Pharmaceutical removal in synthetic human urine using biochar†
Received
24th August 2016
, Accepted 8th February 2017
First published on 14th February 2017
Abstract
This research addresses the potential for biochar to remove pharmaceuticals from synthetic urine, thereby allowing the treated urine to be used as a contaminant-free nutrient product. Four biochars and one activated carbon from different source materials were tested: activated coconut carbon, coconut shell, bamboo, southern yellow pine, and northern hardwood. Batch tests were conducted for 24 hours using different compositions of synthetic urine and secondary wastewater effluent with biochar doses of 0.8 and 40 g L−1 and an initial pharmaceutical concentration of 0.2 mmol L−1. Seven pharmaceuticals were tested in this study: acetylsalicylic acid, paracetamol, ibuprofen, naproxen, citalopram, carbamazepine, and diclofenac. Activated coconut carbon, bamboo, and southern yellow pine biochars had the highest pharmaceutical removal in urine compositions at 40 g L−1, adsorbing greater than 90% of each pharmaceutical. These biochars also demonstrated the ability to remove pharmaceuticals in the presence of nutrients, where the maximum removal of phosphorus and nitrogen was 36% by activated coconut carbon, 9% by bamboo, and 23% by southern yellow pine in all waste waters. Due to the high concentrations of nutrients naturally present in urine, there remains a high concentration of nitrogen and phosphorus after biochar treatment. The interactions between biochar, nutrients, and pharmaceuticals suggest that biochar has the ability to remove pharmaceuticals while maintaining nutrient concentrations in solution for future use as a nutrient product.
Water impact
Human urine contributes a significant load of nutrients and pharmaceuticals to wastewater, but a small fraction of the volumetric flow. Many wastewater treatment facilities do not recover nutrients or specifically target pharmaceuticals, which creates multiple impacts. Urine source separation and biochar treatment would remove pharmaceuticals, thereby enabling contaminant-free nutrient recovery and promoting a more sustainable approach to wastewater treatment.
|
1 Introduction
A shift towards low-cost, locally sourced materials for the treatment of wastewater must be considered in order to progress as a sustainable society. Wastewater can be viewed as a valuable resource with nutrients present (i.e., phosphorus, potassium, and nitrogen), however, because large volumes of wastewater are generated, it is not efficient to recover nutrients from a dilute waste stream.1 Furthermore, the majority of wastewater treatment processes do not address emerging contaminants such as pharmaceuticals,2 thus, directing the focus towards the treatment of a concentrated waste stream could reduce the loading of these contaminants to the environment while creating the opportunity to recover contaminant-free nutrients. Urine source separation, in which urine is collected and treated as a separate waste stream, is a novel approach for targeting high concentrations of nutrients and pharmaceuticals to reduce the overall loading to conventional wastewater facilities. On average, urine accounts for 1% of the conventional wastewater volumetric flow but contributes approximately 64% of pharmaceuticals, 80% of nitrogen, and 50% of phosphorus on a mass basis.3–5 The high concentration of nutrients in source separated urine presents an opportunity for separation and subsequent use of the urine, for example, as a nutrient product in agriculture.3,4 However, previous research confirms that pharmaceuticals such as carbamazepine were detected in human urine when the subjects consumed agricultural products irrigated with wastewater.6 Thus, the presence of pharmaceuticals in urine is a critical issue that needs to be addressed for the implementation of source separated urine as a nutrient-rich product.
Previous studies have investigated the use of ion exchange, membrane separation, advanced oxidation, and electrodialysis for the removal of pharmaceuticals from urine.7–12 These processes require engineered materials and chemical and energy inputs. There have been fewer previous studies on the effectiveness of low-cost materials and processes for pharmaceutical removal from urine. Activated carbon has been extensively studied in water and wastewater for sorption of trace organics and has shown to be very effective.13–17 The activation process for activated carbon increases its surface area and porosity which allows for increased adsorption capacity. However, in a life cycle assessment (LCA) conducted by Sparrevik et al., the activation process significantly increases the energy and resources necessary for production of activated carbon.18 Biochar, a carbonaceous residue formed from pyrolysis of waste materials, is often considered a carbon-negative precursor to activated carbon and on average is $1000 cheaper per ton ($246 per ton for unactivated biochar versus $1500 per ton for activated carbon).18–22 Originally, biochar was developed as a soil amendment for carbon sequestration and retention of nutrients to minimize leaching and pollution from fertilized soils.23 Numerous studies have been conducted on the uptake of nutrients such as phosphate and nitrate by biochar in aqueous solutions for application as a soil amendment.24–28 Furthermore, a study conducted by Pillai et al. found urea adsorption by microwave activated biochar in human urine, however, the adsorption of nutrients by unactivated biochar in source separated urine has not been investigated.29
The application of biochar has expanded beyond adsorption of nutrients to investigating the removal of contaminants such as dyes, metals, and pharmaceuticals in aqueous solution.15,27,30–35 For example, pharmaceutical removal by biochar was explored by Yao et al. when biochar was amended in soils for sulfamethoxazole removal.36 The biochar amended soils reduced sulfamethoxazole leaching from 60% to an average of approximately 8%.36 However, limited studies have been conducted on the removal of pharmaceuticals by biochar for water and wastewater treatment. Essandoh et al. studied the removal of salicylic acid and ibuprofen in aqueous solutions using pine wood biochar where the sorption capacity was determined to be 22.7 and 10.7 mg g−1, respectively.37 Sulfamethoxazole and warfarin removal was studied using eucalyptus and pinewood biochars. The results showed that high temperature biochars were able to perform similarly to commercial activated carbons.14 Thus, both studies exhibited the adsorptive properties of biochar for pharmaceuticals which displays its efficacy as an adsorbent.
