef CAS PubMed.
-
Particle-Stabilized Emulsions and Colloids, ed. T. Ngai and S. Bon, Royal Society of Chemistry, Cambridge, 2014 Search PubMed.
- G. Boniello, C. Blanc, D. Fedorenko, M. Medfai, N. B. Mbarek, M. In, M. Gross, A. Stocco and M. Nobili, Nat. Mater., 2015, 14, 908–911 CrossRef CAS PubMed.
- G. Meng, J. Paulose, D. R. Nelson and V. N. Manoharan, Science, 2014, 343, 634–637 CrossRef CAS PubMed.
- M. Destribats, S. Gineste, E. Laurichesse, H. Tanner, F. Leal-Calderon, V. Héroguez and V. Schmitt, Langmuir, 2014, 30, 9313–9326 CrossRef CAS PubMed.
- H. Wang, V. Singh and S. H. Behrens, J. Phys. Chem. Lett., 2012, 3, 2986–2990 CrossRef CAS PubMed.
- D. M. Kaz, R. McGorty, M. Mani, M. P. Brenner and V. N. Manoharan, Nat. Mater., 2012, 11, 138–142 CrossRef CAS PubMed.
- L. Isa, E. Amstad, K. Schwenke, E. D. Gado, P. Ilg, M. Kröger and E. Reimhult, Soft Matter, 2011, 7, 7663–7675 RSC.
- M. Maas, C. C. Ooi and G. G. Fuller, Langmuir, 2010, 26, 17867–17873 CrossRef CAS PubMed.
- M. E. Leunissen, A. van Blaaderen, A. D. Hollingsworth, M. T. Sullivan and P. M. Chaikin, Proc. Natl. Acad. Sci. U. S. A., 2007, 104, 2585–2590 CrossRef CAS PubMed.
- J. Zwanikken and R. van Roij, Phys. Rev. Lett., 2007, 99, 178301 CrossRef PubMed.
- M. Oettel, Phys. Rev. E: Stat., Nonlinear, Soft Matter Phys., 2007, 76, 041403 CrossRef CAS PubMed.
- P. A. Kralchevsky, I. B. Ivanov, K. P. Ananthapadmanabhan and A. Lips, Langmuir, 2005, 21, 50–63 CrossRef CAS PubMed.
- X. Quan, C. Peng, J. Dong and J. Zhou, Soft Matter, 2016, 12, 3352–3359 RSC.
- L. Isa, F. Lucas, R. Wepf and E. Reimhult, Nat. Commun., 2011, 2, 438 CrossRef PubMed.
- A. Stocco, G. Su, M. Nobili, M. In and D. Wang, Soft Matter, 2014, 10, 6999–7007 RSC.
- B. M. Weon, J. S. Lee, J. T. Kim, J. Pyo and J. H. Je, Curr. Opin. Colloid Interface Sci., 2012, 17, 388–395 CrossRef CAS.
- D. O. Grigoriev, J. Krägel, V. Dutschk, R. Miller and H. Möhwald, Phys. Chem. Chem. Phys., 2007, 9, 6447–6454 RSC.
- O. S. Deshmukh, D. van den Ende, M. C. Stuart, F. Mugele and M. H. G. Duits, Adv. Colloid Interface Sci., 2015, 222, 215–227 CrossRef CAS PubMed.
- A. J. Mendoza, E. Guzmán, F. Martínez-Pedrero, H. Ritacco, R. G. Rubio, F. Ortega, V. M. Starov and R. Miller, Adv. Colloid Interface Sci., 2014, 206, 303–319 CrossRef CAS PubMed.
- Z. Niu, J. He, T. P. Russell and Q. Wang, Angew. Chem., Int. Ed., 2010, 49, 10052–10066 CrossRef CAS PubMed.
- R. McGorty, J. Fung, D. Kaz and V. N. Manoharan, Mater. Today, 2010, 13, 34–42 CrossRef CAS.
- F. Leal-Calderon and V. Schmitt, Curr. Opin. Colloid Interface Sci., 2008, 13, 217–227 CrossRef CAS.
- C. Zeng, H. Bissig and A. D. Dinsmore, Solid State Commun., 2006, 139, 547–556 CrossRef CAS.
- A. Böker, J. He, T. Emrick and T. P. Russell, Soft Matter, 2007, 3, 1231 RSC.
- D. Wang, H. Duan and H. Möhwald, Soft Matter, 2005, 1, 412 RSC.
- E. Vignati, R. Piazza and T. P. Lockhart, Langmuir, 2003, 19, 6650–6656 CrossRef CAS.
- B. P. Binks, Curr. Opin. Colloid Interface Sci., 2002, 7, 21–41 CrossRef CAS.
- A. B. Subramaniam, M. Abkarian and H. A. Stone, Nat. Mater., 2005, 4, 553–556 CrossRef CAS PubMed.
- A. S. Utada, E. Lorenceau, D. R. Link, P. D. Kaplan, H. A. Stone and D. A. Weitz, Science, 2005, 308, 537–541 CrossRef CAS PubMed.
- D. Lee and D. A. Weitz, Adv. Mater., 2008, 20, 3498–3503 CrossRef CAS.
- D. Lee and D. A. Weitz, Small, 2009, 5, 1932–1935 CrossRef CAS PubMed.
- S. Zhou, J. Fan, S. S. Datta, M. Guo, X. Guo and D. A. Weitz, Adv. Funct. Mater., 2013, 23, 5925–5929 CrossRef CAS.
- R. K. Shah, J.-W. Kim and D. A. Weitz, Langmuir, 2009, 26, 1561–1565 CrossRef PubMed.
- J. S. Sander and A. R. Studart, Langmuir, 2011, 27, 3301–3307 CrossRef CAS PubMed.
- J. I. Park, Z. Nie, A. Kumachev, A. I. Abdelrahman, B. P. Binks, H. A. Stone and E. Kumacheva, Angew. Chem., 2009, 121, 5404–5408 CrossRef.
- E. Tumarkin, J. I. Park, Z. Nie and E. Kumacheva, Chem. Commun., 2011, 47, 12712 RSC.
- Z. Nie, J. I. Park, W. Li, S. A. F. Bon and E. Kumacheva, J. Am. Chem. Soc., 2008, 130, 16508–16509 CrossRef CAS PubMed.
- J. Zhang, R. J. Coulston, S. T. Jones, J. Geng, O. A. Scherman and C. Abell, Science, 2012, 335, 690–694 CrossRef CAS PubMed.
- J. He, L. Wang, Z. Wei, Y. Yang, C. Wang, X. Han and Z. Nie, ACS Appl. Mater. Interfaces, 2013, 5, 9746–9751 CAS.
- L. Wang, Y. Liu, J. He, M. J. Hourwitz, Y. Yang, J. T. Fourkas, X. Han and Z. Nie, Small, 2015, 11, 3762–3767 CrossRef CAS PubMed.
- F. Caruso, H. Lichtenfeld, M. Giersig and H. Möhwald, J. Am. Chem. Soc., 1998, 120, 8523–8524 CrossRef CAS.
- F. Caruso, Adv. Mater., 2001, 13, 11–22 CrossRef CAS.
- Y. Wang, A. S. Angelatos and F. Caruso, Chem. Mater., 2008, 20, 848–858 CrossRef CAS.
- X. W. (David) Lou, L. A. Archer and Z. Yang, Adv. Mater., 2008, 20, 3987–4019 CrossRef.
- F. Caruso, Chem. – Eur. J., 2000, 6, 413–419 CrossRef CAS.
- J. Guo, B. L. Tardy, A. J. Christofferson, Y. Dai, J. J. Richardson, W. Zhu, M. Hu, Y. Ju, J. Cui, R. R. Dagastine, I. Yarovsky and F. Caruso, Nat. Nanotechnol., 2016, 11, 1105–1111 CrossRef CAS PubMed.
- M. S. Fleming, T. K. Mandal and D. R. Walt, Chem. Mater., 2001, 13, 2210–2216 CrossRef CAS.
- S.-W. Kim, M. Kim, W. Y. Lee and T. Hyeon, J. Am. Chem. Soc., 2002, 124, 7642–7643 CrossRef CAS PubMed.
- D. Mertz, J. Cui, Y. Yan, G. Devlin, C. Chaubaroux, A. Dochter, R. Alles, P. Lavalle, J. C. Voegel, A. Blencowe, P. Auffinger and F. Caruso, ACS Nano, 2012, 6, 7584–7594 CrossRef CAS PubMed.
- D. Mertz, H. Wu, J. S. Wong, J. Cui, P. Tan, R. Alles and F. Caruso, J. Mater. Chem., 2012, 22, 21434 RSC.
- D. Mertz, C. Affolter-Zbaraszczuk, J. Barthès, J. Cui, F. Caruso, T. F. Baumert, J.-C. Voegel, J. Ogier and F. Meyer, Nanoscale, 2014, 6, 11676–11680 RSC.
- K. Gawlitza, S. T. Turner, F. Polzer, S. Wellert, M. Karg, P. Mulvaney and R. von Klitzing, Phys. Chem. Chem. Phys., 2013, 15, 15623 RSC.
- B. Du, Z. Cao, Z. Li, A. Mei, X. Zhang, J. Nie, J. Xu and Z. Fan, Langmuir, 2009, 25, 12367–12373 CrossRef CAS PubMed.
- T. Bollhorst, S. Jakob, J. Köser, M. Maas and K. Rezwan, J. Mater. Chem. B, 2017 10.1039/c6tb03069f.
- M. Destribats, V. Schmitt and R. Backov, Langmuir, 2010, 26, 1734–1742 CrossRef CAS PubMed.
- R. T. Rosenberg and N. R. Dan, J. Colloid Interface Sci., 2010, 349, 498–504 CrossRef CAS PubMed.
- R. T. Rosenberg and N. R. Dan, J. Colloid Interface Sci., 2011, 354, 478–482 CrossRef CAS PubMed.
- R. T. Rosenberg and N. Dan, J. Biomater. Nanobiotechnol., 2011, 02, 1–7 CrossRef CAS.
- T. Huang, H. Li, L. Huang, S. Li, K. Li and Y. Zhou, Langmuir, 2016, 32, 991–996 CrossRef CAS PubMed.
- L. Zhang, K. Yu and A. Eisenberg, Science, 1996, 272, 1777–1779 CAS.
- Y. Yu and A. Eisenberg, J. Am. Chem. Soc., 1997, 119, 8383–8384 CrossRef CAS.
- C. Tanford, Science, 1978, 200, 1012–1018 CAS.
- D. Chandler, Nature, 2005, 437, 640–647 CrossRef CAS PubMed.
- Y. Mai and A. Eisenberg, Chem. Soc. Rev., 2012, 41, 5969–5985 RSC.
- P. Tanner, P. Baumann, R. Enea, O. Onaca, C. Palivan and W. Meier, Acc. Chem. Res., 2011, 44, 1039–1049 CrossRef CAS PubMed.
- J. He, X. Huang, Y.-C. Li, Y. Liu, T. Babu, M. A. Aronova, S. Wang, Z. Lu, X. Chen and Z. Nie, J. Am. Chem. Soc., 2013, 135, 7974–7984 CrossRef CAS PubMed.
- J. He, P. Zhang, T. Babu, Y. Liu, J. Gong and Z. Nie, Chem. Commun., 2012, 49, 576–578 RSC.
- A. K. Boal, F. Ilhan, J. E. DeRouchey, T. Thurn-Albrecht, T. P. Russell and V. M. Rotello, Nature, 2000, 404, 746–748 CrossRef CAS PubMed.
- E. R. Zubarev, J. Xu, A. Sayyad and J. D. Gibson, J. Am. Chem. Soc., 2006, 128, 15098–15099 CrossRef CAS PubMed.
- R. B. Grubbs, Nat. Mater., 2007, 6, 553–555 CrossRef CAS PubMed.
- J. Hu, T. Wu, G. Zhang and S. Liu, J. Am. Chem. Soc., 2012, 134, 7624–7627 CrossRef CAS PubMed.
- C. Yi, S. Zhang, K. T. Webb and Z. Nie, Acc. Chem. Res., 2017, 50, 12–21 CrossRef CAS PubMed.
- J. Song, G. Niu and X. Chen, Bioconjugate Chem., 2017, 28, 105–114 CrossRef CAS PubMed.
- J. Song, J. Zhou and H. Duan, J. Am. Chem. Soc., 2012, 134, 13458–13469 CrossRef CAS PubMed.
- J. Song, Z. Fang, C. Wang, J. Zhou, B. Duan, L. Pu and H. Duan, Nanoscale, 2013, 5, 5816–5824 RSC.
- J. Song, L. Pu, J. Zhou, B. Duan and H. Duan, ACS Nano, 2013, 7, 9947–9960 CrossRef CAS PubMed.
- J. Song, X. Yang, O. Jacobson, L. Lin, P. Huang, G. Niu, Q. Ma and X. Chen, ACS Nano, 2015, 9, 9199–9209 CrossRef CAS PubMed.
- J. Song, P. Huang and X. Chen, Nat. Protoc., 2016, 11, 2287–2299 CrossRef CAS PubMed.
- Z. Nie, D. Fava, M. Rubinstein and E. Kumacheva, J. Am. Chem. Soc., 2008, 130, 3683–3689 CrossRef CAS PubMed.
- D. Fava, Z. Nie, M. A. Winnik and E. Kumacheva, Adv. Mater., 2008, 20, 4318–4322 CrossRef CAS.
- J. Lin, S. Wang, P. Huang, Z. Wang, S. Chen, G. Niu, W. Li, J. He, D. Cui, G. Lu, X. Chen and Z. Nie, ACS Nano, 2013, 7, 5320–5329 CrossRef CAS PubMed.
- J. He, Z. Wei, L. Wang, Z. Tomova, T. Babu, C. Wang, X. Han, J. T. Fourkas and Z. Nie, Angew. Chem., Int. Ed., 2013, 52, 2463–2468 CrossRef CAS PubMed.
- T. Bian, L. Shang, H. Yu, M. T. Perez, L.-Z. Wu, C.-H. Tung, Z. Nie, Z. Tang and T. Zhang, Adv. Mater., 2014, 26, 5613–5618 CrossRef CAS PubMed.
- Y. Liu, J. He, K. Yang, C. Yi, Y. Liu, L. Nie, N. M. Khashab, X. Chen and Z. Nie, Angew. Chem., Int. Ed., 2015, 54, 15809–15812 CrossRef CAS PubMed.