Although research on nutrient uptake by biochar has been conducted, the findings are limited to aqueous solutions and a single study by activated biochar in urine. Similarly, previous research on pharmaceutical removal by biochar was performed in aqueous solutions with a narrow subset of pharmaceuticals. The physical–chemical properties of pharmaceuticals and nutrients are solution-dependent, therefore, the unique chemistry of urine must be considered for adsorption by biochar. To our knowledge, prior research has not evaluated the effectiveness of biochar adsorption properties in the presence of both pharmaceuticals and nutrients. Therefore, the overall goal of this research was to investigate the use of biochar for adsorption of pharmaceuticals from nutrients in source separated urine. This research includes three objectives: (1) evaluate the uptake by biochar of seven pharmaceuticals in fresh urine, hydrolyzed urine, and wastewater effluent, (2) determine the effect of the physical–chemical properties of (i) biochar and (ii) pharmaceuticals on adsorption, and (3) understand the ability of biochar to remove pharmaceuticals in the presence of nutrients in different waste streams.
2 Experimental
2.1 Pharmaceutical selection
The FDA has approved over 1400 new molecular entities for use as therapeutics as of 2013.38 Due to the vastness of the pharmaceutical industry and subsequent human use, there are numerous pharmaceuticals that are present in the environment, which makes treatment challenging. As a result, a representative subset of pharmaceuticals were chosen for this research based on previous studies conducted by Lienert et al. and the California Department of Public Health (Title 22 60320.220) for direct beneficial use.39,40 The former study categorized 42 pharmaceuticals from 22 therapeutic groups with a focus on toxicity and prevalence to understand the eco-toxicological hazard of these micropollutants. The study focused on urine as the waste water and was dependent upon human consumption, excretion, and the consequent eco-toxicological hazard. Some of the most toxic pharmaceuticals included non-steroidal anti-inflammatory drugs, analgesics, and antibiotics such as ibuprofen, diclofenac, acetylsalicylic acid, and amoxicillin. The latter study was not limited to pharmaceuticals and evaluated an array of ubiquitous micropollutants such as endocrine disrupting contaminants and organic chemicals that are present in water and wastewater sources for implications to direct potable reuse. Title 22 for direct beneficial use developed 9 categories of functional groups and sorted representative micropollutants based on active functional groups into each of the categories. The 9 functional group categories were hydroxyl aromatic, amino/aminoacyl aromatic, non-aromatic carbon–carbon, deprotonated amine, alkoxy polyaromatic, alkoxy aromatic, alkyl aromatic, saturated aliphatic, and nitroaromatic. In order to test a representative group of pharmaceuticals, the 25 most prevalent and toxic pharmaceuticals in urine from Lienert et al. were sorted based on structure into the 9 functional group categories from Title 22: direct beneficial reuse. As a result, seven of the nine categories were represented by pharmaceuticals with the saturated aliphatic and nitroaromatic groups not applicable. Following this framework, seven representative pharmaceuticals were chosen in this study: paracetamol, diclofenac, carbamazepine, citalopram, naproxen, acetylsalicylic acid, and ibuprofen. Paracetamol (Sigma-Aldrich) is a weakly acidic pharmaceutical that is also known as acetaminophen from the analgesic drug class. Acetylsalicylic acid (Acros Organics) is a weakly acidic pharmaceutical known as aspirin. Diclofenac sodium (MP Biomedicals), naproxen sodium (Sigma-Aldrich), and ibuprofen (Fluka Analytical) are all weakly acidic pharmaceuticals from the non-steroidal anti-inflammatory (NSAID) drug class. Carbamazepine (Acros Organics) is a weakly basic pharmaceutical from the anti-epileptic agent drug class. Citalopram hydrobromide (Spectrum Laboratories) is a weakly basic pharmaceutical from the selective serotonin reuptake inhibitor (SSRI) drug class. Individual stock solutions of each pharmaceutical were made in a 50% methanol/50% water solution and were spiked in fresh urine, hydrolyzed urine, and wastewater effluent at a concentration of 0.2 mmol L−1.
2.2 Synthetic urine and wastewater effluent
Three types of synthetic waste water were used in this study: fresh urine, hydrolyzed urine, and secondary wastewater effluent. The composition of fresh urine and hydrolyzed urine can vary significantly based on diet and other physiological factors, while wastewater effluent can vary based on treatment operation and community type. Thus, synthetic compositions were used in this study to maintain consistency. The compositions of synthetic fresh urine, synthetic hydrolyzed urine, and synthetic secondary wastewater effluent are shown in Table 3. The compositions of fresh urine and hydrolyzed urine were based on previous literature reports.4,41,42 The composition of synthetic wastewater effluent was used to represent biologically treated wastewater and was also adapted from previous literature reports.43,44
2.3 Biochars
Four biochars and one activated carbon were tested in this study: bamboo (Lewis Bamboo), activated coconut carbon (Siemens), southern yellow pine (US Biochar Green), coconut shell (Charcoal House LLC), and northern hardwood (Charcoal House LLC). The biochars were characterized by nitrogen adsorption–desorption using a Quantachrome NOVA 2200e instrument. Biochar samples were degassed under vacuum at 110 °C and then placed into a liquid nitrogen bath. The temperature of the bath was kept constant at approximately −196 °C during which finite volumes of dinitrogen gas were introduced. Surface characteristics such as pore size, pore volume, and surface area were determined by plotting the amount of gas adsorbed versus the relative equilibrium pressure. Surface area was determined by the Brunauer–Emmett–Teller (BET) equation. The biochars were crushed, dried, and sieved before use and were size-reduced to an 8 × 60 mesh size (0.25 mm–2.38 mm). Tabulated values of the biochar characteristics are given in Table 2.