- K. J. M. Bishop, Angew. Chem., Int. Ed., 2016, 55, 1598–1600 CrossRef CAS PubMed.
- M. S. Nikolic, C. Olsson, A. Salcher, A. Kornowski, A. Rank, R. Schubert, A. Frömsdorf, H. Weller and S. Förster, Angew. Chem., 2009, 121, 2790–2792 CrossRef.
- Y. Guo, S. Harirchian-Saei, C. M. S. Izumi and M. G. Moffitt, ACS Nano, 2011, 5, 3309–3318 CrossRef CAS PubMed.
- A. D. Bangham and R. W. Horne, J. Mol. Biol., 1964, 8, IN2-668-IN10 CrossRef.
- A. D. Bangham, M. M. Standish and J. C. Watkins, J. Mol. Biol., 1965, 13, 238–IN27 CrossRef CAS PubMed.
- A. D. Bangham, Chem. Phys. Lipids, 1993, 64, 275–285 CrossRef CAS PubMed.
- T. M. Allen and P. R. Cullis, Adv. Drug Delivery Rev., 2013, 65, 36–48 CrossRef CAS PubMed.
- W. T. Al-Jamal and K. Kostarelos, Nanomedicine, 2007, 2, 85–98 CrossRef CAS PubMed.
- E. Amstad and E. Reimhult, Nanomedicine, 2011, 7, 145–164 CrossRef PubMed.
- J. N. Cha, H. Birkedal, L. E. Euliss, M. H. Bartl, M. S. Wong, T. J. Deming and G. D. Stucky, J. Am. Chem. Soc., 2003, 125, 8285–8289 CrossRef CAS PubMed.
- S. Lecommandoux, O. Sandre, F. Chécot, J. Rodriguez-Hernandez and R. Perzynski, Adv. Mater., 2005, 17, 712–718 CrossRef CAS.
- G. Gopalakrishnan, C. Danelon, P. Izewska, M. Prummer, P.-Y. Bolinger, I. Geissbühler, D. Demurtas, J. Dubochet and H. Vogel, Angew. Chem., Int. Ed., 2006, 45, 5478–5483 CrossRef CAS PubMed.
- S.-H. Park, S.-G. Oh, J.-Y. Mun and S.-S. Han, Colloids Surf., B, 2006, 48, 112–118 CrossRef CAS PubMed.
- L. Paasonen, T. Laaksonen, C. Johans, M. Yliperttula, K. Kontturi and A. Urtti, J. Controlled Release, 2007, 122, 86–93 CrossRef CAS PubMed.
- S.-H. Park, S.-G. Oh, K.-D. Suh, S.-H. Han, D. J. Chung, J.-Y. Mun, S.-S. Han and J.-W. Kim, Colloids Surf., B, 2009, 70, 108–113 CrossRef CAS PubMed.
- S.-H. Park, S.-G. Oh, J.-Y. Mun and S.-S. Han, Colloids Surf., B, 2005, 44, 117–122 CrossRef CAS PubMed.
- W. T. Al-Jamal, K. T. Al-Jamal, B. Tian, L. Lacerda, P. H. Bomans, P. M. Frederik and K. Kostarelos, ACS Nano, 2008, 2, 408–418 CrossRef CAS PubMed.
- L. Feng, X. Kong, K. Chao, Y. Sun, Q. Zeng and Y. Zhang, Mater. Chem. Phys., 2005, 93, 310–313 CrossRef CAS.
- W. Mueller, K. Koynov, K. Fischer, S. Hartmann, S. Pierrat, T. Basché and M. Maskos, Macromolecules, 2009, 42, 357–361 CrossRef CAS.
- G. D. Bothun, A. E. Rabideau and M. A. Stoner, J. Phys. Chem. B, 2009, 113, 7725–7728 CrossRef CAS PubMed.
- M. Krack, H. Hohenberg, A. Kornowski, P. Lindner, H. Weller and S. Förster, J. Am. Chem. Soc., 2008, 130, 7315–7320 CrossRef CAS PubMed.
- X. Zhang, Y. Yang, J. Tian and H. Zhao, Chem. Commun., 2009, 3807–3809 RSC.
- Y. Mai and A. Eisenberg, J. Am. Chem. Soc., 2010, 132, 10078–10084 CrossRef CAS PubMed.
- M. R. Rasch, E. Rossinyol, J. L. Hueso, B. W. Goodfellow, J. Arbiol and B. A. Korgel, Nano Lett., 2010, 10, 3733–3739 CrossRef CAS PubMed.
- M. R. Rasch, Y. Yu, C. Bosoy, B. W. Goodfellow and B. A. Korgel, Langmuir, 2012, 28, 12971–12981 CrossRef CAS PubMed.
- M. R. Preiss and G. D. Bothun, Expert Opin. Drug Delivery, 2011, 8, 1025–1040 CrossRef CAS PubMed.
- M. Schulz, A. Olubummo and W. H. Binder, Soft Matter, 2012, 8, 4849 RSC.
- S. J. Soenen, G. V. Velde, A. Ketkar-Atre, U. Himmelreich and M. De Cuyper, WIREs Nanomed. Nanobiotechnol., 2011, 3, 197–211 CrossRef CAS PubMed.
- E. Amstad, J. Kohlbrecher, E. Müller, T. Schweizer, M. Textor and E. Reimhult, Nano Lett., 2011, 11, 1664–1670 CrossRef CAS PubMed.
- D. Qiu, X. An, Z. Chen and X. Ma, Chem. Phys. Lipids, 2012, 165, 563–570 CrossRef CAS PubMed.
- D. Qiu and X. An, Colloids Surf., B, 2013, 104, 326–329 CrossRef CAS PubMed.
- K. Katagiri, Y. Imai, K. Koumoto, T. Kaiden, K. Kono and S. Aoshima, Small, 2011, 7, 1683–1689 CrossRef CAS PubMed.
- Q. Wei, T. Li, G. Wang, H. Li, Z. Qian and M. Yang, Biomaterials, 2010, 31, 7332–7339 CrossRef CAS PubMed.
- C. Sanson, O. Diou, J. Thévenot, E. Ibarboure, A. Soum, A. Brûlet, S. Miraux, E. Thiaudière, S. Tan, A. Brisson, V. Dupuis, O. Sandre and S. Lecommandoux, ACS Nano, 2011, 5, 1122–1140 CrossRef CAS PubMed.
- H. Oliveira, E. Pérez-Andrés, J. Thevenot, O. Sandre, E. Berra and S. Lecommandoux, J. Controlled Release, 2013, 169, 165–170 CrossRef CAS PubMed.
- R. J. Hickey, A. S. Haynes, J. M. Kikkawa and S.-J. Park, J. Am. Chem. Soc., 2011, 133, 1517–1525 CrossRef CAS PubMed.
- R. J. Hickey, J. Koski, X. Meng, R. A. Riggleman, P. Zhang and S.-J. Park, ACS Nano, 2014, 8, 495–502 CrossRef CAS PubMed.
- R. J. Hickey, Q. Luo and S.-J. Park, ACS Macro Lett., 2013, 2, 805–808 CrossRef CAS.
- Y. Liu, Y. Li, J. He, K. J. Duelge, Z. Lu and Z. Nie, J. Am. Chem. Soc., 2014, 136, 2602–2610 CrossRef CAS PubMed.