2.4 Batch tests
Batch tests were conducted to evaluate the removal of pharmaceuticals and nutrients by biochar. The four biochars, one activated carbon, and seven pharmaceuticals were tested separately in triplicate. Two doses of biochar (0.8 g L−1 and 40 g L−1) were added to 125 mL of fresh urine, hydrolyzed urine, and wastewater effluent. All of the samples were placed on a mechanical shaker table at 300 rpm for 24 h based on previous studies.24,27,36,45 The fresh urine and hydrolyzed urine batches were filtered using 0.45 μm PVDF filters (Millipore Durapore) prior to analysis. The wastewater effluent was stepwise filtered using 1.5 μm GE Whatman Grade 934-AH filters and 0.45 μm PVDF filters prior to analysis. All samples were stored in amber glass bottles at 4 °C until analyzed. A control sample for each waste water was tested without the addition of pharmaceuticals or biochar to determine any changes in solution chemistry from mixing. Additional control samples included biochar in each waste water to determine the change in solution chemistry from biochar addition.
2.5 Analytical methods
The concentrations of paracetamol, diclofenac, carbamazepine, citalopram, naproxen, acetylsalicylic acid, and ibuprofen were analyzed on a UV–visible spectrophotometer (Hitachi High Technologies) using a 1 cm quartz cuvette following methods adapted from previous literature reports.45,46 The relatively high initial pharmaceutical concentration was due to analytical constraints. Wavelength scans were conducted for all of the pharmaceuticals to obtain the peak absorbance to analyze the unknown concentrations in the samples (see Table 1). A calibration curve was developed for each pharmaceutical individually with increasing concentrations of 0.003, 0.013, 0.025, 0.050, 0.10 and 0.20 mmol L−1. For all calibration curves, the coefficient of determination (R2) was greater than 0.98.
Table 1 Properties of the seven pharmaceuticals tested71–73
Pharmaceutical |
Chemical structure |
Functional group categories |
WVa (nm) |
Molecular weight (g mol−1) |
pKa |
Log Kow |
UV spectrophotometer wavelength at which pharmaceuticals were analyzed, determined in this study experimentally through wavelength scans.
|
Paracetamol CAS 103-90-2 |
|
Hydroxy aromatic |
243 |
151.2 |
9.38 |
0.46 |
Acetylsalicylic acid CAS 50-78-2 |
|
Alkoxy aromatic |
225 |
180.2 |
3.49 |
1.19 |
Diclofenac CAS 15307-79-6 |
|
Amino/acylamino aromatic |
275 |
318.1 |
4.15 |
4.51 |
Naproxen CAS 26159-54-2 |
|
Alkoxy poly-aromatic |
262 |
252.2 |
4.19 |
3.18 |
Ibuprofen CAS 31121-9304 |
|
Alkyl aromatic |
221 |
228.3 |
4.91 |
3.97 |
Carbamazepine CAS 298-46-4 |
|
Non-aromatic C C |
284 |
232.3 |
13.9 |
2.25 |
Citalopram CAS 59729-32-7 |
|
Deprotonated amine |
238 |
405.4 |
9.78 |
1.39 |
Table 2 Properties of the tested biochars
Biochar material |
Pyrolysis Ta (°C) |
Contact pHb |
BET surface areab (m2 g−1) |
Pore sizeb (Å) |
BJH pore volumeb (cm3 g−1) |
Provided by the manufacturer.
Determined experimentally in this study.
|
Bamboo (BB) |
315+ |
9.6 |
68.7 |
14.8 |
0.017 |
Activated coconut carbon (AC) |
Not available |
10.8 |
1120 |
10.1 |
0.083 |
Softwood pine (SW) |
550 |
8.2 |
313 |
10.6 |
0.028 |
Coconut shell (CS) |
400–500 |
8.3 |
13.0 |
11.0 |
0.021 |
Northern hardwood (HW) |
400–500 |
10.8 |
102 |
13.6 |
0.012 |
Table 3 Composition of synthetic fresh urine, hydrolyzed urine, and wastewater effluent4,43,74,75
Chemicals |
Fresh urine (mmol L−1) |
Fresh urine (mg L−1) |
Hydrolyzed urine (mmol L−1) |
Hydrolyzed urine (mg L−1) |
Wastewater effluent (mg L−1) |
pH |
6 |
6 |
9 |
9 |
7.5 |
Urea-N |
|
7003 |
— |
— |
— |
NH4-N |
— |
— |
500 |
7003 |
5.0 |
Cl− |
100 |
3545 |
100 |
3545 |
— |
PO4-P |
20 |
619 |
14 |
434 |
1.6 |
SO42− |
15 |
1441 |
15 |
1441 |
0.06 |
HCO3-C |
— |
— |
250 |
3003 |
3.0 |
Na+ |
94 |
1471 |
90 |
2391 |
— |
K+ |
40 |
1564 |
40 |
1564 |
2.7 |
Ca2+ |
4 |
160 |
— |
— |
— |
Mg2+ |
4 |
97.2 |
— |
— |
0.10 |
Beef extract |
— |
— |
— |
— |
1.8 |
Peptone |
— |
— |
— |
— |
2.7 |
Humic acid |
— |
— |
— |
— |
4.2 |
Tannic acid |
— |
— |
— |
— |
4.2 |
Sodium lignin sulfonate |
— |
— |
— |
— |
2.4 |
Sodium lauryl sulfate |
— |
— |
— |
— |
0.94 |
Acacia gum |
— |
— |
— |
— |
4.7 |
Arabic acid |
— |
— |
— |
— |
5 |
Phosphate concentrations were measured following the Standard Method 4500P ascorbic acid method (EPA 356.3) on a UV–visible spectrophotometer (Hitachi High Technologies) using a 1 cm quartz cuvette at 880 nm.47 The calibration curve for phosphate had increasing concentrations of 0, 0.15, 0.30, 0.60, and 1.2 mg L−1 as P. The R2 for all calibration curves was greater than 0.95. A 1000 mg L−1 stock solution was made using NaH2PO4 and diluted to 5 mg L−1 as P to develop the standards. Due to the low upper limit of the calibration curve, fresh urine, hydrolyzed urine, and wastewater effluent had to be diluted prior to analysis by 1
:
1000, 1
:
500, and 1
:
5, respectively. Phosphate concentrations were measured in all of the batch experiments, however, nitrogen was measured in a single batch.