- Y. Liu, X. Yang, Z. Huang, P. Huang, Y. Zhang, L. Deng, Z. Wang, Z. Zhou, Y. Liu, H. Kalish, N. M. Khachab, X. Chen and Z. Nie, Angew. Chem., Int. Ed., 2016, 55, 15297–15300 CrossRef CAS PubMed.
- C. C. Berton-Carabin and K. Schroën, Annu. Rev. Food Sci. Technol., 2015, 6, 263–297 CrossRef CAS PubMed.
- K. L. Thompson, M. Williams and S. P. Armes, J. Colloid Interface Sci., 2015, 447, 217–228 CrossRef CAS PubMed.
- J. Huo, M. Marcello, A. Garai and D. Bradshaw, Adv. Mater., 2013, 25, 2717–2722 CrossRef CAS PubMed.
- X.-W. Xu, X.-M. Zhang, C. Liu, Y.-L. Yang, J.-W. Liu, H.-P. Cong, C.-H. Dong, X.-F. Ren and S.-H. Yu, J. Am. Chem. Soc., 2013, 135, 12928–12931 CrossRef CAS PubMed.
- Y.-S. Cho, G.-R. Yi, J.-M. Lim, S.-H. Kim, V. N. Manoharan, D. J. Pine and S.-M. Yang, J. Am. Chem. Soc., 2005, 127, 15968–15975 CrossRef CAS PubMed.
- Z. Rozynek and A. Józefczak, Eur. Phys. J.: Spec. Top., 2016, 225, 741–756 CrossRef CAS.
- P. Datskos, G. Polizos, M. Bhandari, D. A. Cullen and J. Sharma, RSC Adv., 2016, 6, 26734–26737 RSC.
- Y. Yin, R. M. Rioux, C. K. Erdonmez, S. Hughes, G. A. Somorjai and A. P. Alivisatos, Science, 2004, 304, 711–714 CrossRef CAS PubMed.
- B. Liu and H. C. Zeng, J. Am. Chem. Soc., 2004, 126, 16744–16746 CrossRef CAS PubMed.
- B. Liu and H. C. Zeng, J. Am. Chem. Soc., 2004, 126, 8124–8125 CrossRef CAS PubMed.
- B. Liu and H. C. Zeng, Chem. Mater., 2007, 19, 5824–5826 CrossRef CAS.
- M. in het Panhuis and V. N. Paunov, Chem. Commun., 2005, 1726 RSC.
- Y. Nonomura and N. Kobayashi, J. Colloid Interface Sci., 2009, 330, 463–466 CrossRef CAS PubMed.
- D. Wang, C. Song, Z. Hu and X. Fu, J. Phys. Chem. B, 2005, 109, 1125–1129 CAS.
- A. B. Subramaniam, J. Wan, A. Gopinath and H. A. Stone, Soft Matter, 2011, 7, 2600 RSC.
- L. Zhang, F. Zhang, Y.-S. Wang, Y.-L. Sun, W.-F. Dong, J.-F. Song, Q.-S. Huo and H.-B. Sun, Soft Matter, 2011, 7, 7375–7381 RSC.
- A. Kumar, B. J. Park, F. Tu and D. Lee, Soft Matter, 2013, 9, 6604–6617 RSC.
- N. Zhao and M. Gao, Adv. Mater., 2009, 21, 184–187 CrossRef CAS.
- H. Yang, F. Liang, Y. Chen, Q. Wang, X. Qu and Z. Yang, NPG Asia Mater., 2015, 7, e176 CrossRef CAS.
- C. H. J. Evers, J. A. Luiken, P. G. Bolhuis and W. K. Kegel, Nature, 2016, 534, 364–368 CrossRef PubMed.
- B. Samanta, D. Patra, C. Subramani, Y. Ofir, G. Yesilbag, A. Sanyal and V. M. Rotello, Small, 2009, 5, 685–688 CrossRef CAS PubMed.
- A. Kaiser, T. Liu, W. Richtering and A. M. Schmidt, Langmuir, 2009, 25, 7335–7341 CrossRef CAS PubMed.
- I. Akartuna, E. Tervoort, A. R. Studart and L. J. Gauckler, Langmuir, 2009, 25, 12419–12424 CrossRef CAS PubMed.
- H. Liu, C. Wang, Q. Gao, J. Chen, B. Ren, X. Liu and Z. Tong, Int. J. Pharm., 2009, 376, 92–98 CrossRef CAS PubMed.
- P. K. Jain and M. A. El-Sayed, Chem. Phys. Lett., 2010, 487, 153–164 CrossRef CAS.
- Y. Lin, H. Skaff, A. Böker, A. D. Dinsmore, T. Emrick and T. P. Russell, J. Am. Chem. Soc., 2003, 125, 12690–12691 CrossRef CAS PubMed.
- H. Skaff, Y. Lin, R. Tangirala, K. Breitenkamp, A. Böker, T. P. Russell and T. Emrick, Adv. Mater., 2005, 17, 2082–2086 CrossRef CAS.
- D. Patra, F. Ozdemir, O. R. Miranda, B. Samanta, A. Sanyal and V. M. Rotello, Langmuir, 2009, 25, 13852–13854 CrossRef CAS PubMed.
- Y. Jeong, B. Duncan, M.-H. Park, C. Kim and V. M. Rotello, Chem. Commun., 2011, 47, 12077 RSC.
- B. Radt, T. A. Smith and F. Caruso, Adv. Mater., 2004, 16, 2184–2189 CrossRef CAS.
- A. S. Angelatos, B. Radt and F. Caruso, J. Phys. Chem. B, 2005, 109, 3071–3076 CrossRef CAS PubMed.
- E. Glogowski, R. Tangirala, J. He, T. P. Russell and T. Emrick, Nano Lett., 2007, 7, 389–393 CrossRef CAS PubMed.
- D. Liu, F. Zhou, C. Li, T. Zhang, H. Zhang, W. Cai and Y. Li, Angew. Chem., Int. Ed., 2015, 54, 9596–9600 CrossRef CAS PubMed.
- S. Anandhakumar, M. Sasidharan, C.-W. Tsao and A. M. Raichur, ACS Appl. Mater. Interfaces, 2014, 6, 3275–3281 CAS.
- D. Radziuk, D. G. Shchukin, A. Skirtach, H. Möhwald and G. Sukhorukov, Langmuir, 2007, 23, 4612–4617 CrossRef CAS PubMed.
- G. Kaur, J. He, J. Xu, S. Pingali, G. Jutz, A. Böker, Z. Niu, T. Li, D. Rawlinson, T. Emrick, B. Lee, P. Thiyagarajan, T. P. Russell and Q. Wang, Langmuir, 2009, 25, 5168–5176 CrossRef CAS PubMed.
- P. van Rijn and A. Böker, J. Mater. Chem., 2011, 21, 16735 RSC.
- B. A. Rhodes, I. Zolle and H. N. Wagner, J. Clin. Res., 1968, 16, 245–250 Search PubMed.
- J. M. Gallo, C. T. Hung and D. G. Perrier, Int. J. Pharm., 1984, 22, 63–74 CrossRef CAS.
- R. Arshady, J. Controlled Release, 1990, 14, 111–131 CrossRef CAS.
- O. P. Tiourina and G. B. Sukhorukov, Int. J. Pharm., 2002, 242, 155–161 CrossRef CAS PubMed.
- N. G. Balabushevich, O. P. Tiourina, D. V. Volodkin, N. I. Larionova and G. B. Sukhorukov, Biomacromolecules, 2003, 4, 1191–1197 CrossRef CAS PubMed.
- Z. An, H. Möhwald and J. Li, Biomacromolecules, 2006, 7, 580–585 CrossRef CAS PubMed.
- Z. An, C. Tao, G. Lu, H. Möhwald, S. Zheng, Y. Cui and J. Li, Chem. Mater., 2005, 17, 2514–2519 CrossRef CAS.
- W. Tong, C. Gao and H. Möhwald, Colloid Polym. Sci., 2008, 286, 1103–1109 CAS.
- L. Duan, Q. He, X. Yan, Y. Cui, K. Wang and J. Li, Biochem. Biophys. Res. Commun., 2007, 354, 357–362 CrossRef CAS PubMed.
- W. Qi, X. Yan, L. Duan, Y. Cui, Y. Yang and J. Li, Biomacromolecules, 2009, 10, 1212–1216 CrossRef CAS PubMed.
- D. Mertz, P. Tan, Y. Wang, T. K. Goh, A. Blencowe and F. Caruso, Adv. Mater., 2011, 23, 5668–5673 CrossRef CAS PubMed.
- S. Fujii, A. Aichi, M. Muraoka, N. Kishimoto, K. Iwahori, Y. Nakamura and I. Yamashita, J. Colloid Interface Sci., 2009, 338, 222–228 CrossRef CAS PubMed.
- P. van Rijn, H. Park, K. Özlem Nazli, N. C. Mougin and A. Böker, Langmuir, 2013, 29, 276–284 CrossRef CAS PubMed.
- B. T. Nguyen, T. Nicolai and L. Benyahia, Langmuir, 2013, 29, 10658–10664 CrossRef CAS PubMed.
- Y. Song, U. Shimanovich, T. C. T. Michaels, Q. Ma, J. Li, T. P. J. Knowles and H. C. Shum, Nat. Commun., 2016, 7, 12934 CrossRef CAS PubMed.
- X. Huang, M. Li and S. Mann, Chem. Commun., 2014, 50, 6278–6280 RSC.
- X. Liu, P. Zhou, Y. Huang, M. Li, X. Huang and S. Mann, Angew. Chem., 2016, 128, 7211–7216 CrossRef.
- S. Mann, Acc. Chem. Res., 2012, 45, 2131–2141 CrossRef CAS PubMed.
- J. Fothergill, M. Li, S. A. Davis, J. A. Cunningham and S. Mann, Langmuir, 2014, 30, 14591–14596 CrossRef CAS PubMed.
- R. K. Kumar, M. Li, S. N. Olof, A. J. Patil and S. Mann, Small, 2013, 9, 357–362 CrossRef CAS PubMed.
- K. Akkarachaneeyakorn, M. Li, S. A. Davis and S. Mann, Langmuir, 2016, 32, 2912–2919 CrossRef CAS PubMed.
- M. Li, X. Huang and S. Mann, Small, 2014, 10, 3291–3298 CrossRef CAS PubMed.
- A. J. Worthen, L. M. Foster, J. Dong, J. A. Bollinger, A. H. Peterman, L. E. Pastora, S. L. Bryant, T. M. Truskett, C. W. Bielawski and K. P. Johnston, Langmuir, 2014, 30, 984–994 CrossRef CAS PubMed.
- T. Nallamilli, B. P. Binks, E. Mani and M. G. Basavaraj, Langmuir, 2015, 31, 11200–11208 CrossRef CAS PubMed.
- T. G. Mason, J. N. Wilking, K. Meleson, C. B. Chang and S. M. Graves, J. Phys.: Condens. Matter, 2006, 18, R635 CrossRef CAS.
- C. A. S. Batista, R. G. Larson and N. A. Kotov, Science, 2015, 350, 1242477 CrossRef PubMed.
- Q. Li, U. Jonas, X. S. Zhao and M. Kappl, Asia-Pac. J. Chem. Eng., 2008, 3, 255–268 CrossRef CAS.
- C. P. Whitby, D. Fornasiero, J. Ralston, L. Liggieri and F. Ravera, J. Phys. Chem. C, 2012, 116, 3050–3058 CAS.
- T. Bollhorst, T. Grieb, A. Rosenauer, G. Fuller, M. Maas and K. Rezwan, Chem. Mater., 2013, 25, 3464–3471 CrossRef CAS.
- S. Sihler, A. Schrade, Z. Cao and U. Ziener, Langmuir, 2015, 31, 10392–10401 CrossRef CAS PubMed.
- A. Schrade, Z. Cao, K. Landfester and U. Ziener, Langmuir, 2011, 27, 6689–6700 CrossRef CAS PubMed.
- S. Li, B. A. Moosa, J. G. Croissant and N. M. Khashab, Angew. Chem., 2015, 54, 6804–6808 CrossRef CAS PubMed.
- K. Chen, S. Zhou, S. Yang and L. Wu, Adv. Funct. Mater., 2015, 25, 1035–1041 CrossRef CAS.
- F. Caruso, R. A. Caruso and H. Möhwald, Chem. Mater., 1999, 11, 3309–3314 CrossRef CAS.
- F. Caruso and H. Möhwald, Langmuir, 1999, 15, 8276–8281 CrossRef CAS.
- R. A. Caruso, A. Susha and F. Caruso, Chem. Mater., 2001, 13, 400–409 CrossRef CAS.
- M. Chen, L. Wu, S. Zhou and B. You, Macromolecules, 2004, 37, 9613–9619 CrossRef CAS.
- M. Chen, S. Zhou, B. You and L. Wu, Macromolecules, 2005, 38, 6411–6417 CrossRef CAS.
- M. J. Percy, C. Barthet, J. C. Lobb, M. A. Khan, S. F. Lascelles, M. Vamvakaki and S. P. Armes, Langmuir, 2000, 16, 6913–6920 CrossRef CAS.
- M. J. Percy and S. P. Armes, Langmuir, 2002, 18, 4562–4565 CrossRef CAS.
- M. J. Percy, V. Michailidou, S. P. Armes, C. Perruchot, J. F. Watts and S. J. Greaves, Langmuir, 2003, 19, 2072–2079 CrossRef CAS.
- S. Fortuna, C. A. L. Colard, A. Troisi and S. A. F. Bon, Langmuir, 2009, 25, 12399–12403 CrossRef CAS PubMed.
- R. Chen, D. J. G. Pearce, S. Fortuna, D. L. Cheung and S. A. F. Bon, J. Am. Chem. Soc., 2011, 133, 2151–2153 CrossRef CAS PubMed.
- K. Isenbügel, H. Ritter, R. Branscheid and U. Kolb, Macromol. Rapid Commun., 2010, 31, 2121–2126 CrossRef PubMed.
- S. Zhou, A. Sugawara-Narutaki, S. Tsuboike, J. Wang, A. Shimojima and T. Okubo, Langmuir, 2015, 31, 13214–13220 CrossRef CAS PubMed.
- B. Y. Guan, L. Yu and X. W. (David) Lou, Adv. Mater., 2016, 28, 9596–9601 CrossRef CAS PubMed.