A representative batch for total nitrogen was measured for fresh urine and hydrolyzed urine spiked with naproxen using a Shimadzu TOC-Vcph/TN analyzer with an ASI-V autosampler. Samples were analyzed in duplicate with less than 5% error in measurement and the calibration standard checks. An increasing concentration calibration curve (0, 5, 10, 15, 20, 30 mg L−1 as N) was made from 1000 mg L−1 N from a NaNO3 solution. Fresh urine, hydrolyzed urine, and wastewater effluent had to be diluted prior to analysis by 1
:
500, 1
:
200, and 1
:
10, respectively.
pH and conductivity measurements were taken after each test using an Accumet AB-15 + pH meter and an Orion Star A215 conductivity meter as shown in Tables S10–S16.† Prior to each use, the pH meter was calibrated using a pH 4, pH 7, and pH 10 buffer. The conductivity meter was calibrated prior to each use using increasing calibration points of 1413 μS cm−1, 12
900 μS cm−1, and 80
000 μS cm−1.
2.6 Data analysis
All samples were collected in triplicate where the mean and the standard deviation of the triplicate samples were calculated. Error bars on figures show one standard deviation of the triplicate samples. The trends of adsorption as described in sections 3 and 4 are defined as approximately equal (≈) if the percent removal difference between the variables (e.g., biochars, waste waters, pharmaceuticals) is within ±5%. However, the approximately equal is omitted if the majority of variables depict the same relationship. For example, if a biochar achieved 90% removal in hydrolyzed urine and 98% in fresh urine but 86% removal in wastewater, the trend is described as wastewater < hydrolyzed urine < fresh urine. In all other cases, the trends are described with greater than (>), less than (<) or approximately equal (≈).
Two-factor ANOVA with replication was conducted to determine if there was a significant difference (α = 0.05) in the pharmaceutical removal by biochars as well as the waste water compositions. The null hypotheses state that there is no statistically significant difference between i) pharmaceutical removal by the biochars and ii) the waste water compositions (p > 0.05). The alternative hypotheses state that there is a significant difference between i) pharmaceutical removal by the biochars and ii) the waste water compositions (p < 0.05).
3 Results
3.1 Pharmaceutical removal by biochar in synthetic waste waters
ASA, DCF, IBP, and NPX are negatively charged in fresh urine (pH 6), secondary wastewater effluent (pH 7.5), and hydrolyzed urine (pH 9). The order of decreasing removal by biochar at 40 g L−1 dose was activated coconut carbon ≈ southern yellow pine > bamboo > northern hardwood > coconut shell (Fig. 1). At the 40 g L−1 dose, greater than 90% removal of DCF was achieved by bamboo, activated coconut carbon, and southern yellow pine biochars in all waste waters. For IBP and NPX, more than 90% was removed by activated coconut carbon and southern yellow pine with bamboo achieving greater than 90% removal in fresh urine for NPX. For ASA, greater than 90% removal was achieved by activated coconut carbon in all compositions and southern yellow pine in fresh urine and wastewater effluent. The order of decreasing removal by biochar at 0.8 g L−1 dose was activated coconut carbon > southern yellow pine > bamboo > northern hardwood ≈ coconut shell (Fig. 2). At the 0.8 g L−1 dose, activated coconut carbon was the only biochar that achieved greater than 90% removal in all compositions.
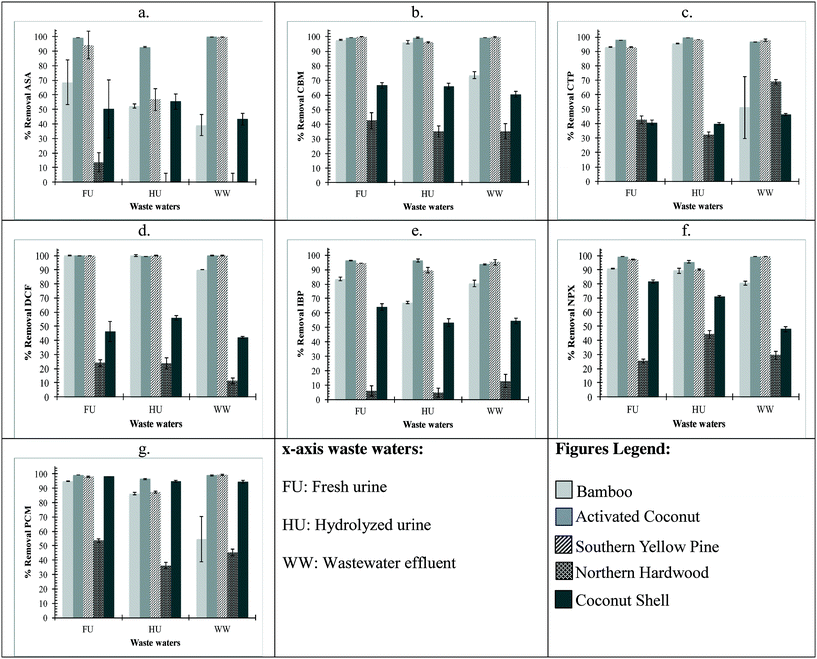 |