- F. Caruso, A. S. Susha, M. Giersig and H. Möhwald, Adv. Mater., 1999, 11, 950–953 CrossRef CAS.
- F. Caruso, M. Spasova, A. Susha, M. Giersig and R. A. Caruso, Chem. Mater., 2000, 13, 109–116 CrossRef.
- V. Salgueiriño-Maceira, M. Spasova and M. Farle, Adv. Funct. Mater., 2005, 15, 1036–1040 CrossRef.
- S.-L. Shen, W. Wu, K. Guo, H. Meng and J.-F. Chen, Colloids Surf., A, 2007, 311, 99–105 CrossRef CAS.
- P. Arosio, J. Thévenot, T. Orlando, F. Orsini, M. Corti, M. Mariani, L. Bordonali, C. Innocenti, C. Sangregorio, H. Oliveira, S. Lecommandoux, A. Lascialfari and O. Sandre, J. Mater. Chem. B, 2013, 1, 5317–5328 RSC.
- N. J. Halas, S. Lal, W.-S. Chang, S. Link and P. Nordlander, Chem. Rev., 2011, 111, 3913–3961 CrossRef CAS PubMed.
- M. Rycenga, C. M. Cobley, J. Zeng, W. Li, C. H. Moran, Q. Zhang, D. Qin and Y. Xia, Chem. Rev., 2011, 111, 3669–3712 CrossRef CAS PubMed.
- P. K. Jain, X. Huang, I. H. El-Sayed and M. A. El-Sayed, Acc. Chem. Res., 2008, 41, 1578–1586 CrossRef CAS PubMed.
- Z. Liang, A. Susha and F. Caruso, Chem. Mater., 2003, 15, 3176–3183 CrossRef CAS.
- M. Karg and T. Hellweg, J. Mater. Chem., 2009, 19, 8714 RSC.
- P. B. Landon, A. H. Mo, C. Zhang, C. D. Emerson, A. D. Printz, A. F. Gomez, C. J. DeLaTorre, D. A. M. Colburn, P. Anzenberg, M. Eliceiri, C. O’Connell and R. Lal, ACS Appl. Mater. Interfaces, 2014, 6, 9937–9941 CAS.
- J. Tian, L. Yuan, M. Zhang, F. Zheng, Q. Xiong and H. Zhao, Langmuir, 2012, 28, 9365–9371 CrossRef CAS PubMed.
- L. Cheng, A. Liu, S. Peng and H. Duan, ACS Nano, 2010, 4, 6098–6104 CrossRef CAS PubMed.
- L. Wu, U. Glebe and A. Böker, Polym. Chem., 2015, 6, 5143–5184 RSC.
- H. Deng, Y. Zhong, M. Du, Q. Liu, Z. Fan, F. Dai and X. Zhang, Theranostics, 2014, 4, 904–918 CrossRef CAS PubMed.
- Y. Zhang and H. Zhao, Langmuir, 2016, 32, 3567–3579 CrossRef CAS PubMed.
- K. Niikura, N. Iyo, T. Higuchi, T. Nishio, H. Jinnai, N. Fujitani and K. Ijiro, J. Am. Chem. Soc., 2012, 134, 7632–7635 CrossRef CAS PubMed.
- K. Niikura, N. Iyo, Y. Matsuo, H. Mitomo and K. Ijiro, ACS Appl. Mater. Interfaces, 2013, 5, 3900–3907 CAS.
- J. Wei, K. Niikura, T. Higuchi, T. Kimura, H. Mitomo, H. Jinnai, Y. Joti, Y. Bessho, Y. Nishino, Y. Matsuo and K. Ijiro, J. Am. Chem. Soc., 2016, 138, 3274–3277 CrossRef CAS PubMed.
- X. An, F. Zhang, Y. Zhu and W. Shen, Chem. Commun., 2010, 46, 7202–7204 RSC.
- R. M. Gorgoll, T. Tsubota, K. Harano and E. Nakamura, J. Am. Chem. Soc., 2015, 137, 7568–7571 CrossRef CAS PubMed.
- Y.-C. Yeh, R. Tang, R. Mout, Y. Jeong and V. M. Rotello, Angew. Chem., Int. Ed., 2014, 53, 5137–5141 CAS.
- F. Caruso and H. Möhwald, J. Am. Chem. Soc., 1999, 121, 6039–6046 CrossRef CAS.
- C. Schüler and F. Caruso, Macromol. Rapid Commun., 2000, 21, 750–753 CrossRef.
- F. Caruso, H. Fiedler and K. Haage, Colloids Surf., A, 2000, 169, 287–293 CrossRef CAS.
- F. Caruso and C. Schüler, Langmuir, 2000, 16, 9595–9603 CrossRef CAS.
- Y. Lvov and F. Caruso, Anal. Chem., 2001, 73, 4212–4217 CrossRef CAS PubMed.
- T. Li, Z. Niu, T. Emrick, T. P. Russell and Q. Wang, Small, 2008, 4, 1624–1629 CrossRef CAS PubMed.
- E. Phillips, O. Penate-Medina, P. B. Zanzonico, R. D. Carvajal, P. Mohan, Y. Ye, J. Humm, M. Gönen, H. Kalaigian, H. Schöder, H. W. Strauss, S. M. Larson, U. Wiesner and M. S. Bradbury, Sci. Transl. Med., 2014, 6, 260ra149 CrossRef PubMed.
- M. S. Bradbury, M. Pauliah, P. Zanzonico, U. Wiesner and S. Patel, WIREs Nanomed. Nanobiotechnol., 2016, 8, 535–553 CrossRef CAS PubMed.
- C. Caltagirone, A. Bettoschi, A. Garau and R. Montis, Chem. Soc. Rev., 2015, 44, 4645–4671 RSC.
- S. E. Kim, L. Zhang, K. Ma, M. Riegman, F. Chen, I. Ingold, M. Conrad, M. Z. Turker, M. Gao, X. Jiang, S. Monette, M. Pauliah, M. Gonen, P. Zanzonico, T. Quinn, U. Wiesner, M. S. Bradbury and M. Overholtzer, Nat. Nanotechnol., 2016, 11, 977–985 CrossRef CAS PubMed.
- Y. Pan, J.-P. Volkmer, K. E. Mach, R. V. Rouse, J.-J. Liu, D. Sahoo, T. C. Chang, T. J. Metzner, L. Kang, M. van de Rijn, E. C. Skinner, S. S. Gambhir, I. L. Weissman and J. C. Liao, Sci. Transl. Med., 2014, 6, 260ra148 CrossRef PubMed.
- H. Soo Choi, W. Liu, P. Misra, E. Tanaka, J. P. Zimmer, B. Itty Ipe, M. G. Bawendi and J. V. Frangioni, Nat. Biotechnol., 2007, 25, 1165–1170 CrossRef PubMed.
- D. Pissuwan, T. Niidome and M. B. Cortie, J. Controlled Release, 2011, 149, 65–71 CrossRef CAS PubMed.
- J. Dobson, Gene Ther., 2006, 13, 283–287 CrossRef CAS PubMed.
- Y.-X. J. Wang, Quant. Imaging Med. Surg., 2011, 1, 35–40 Search PubMed.
- R. Weissleder, M. Nahrendorf and M. J. Pittet, Nat. Mater., 2014, 13, 125–138 CrossRef CAS PubMed.