| Fig. 1 Pharmaceutical removal by biochar at 40 g L−1 dose and 300 rpm for 24 h (C0 = 0.2 mmol L−1). Pharmaceuticals: a. ASA, b. CBM, c. CTP, d. DCF, e. IBP, f. NPX, and g. PCM. Error bars indicate one standard deviation for triplicate samples. | |
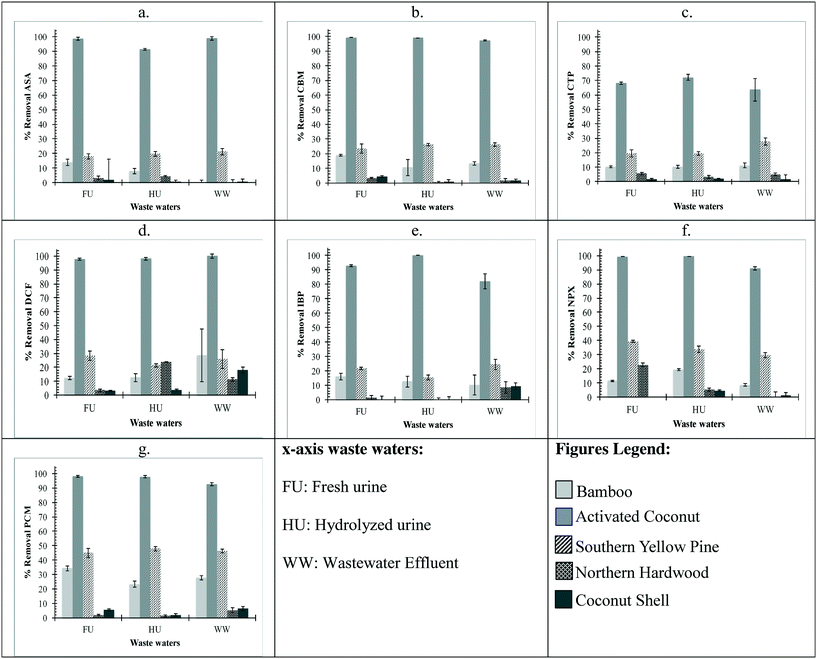 |
| Fig. 2 Pharmaceutical removal by biochar at 0.8 g L−1 dose and 300 rpm for 24 h (C0 = 0.2 mmol L−1). Pharmaceuticals: a. ASA, b. CBM, c. CTP, d. DCF, e. IBP, f. NPX, and g. PCM. Error bars indicate one standard deviation for triplicate samples. | |
PCM is neutral in fresh urine (pH 6) and wastewater effluent (pH 7.5), and partially deprotonates in hydrolyzed urine (pH 9) to give a total species distribution of 75% neutral and 25% negatively charged. The trend of decreasing PCM removal by biochar at 40 g L−1 dose was activated coconut carbon ≈ northern hardwood ≈ southern yellow pine > bamboo > coconut shell in all three waste waters (Fig. 1g). Considering all four biochars and one activated carbon, PCM removal was slightly higher in fresh urine than wastewater effluent or hydrolyzed urine. At the 40 g L−1 dose, activated coconut carbon achieved greater than 96% removal in all waste water types. Southern yellow pine achieved greater than 97% removal in fresh urine and wastewater effluent but less than 90% removal in hydrolyzed urine. Bamboo achieved 94% removal in fresh urine but less than 90% removal in hydrolyzed urine and wastewater effluent. The trend of decreasing PCM removal by biochar at 0.8 g L−1 dose was activated coconut carbon > southern yellow pine > bamboo > northern hardwood ≈ coconut shell (Fig. 2g). At the 0.8 g L−1 dose, PCM removal greater than 90% was achieved by activated coconut carbon alone.
CBM is neutral in fresh urine (pH 6), secondary wastewater effluent (pH 7.5), and hydrolyzed urine (pH 9). The trend of decreasing CBM removal by biochar at 40 g L−1 dose was activated coconut carbon ≈ southern yellow pine ≈ bamboo > northern hardwood > coconut shell (Fig. 1b). This trend was very consistent for all three waste waters with the exception of lower CBM removal by bamboo in secondary wastewater effluent than that in fresh urine or hydrolyzed urine. At the 40 g L−1 dose, greater than 99% removal was achieved by activated coconut carbon and greater than 96% removal was achieved by southern yellow pine. Bamboo achieved greater than 96% removal in fresh and hydrolyzed urine. The trend of decreasing CBM removal by biochar at 0.8 g L−1 dose was activated coconut carbon > southern yellow pine > bamboo > northern hardwood ≈ coconut shell (Fig. 2b). At the 0.8 g L−1 dose, activated coconut carbon was the only biochar to achieve greater than 97% removal for all compositions.
CTP is positively charged in fresh urine (pH 6), secondary wastewater effluent (pH 7.5), and hydrolyzed urine (pH 9). The trend of decreasing CTP removal by biochar at 40 g L−1 dose was activated coconut carbon ≈ southern yellow pine ≈ bamboo > northern hardwood ≈ coconut shell (Fig. 1c). This trend deviated for secondary wastewater effluent where CTP removal by bamboo was lower in wastewater effluent than that in fresh or hydrolyzed urine, and CTP removal by coconut shell was higher in wastewater effluent than that in fresh or hydrolyzed urine. At the 40 g L−1 dose, greater than 92% removal by activated coconut carbon and southern yellow pine was achieved in all waste waters while bamboo achieved greater than 90% removal in fresh urine and hydrolyzed urine. The trend of decreasing CTP removal by biochar at 0.8 g L−1 dose was activated coconut carbon > southern yellow pine > bamboo > northern hardwood ≈ coconut shell (Fig. 2c). At the 0.8 g L−1 dose, activated coconut carbon achieved less than 70% removal in urine and waste water.
The pharmaceutical loading on biochar was determined for both doses as shown in Tables S1 and S2 in the ESI.† Although the percent removal of pharmaceuticals was higher at the 40 g L−1 dose of biochar, the 0.8 g L−1 dose of biochar showed a higher pharmaceutical loading on the biochar. The overall decreasing trend for pharmaceutical loading on biochar was activated coconut carbon > southern yellow pine > bamboo > coconut shell ≈ northern hardwood. The adsorption of pharmaceuticals by activated coconut carbon and southern yellow pine is in agreement with respect to percent removal of the pharmaceuticals.
A two-factor ANOVA with replication statistical analysis was conducted to determine if there was a significant difference between the biochars for pharmaceutical removal in all waste water compositions. Table S4† reports the p-values for each pharmaceutical and biochar dose where factor i represents the statistical difference between biochars and factor ii represents the difference between waste water compositions. The results show that the factor i p-values for all of the pharmaceuticals is much lower than 0.05 (p < 0.05), therefore, there is a statistically significant difference between the biochars for removal of each pharmaceutical. Factor ii results show that there is a statistically significant difference in waste water composition for all of the pharmaceuticals, excluding CTP at the 40 g L−1 dose.
3.2 Nutrient removal by biochar in synthetic waste waters
The concentrations of nutrients as phosphate (PO4-P) and total nitrogen (TN) for each biochar at the 40 g L−1 dose after 24 h are shown in Fig. 3. The overall trends show that activated coconut carbon adsorbed the highest amount of PO4-P in all solutions with a removal of 332 mg L−1, 55.1 mg L−1, and 1.48 mg L−1 in fresh urine, hydrolyzed urine, and wastewater effluent, respectively. The decreasing phosphorus removal trend for fresh urine was activated coconut carbon > northern hardwood > southern yellow pine > bamboo > coconut shell, for hydrolyzed urine was activated coconut carbon > southern yellow pine > northern hardwood > coconut shell > bamboo, and for wastewater effluent was activated coconut carbon > northern hardwood > southern yellow pine > coconut shell > bamboo (Fig. 3 and S6–S8†). Bamboo showed release of phosphate in hydrolyzed urine and wastewater effluent with an addition of 27.0 mg L−1 and 19.1 mg L−1, respectively. As shown in Fig. 3, the phosphate concentrations in fresh urine have large error bars, on average, ±186 mg L−1. The urine species and concentrations were used as an input for Visual MINTEQ and the large error bars were attributed to precipitation of hydroxyapatite and struvite in solution. As all the biochars have contact pH values greater than 8, the addition of biochar to fresh urine increases the solution pH from a solution pH of 5.5 to greater than pH 6. This causes the precipitation of phosphate constituents, thus resulting in variable phosphate concentrations. Previous research has also confirmed that the precipitation of struvite begins at a pH of 7.2 where hydroxyapatite precipitation follows once struvite has reduced magnesium concentration in solution.48
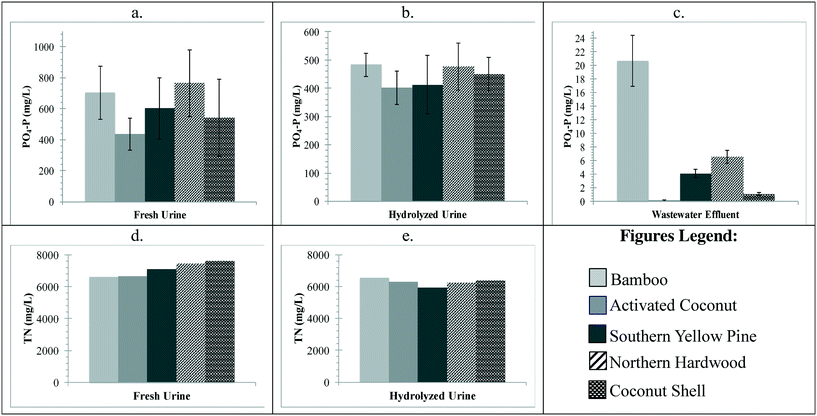 |
| Fig. 3 Phosphate concentrations upon completion of batch tests at 40 g L−1 dose in a. fresh urine (C0 = 619 mg L−1 PO4-P), b. hydrolyzed urine (C0 = 433 mg L−1 PO4-P), and c. wastewater effluent (C0 = 1.54 mg L−1 PO4-P). Error bars indicate one standard deviation from seven sets of triplicate samples. Nitrogen removal by biochar for representative batches in d. fresh urine (C0 = 7362 mg L−1 TN) and e. hydrolyzed urine (C0 = 7092 mg L−1 TN). | |
The average initial measured PO4-P concentrations and calculated phosphorus speciation were 622 mg L−1 and 70% H2PO4−/10% HPO42− in fresh urine, 438 mg L−1 and 72% H2PO4−/.04% HPO42− in hydrolyzed urine, and 1.52 mg L−1 and 68% H2PO4−/32% HPO42− in secondary wastewater effluent. In fresh urine, activated coconut carbon showed a 40% reduction in PO4-P concentration. Southern yellow pine and northern hardwood showed a reduction in PO4-P concentration of 1.5% to 29%; however, the variability in PO4-P concentration measurements resulted in error bars overlapping with the initial PO4-P concentration. Bamboo showed release of phosphate in hydrolyzed urine and wastewater effluent with an addition of 27.0 mg L−1 and 19.1 mg L−1, respectively. In hydrolyzed urine, the variability in PO4-P concentration measurements resulted in all biochars overlapping with the initial PO4-P concentration.
Fresh urine, hydrolyzed urine, and wastewater effluent initially contained 7362 mg L−1 as N of urea, 7092 mg L−1 as N of total ammonia (NH3 and NH4+), and 5.0 mg L−1 as N of total ammonia, respectively. The decreasing trend for TN removal in fresh urine was bamboo > activated coconut carbon > southern yellow pine > northern hardwood > coconut shell (Fig. 3). The decreasing trend for TN removal in hydrolyzed urine was southern yellow pine > activated coconut carbon > coconut shell > northern hardwood > bamboo (Fig. 3 and S9†). Because of the high concentrations of TN in urine, the biochars removed less than 10% TN in fresh urine and less than 20% TN in hydrolyzed urine. TN in wastewater effluent was not measured due to the low concentrations of nitrogen initially (0.36 mmol L−1).