- S. Zanganeh, G. Hutter, R. Spitler, O. Lenkov, M. Mahmoudi, A. Shaw, J. S. Pajarinen, H. Nejadnik, S. Goodman, M. Moseley, L. M. Coussens and H. E. Daldrup-Link, Nat. Nanotechnol., 2016, 11, 986–994 CrossRef CAS PubMed.
- K. Zarschler, L. Rocks, N. Licciardello, L. Boselli, E. Polo, K. P. Garcia, L. De Cola, H. Stephan and K. A. Dawson, Nanomedicine, 2016, 12, 1663–1701 CAS.
- Y. Liu, J.-J. Yin and Z. Nie, Nano Res., 2014, 7, 1719–1730 CrossRef CAS.
- H. F. Krug and P. Wick, Angew. Chem., Int. Ed., 2011, 50, 1260–1278 CrossRef CAS PubMed.
- H. F. Krug, Angew. Chem., Int. Ed., 2014, 53, 12304–12319 CAS.
- A. Nel, T. Xia, L. Mädler and N. Li, Science, 2006, 311, 622–627 CrossRef CAS PubMed.
- M. Yu and J. Zheng, ACS Nano, 2015, 9, 6655–6674 CrossRef CAS PubMed.
- M. Longmire, P. L. Choyke and H. Kobayashi, Nanomedicine, 2008, 3, 703–717 CrossRef CAS PubMed.
- N. Khlebtsov and L. Dykman, Chem. Soc. Rev., 2011, 40, 1647–1671 RSC.
- N. Bertrand, J. Wu, X. Xu, N. Kamaly and O. C. Farokhzad, Adv. Drug Delivery Rev., 2014, 66, 2–25 CrossRef CAS PubMed.
- A. M. Smith, M. C. Mancini and S. Nie, Nat. Nanotechnol., 2009, 4, 710–711 CrossRef CAS PubMed.
- X. Huang, I. H. El-Sayed, W. Qian and M. A. El-Sayed, J. Am. Chem. Soc., 2006, 128, 2115–2120 CrossRef CAS PubMed.
- A. Agarwal, S. W. Huang, M. O’Donnell, K. C. Day, M. Day, N. Kotov and S. Ashkenazi, J. Appl. Phys., 2007, 102, 064701 CrossRef.
- S. Laurent, S. Dutz, U. O. Häfeli and M. Mahmoudi, Adv. Colloid Interface Sci., 2011, 166, 8–23 CrossRef CAS PubMed.
- B. Gleich and J. Weizenecker, Nature, 2005, 435, 1214–1217 CrossRef CAS PubMed.
- M. H. Pablico-Lansigan, S. F. Situ and A. C. S. Samia, Nanoscale, 2013, 5, 4040 RSC.
- H. E. Daldrup-Link, D. Golovko, B. Ruffell, D. G. DeNardo, R. Castaneda, C. Ansari, J. Rao, G. A. Tikhomirov, M. F. Wendland, C. Corot and L. M. Coussens, Clin. Cancer Res., 2011, 17, 5695–5704 CrossRef CAS PubMed.
- P. H. R. Keen, N. K. H. Slater and A. F. Routh, Langmuir, 2014, 30, 1939–1948 CrossRef CAS PubMed.
- C. Zhang, C. Hu, Y. Zhao, M. Möller, K. Yan and X. Zhu, Langmuir, 2013, 29, 15457–15462 CrossRef CAS PubMed.
- Y. Zhao, Y. Li, D. E. Demco, X. Zhu and M. Möller, Langmuir, 2014, 30, 4253–4261 CrossRef CAS PubMed.
- F. Porta and A. Kros, Part. Part. Syst. Charact., 2013, 30, 606–613 CrossRef CAS.
- M. Ray, R. Tang, Z. Jiang and V. M. Rotello, Bioconjugate Chem., 2015, 26, 1004–1007 CrossRef CAS PubMed.
- C. S. Kim, R. Mout, Y. Zhao, Y.-C. Yeh, R. Tang, Y. Jeong, B. Duncan, J. A. Hardy and V. M. Rotello, Bioconjugate Chem., 2015, 26, 950–954 CrossRef CAS PubMed.
- J. Hardie, Y. Jiang, E. R. Tetrault, P. C. Ghazi, G. Y. Tonga, M. E. Farkas and V. M. Rotello, Nanotechnology, 2016, 27, 374001 CrossRef PubMed.
- R. Tang, Z. Jiang, M. Ray, S. Hou and V. M. Rotello, Nanoscale, 2016, 8, 18038–18041 RSC.
- T.-Y. Liu and T. C. Huang, Acta Biomater., 2011, 7, 3927–3934 CrossRef CAS PubMed.
- H. De Oliveira, J. Thevenot and S. Lecommandoux, WIREs Nanomed. Nanobiotechnol., 2012, 4, 525–546 CrossRef CAS PubMed.
- L. Tang, X. Yang, Q. Yin, K. Cai, H. Wang, I. Chaudhury, C. Yao, Q. Zhou, M. Kwon, J. A. Hartman, I. T. Dobrucki, L. W. Dobrucki, L. B. Borst, S. Lezmi, W. G. Helferich, A. L. Ferguson, T. M. Fan and J. Cheng, Proc. Natl. Acad. Sci. U. S. A., 2014, 111, 15344–15349 CrossRef CAS PubMed.
- N. Chen, H. Wang, Q. Huang, J. Li, J. Yan, D. He, C. Fan and H. Song, Small, 2014, 10, 3603–3611 CrossRef CAS PubMed.
- J. J. Li, D. Hartono, C.-N. Ong, B.-H. Bay and L.-Y. L. Yung, Biomaterials, 2010, 31, 5996–6003 CrossRef CAS PubMed.
- P. Agostinis, K. Berg, K. A. Cengel, T. H. Foster, A. W. Girotti, S. O. Gollnick, S. M. Hahn, M. R. Hamblin, A. Juzeniene, D. Kessel, M. Korbelik, J. Moan, P. Mroz, D. Nowis, J. Piette, B. C. Wilson and J. Golab, Ca-Cancer J. Clin., 2011, 61, 250–281 CrossRef PubMed.
- P. L. Choyke, Sci. Transl. Med., 2014, 6, 260fs44 CrossRef PubMed.
- Y. Jeong, S. T. Kim, Y. Jiang, B. Duncan, C. S. Kim, K. Saha, Y.-C. Yeh, B. Yan, R. Tang, S. Hou, C. Kim, M.-H. Park and V. M. Rotello, Nanomedicine, 2016, 11, 1571–1578 CrossRef CAS PubMed.
- H.-Y. Huang, H.-L. Liu, P.-H. Hsu, C.-S. Chiang, C.-H. Tsai, H.-S. Chi, S.-Y. Chen and Y.-Y. Chen, Adv. Mater., 2015, 27, 655–661 CrossRef CAS PubMed.
- X. Cai, F. Yang and N. Gu, Theranostics, 2012, 2, 103–112 CrossRef CAS PubMed.
- S. Unnikrishnan and A. L. Klibanov, Am. J. Roentgenol., 2012, 199, 292–299 CrossRef PubMed.
- T. B. Brismar, D. Grishenkov, B. Gustafsson, J. Härmark, Å. Barrefelt, S. V. V. N. Kothapalli, S. Margheritelli, L. Oddo, K. Caidahl, H. Hebert and G. Paradossi, Biomacromolecules, 2012, 13, 1390–1399 CrossRef CAS PubMed.
- A. A. Barrefelt, T. B. Brismar, G. Egri, P. Aspelin, A. Olsson, L. Oddo, S. Margheritelli, K. Caidahl, G. Paradossi, L. Dähne, R. Axelsson and M. Hassan, EJNMMI Res., 2013, 3, 12 CrossRef PubMed.
- L. Duan, F. Yang, L. Song, K. Fang, J. Tian, Y. Liang, M. Li, N. Xu, Z. Chen, Y. Zhang and N. Gu, Soft Matter, 2015, 11, 5492–5500 RSC.
- B. Y. S. Kim, J. T. Rutka and W. C. W. Chan, N. Engl. J. Med., 2010, 363, 2434–2443 CrossRef CAS PubMed.
- V. J. Venditto and F. C. Szoka Jr., Adv. Drug Delivery Rev., 2013, 65, 80–88 CrossRef CAS PubMed.
- M. L. Etheridge, S. A. Campbell, A. G. Erdman, C. L. Haynes, S. M. Wolf and J. McCullough, Nanomedicine, 2013, 9, 1–14 CAS.
- E. Blanco, H. Shen and M. Ferrari, Nat. Biotechnol., 2015, 33, 941–951 CrossRef CAS PubMed.
- S. Wilhelm, A. J. Tavares, Q. Dai, S. Ohta, J. Audet, H. F. Dvorak and W. C. W. Chan, Nat. Rev. Mat., 2016, 1, 16014 CrossRef CAS.
- J. P. A. Ioannidis, PLoS Med., 2016, 13, e1002049 Search PubMed.
- M. Björnmalm, M. Faria and F. Caruso, J. Am. Chem. Soc., 2016, 138, 13449–13456 CrossRef PubMed.
-
R. Bawa, G. F. Audette and I. Rubinstein, Handbook of Clinical Nanomedicine: Nanoparticles, Imaging, Therapy and Clinical Applications, Pan Stanford, 2015 Search PubMed.
- R. A. Petros and J. M. DeSimone, Nat. Rev. Drug Discovery, 2010, 9, 615–627 CrossRef CAS PubMed.
- S. M. Moghimi, A. C. Hunter and J. C. Murray, Pharmacol. Rev., 2001, 53, 283–318 CAS.
- Y. Geng, P. Dalhaimer, S. Cai, R. Tsai, M. Tewari, T. Minko and D. E. Discher, Nat. Nanotechnol., 2007, 2, 249–255 CrossRef CAS PubMed.
- H. Cabral, Y. Matsumoto, K. Mizuno, Q. Chen, M. Murakami, M. Kimura, Y. Terada, M.
R. Kano, K. Miyazono, M. Uesaka, N. Nishiyama and K. Kataoka, Nat. Nanotechnol., 2011, 6, 815–823 CrossRef CAS PubMed.
- A. E. Nel, L. Mädler, D. Velegol, T. Xia, E. M. V. Hoek, P. Somasundaran, F. Klaessig, V. Castranova and M. Thompson, Nat. Mater., 2009, 8, 543–557 CrossRef CAS PubMed.
- J. A. Kim, C. Åberg, A. Salvati and K. A. Dawson, Nat. Nanotechnol., 2012, 7, 62–68 CrossRef CAS PubMed.
- J. V. Jokerst, T. Lobovkina, R. N. Zare and S. S. Gambhir, Nanomedicine, 2011, 6, 715–728 CrossRef CAS PubMed.
- S. Schöttler, G. Becker, S. Winzen, T. Steinbach, K. Mohr, K. Landfester, V. Mailänder and F. R. Wurm, Nat. Nanotechnol., 2016, 11, 372–377 CrossRef PubMed.
- S. Tenzer, D. Docter, J. Kuharev, A. Musyanovych, V. Fetz, R. Hecht, F. Schlenk, D. Fischer, K. Kiouptsi, C. Reinhardt, K. Landfester, H. Schild, M. Maskos, S. K. Knauer and R. H. Stauber, Nat. Nanotechnol., 2013, 8, 772–781 CrossRef CAS PubMed.
- A. Salvati, A. S. Pitek, M. P. Monopoli, K. Prapainop, F. B. Bombelli, D. R. Hristov, P. M. Kelly, C. Åberg, E. Mahon and K. A. Dawson, Nat. Nanotechnol., 2013, 8, 137–143 CrossRef CAS PubMed.
- S. Schöttler, K. Landfester and V. Mailänder, Angew. Chem., Int. Ed., 2016, 55, 2016 CrossRef PubMed.
- K. P. García, K. Zarschler, L. Barbaro, J. A. Barreto, W. O’Malley, L. Spiccia, H. Stephan and B. Graham, Small, 2014, 10, 2516–2529 CrossRef PubMed.
- D. F. Moyano, K. Saha, G. Prakash, B. Yan, H. Kong, M. Yazdani and V. M. Rotello, ACS Nano, 2014, 8, 6748–6755 CrossRef CAS PubMed.
- M. Benezra, E. Phillips, M. Overholtzer, P. B. Zanzonico, E. Tuominen, U. Wiesner and M. S. Bradbury, Small, 2015, 11, 1721–1732 CrossRef CAS PubMed.
- S. G. Elci, Y. Jiang, B. Yan, S. T. Kim, K. Saha, D. F. Moyano, G. Yesilbag Tonga, L. C. Jackson, V. M. Rotello and R. W. Vachet, ACS Nano, 2016, 10, 5536–5542 CrossRef CAS PubMed.
- A. Elsaesser and C. V. Howard, Adv. Drug Delivery Rev., 2012, 64, 129–137 CrossRef CAS PubMed.
- H. F. Krug, Angew. Chem., Int. Ed., 2014, 53, 12304–12319 CAS.
- E. Lepeltier, L. Nuhn, C.-M. Lehr and R. Zentel, Nanomedicine, 2015, 10, 3147–3166 CrossRef CAS PubMed.
- R. Toy and K. Roy, Bioengineering Translational Medicine, 2016, 1, 47–62 CrossRef.
- B. Lee Chung, M. J. Toth, N. Kamaly, Y. J. Sei, J. Becraft, W. J. M. Mulder, Z. A. Fayad, O. C. Farokhzad, Y. Kim and R. Langer, Nano Today, 2015, 10, 759–776 CrossRef CAS PubMed.
- C. de Souza Carvalho, N. Daum and C.-M. Lehr, Adv. Drug Delivery Rev., 2014, 75, 129–140 CrossRef CAS PubMed.
- N. Vogel, M. Retsch, C.-A. Fustin, A. del Campo and U. Jonas, Chem. Rev., 2015, 115, 6265–6311 CrossRef CAS PubMed.
- H. Zheng, R. K. Smith, Y. Jun, C. Kisielowski, U. Dahmen and A. P. Alivisatos, Science, 2009, 324, 1309–1312 CrossRef CAS PubMed.
- N. de Jonge and F. M. Ross, Nat. Nanotechnol., 2011, 6, 695–704 CrossRef CAS PubMed.
|
This journal is © The Royal Society of Chemistry 2017 |
Click here to see how this site uses Cookies. View our privacy policy here.