4 Discussion
4.1 Effect of biochar properties
Understanding the separation of pharmaceuticals and nutrients in urine and wastewater cannot be explained by a single biochar property, but rather a combination of multiple properties that are influenced by solution conditions. The aromaticity of the biochar surface promotes van der Waals interactions with the pharmaceuticals via π–π effects. The biochars analyzed in this study were from different source materials as well as different pyrolysis temperatures, which resulted in varied surface properties as shown in Table 2. The three major biopolymers that are altered through the pyrolysis of biochar are lignin, hemicellulose, and cellulose as shown in Table S5.†49–51 As the materials are heated during pyrolysis, the surface initially contains aliphatic domains that are transformed to aromatic domains with heat input.52 Lignin has an aromatic structure that contributes to the aromaticity of biochar surfaces but is more recalcitrant to pyrolysis than hemicellulose and cellulose.53 At lower pyrolysis temperatures, the surface has an increased amount of functional groups that allow for electrostatic interactions and hydrogen bonding interactions with O and H groups.35 The presence of functional groups on the biochar surface can also generate a net charge for the biochar. Table 2 shows the contact pH of each of the biochars as determined by ASTM D6851 - 02.54,55 At a solution pH less than the contact pH for a given biochar, the surface of the biochar is positively charged. At a solution pH greater than the contact pH for a given biochar, the surface of the biochar is negatively charged.56 As a result, biopolymer makeup and pyrolysis temperature have effects on the surface attributes.
Surface area indicates the extent of porous structure that is available for adsorption where a higher surface area can be indicative of increased adsorption ability that will retain pharmaceuticals.57 The order of decreasing surface area for the biochars was activated coconut carbon (1120 m2 g−1) > southern yellow pine (313 m2 g−1) > northern hardwood (102 m2 g−1) > bamboo (68.7 m2 g−1) > coconut shell (13.0 m2 g−1). Activated coconut carbon is a commercial activated carbon, hence the larger surface area in comparison to the unactivated biochars. Fig. S2† shows the percent removal of the 7 pharmaceuticals as a function of surface area. Overall, as the surface area of the biochar increases, the adsorption of the pharmaceuticals increases excluding the northern hardwood biochar.
Adsorption is a function of the pore structure and total pore volume. The biopolymer makeup affects the pore structure in that higher hemicellulose/cellulose content leads to a more microporous structure, whereas higher lignin content leads to a more mesoporous structure.58 For example, the unactivated biochar with the greatest affinity for pharmaceuticals is southern yellow pine which contains 32% and 41% hemicellulose and cellulose, respectively. The total pore volume is determined at the highest pressure to estimate the amount of nitrogen adsorbed and relates the biochars' porous gas volume to total volume. The Barrett–Joyner–Halenda (BJH) pore volume, on the other hand, utilizes the N2 adsorption data and determines the mesoporous pore volume which is essential for diffusion of larger organic contaminants such as pharmaceuticals through the pore network.59 Biochars with larger BJH volumes, i.e., activated coconut carbon and southern yellow pine in this work, will have a greater affinity to adsorb the bulky pharmaceuticals than biochars with lower BJH volumes such as northern hardwood. As shown in Fig. S2,† northern hardwood has a higher surface area than bamboo, yet shows a lower adsorption capacity due to the lower BJH volume.
Three mechanisms that promote pharmaceutical removal by biochar are van der Waals interactions, electrostatic interactions, and hydrogen bonding. Previous studies have shown that biochar surfaces have complex surface chemistry with localized aromaticity as well as the presence of functional groups.53,60–62 Since all of the pharmaceuticals studied have at least one aromatic ring, the aromatic rings of the biochar and pharmaceuticals interact via π–π interactions. The π-electron-rich regions on the biochar surface interact with the π-electron-poor regions of the pharmaceuticals.16 Moreover, the functional groups on the surface result in a charge differential (biochar surface versus pharmaceutical charge) that allows for ion exchange mechanisms or electrostatic interactions. The functionality of the biochar surface also creates the possibility for hydrogen bonding with both the pharmaceuticals and nutrients. Each of the biochars is unique with different biopolymer makeup, pyrolysis temperature, and surface characteristics, which can help explain the difference in pharmaceutical and nutrient removal from solution.
4.1.1 Pharmaceutical removal by biochar.
The order of decreasing pharmaceutical removal by biochar established in this research was activated carbon > southern yellow pine > bamboo > northern hardwood > coconut shell considering all pharmaceuticals, waste waters, and biochar doses. Activated coconut had the greatest affinity for pharmaceuticals due its physical–chemical properties. The pyrolysis temperature was not documented, however, since activated coconut carbon is known to undergo an activation step, this biochar contains a high carbon content with less functionality, high microporous surface area, and large pore volume.63 Activated coconut carbon has a contact pH of 10.8 that results in a positively charged surface in all waste waters. Thus, the charge differential and electrostatic interactions promote removal of negatively charged pharmaceuticals such as NPX, IBP, ASA, and DCF. Moreover, hydrogen bonding between the pharmaceutical and biochar functional groups is a plausible mechanism prompting removal which will be further discussed in section 4.3. Activated coconut carbon showed adsorption of the neutral and positively charged pharmaceuticals CBM and CTP, respectively, as well. The adsorption of CBM and CTP can be attributed to the aromatic rings present in both the pharmaceutical and the biochar that allow for van der Waals interactions.
Southern yellow pine is a softwood biochar with a high cellulose content that promotes the formation of a microporous structure while still maintaining aromatic features on the surface due to pyrolysis. The pyrolysis temperature of southern yellow pine is 550 °C which is obtained via fast pyrolysis. Southern yellow pine had the second largest surface area and pore volume and consistently showed the second highest removal of pharmaceuticals from the waste waters. Southern yellow pine has a contact pH of 8.24 that makes the surface positively charged in fresh urine and wastewater effluent, but negatively charged in hydrolyzed urine. As shown in Fig. 1, the percent removal of CTP is greater for southern yellow pine in hydrolyzed urine than that in fresh urine, which can be attributed to the attraction of the positively charged pharmaceutical to the negatively charged biochar surface. Similarly, the lower percent removal of negatively charged pharmaceuticals (e.g., NPX, ASA, IBP, and DCF) in hydrolyzed urine is due to the reduced attractive electrostatic interactions by the less positively charged biochar surface. Fig. 1a shows that the ASA percent removal by the southern yellow pine biochar is much lower in hydrolyzed urine than those of the other pharmaceuticals. This is not only due to the less positively charged biochar surface, but also due to the hydrophilic nature of ASA at high pH, which will be discussed further in section 4.2.
Bamboo ranks fourth in terms of surface area and pore volume, but performs comparable to southern yellow pine, which has much higher surface area and pore volume. The similar performance of bamboo is due to the higher pyrolysis temperature, which increases the aromaticity of the surface and van der Waals interactions. Furthermore, pharmaceuticals need to enter the pore network and the larger pore size of bamboo allows for diffusion into the mesoporous and microporous regions. When comparing bamboo to northern hardwood, which has similar properties, the bamboo biochar had a lower surface area but has a larger BJH volume that is indicative of mesoporosity and small macroporosity.59 Thus, the majority of the total pore volume for bamboo is microporous that allows for enhanced pharmaceutical adsorption. Northern hardwood had a higher surface area and microporous pore volume than bamboo, but did not show similar removal. The variability in the removal by northern hardwood can be attributed to the lower BJH mesoporous volume as well as the higher lignin and ash content of hardwood-derived biochars that decrease its adsorptive properties.58 Coconut shell has the lowest surface area and pyrolysis temperature, and exhibits greater than 50% removal of neutral (CBM and PCM in fresh urine) and some negatively charged pharmaceuticals (NPX, IBP, and PCM in hydrolyzed urine). Its contact pH of 8.3 results in a net positively charged biochar surface in fresh urine, which explains the adsorption of negatively charged pharmaceuticals, i.e., NPX. The surface charge has a minimal effect on PCM that is neutral in fresh urine and partially negatively charged in hydrolyzed urine. Coconut shell has the highest lignin biopolymer content that contributes to the aromaticity of the biochar surface that could promote van der Waals interactions with neutral pharmaceuticals and negatively charged pharmaceuticals with two aromatic rings such as NPX.
It is important to note that as shown in Tables S1 and S2,† the biochar doses chosen in this study were not optimized but rather chosen to cover a wide range and based on previous research.29,31,36 There is a higher pharmaceutical loading at the 0.8 g L−1 dose than that at the 40 g L−1 dose indicating excess biochar dosing at the 40 g L−1 dose. The 40 g L−1 dose maintains a high percent removal of pharmaceuticals, however, the low pharmaceutical loading shows that there are unoccupied adsorption sites. Thus, biochar dose should be considered to ensure maximum adsorption per gram of adsorbent.
4.1.2 Nutrient removal by biochar.
Activated coconut carbon had the greatest removal of phosphate in all waste waters. This is a key finding in that co-removal of pharmaceuticals and nutrients would not be beneficial for nutrient recovery. Activated coconut carbon has a positively charged surface in all waste waters, indicating the affinity for the negatively charged phosphate (H2PO4−, HPO42−, PO43−) to adsorb to the surface. When comparing the phosphate removal in different waste waters, phosphate removal had a larger standard deviation in fresh urine versus hydrolyzed urine. Hydrolyzed urine, in contrast to fresh urine, is a buffered solution at a pH of 9 with no calcium or magnesium present, thus, the precipitation of phosphate minerals is unlikely. Phosphate is primarily adsorbed by the ion exchange mechanisms through inner sphere and outer sphere complexation.64 Phosphate was adsorbed by the biochar in all waste water compositions which indicates the presence of functional groups on the biochar surface that remove phosphate. The larger variation of phosphate constituents in wastewater can also be attributed to the release of phosphate by biochar, specifically, bamboo biochar.
The nitrogen constituents in urine are positively charged (NH4+) or neutral with lone pairs of electrons (i.e., urea and NH3) that can interact with the biochar surface, however, limited interactions were expected. Nitrogen is the most abundant nutrient in urine which contains approximately 7000 mg L−1 as N. Similar to phosphate removal, activated coconut carbon and southern yellow pine decreased the amount of nitrogen in fresh urine by approximately 10%. Southern yellow pine removed 19% of TN in hydrolyzed urine due to the negatively charged surface that promoted electrostatic interactions with the positively charged and neutral nitrogen species (NH3/NH4+). Moreover, both NH3 and NH4+ have the capability to hydrogen bond with the biochar surface functional groups. With a pKa of 9.4, there is a slightly higher fractional composition of NH3versus NH4+ in hydrolyzed urine. Although activated coconut is also characterized by a high surface area and the presence of micropores, similar results were not observed. The higher contact pH of activated coconut carbon results in a net positively charged surface that causes repulsion of ammonium and minimal interaction with ammonia in hydrolyzed urine. However, in fresh urine, the prominent nitrogen species is neutral urea, thus, activated coconut carbon exhibited higher removal than southern yellow pine. Due to the abundance of nitrogen in urine, the concentration of nitrogen remaining in solution was more than 6600 mg L−1 as N and 6000 mg L−1 as N in fresh and hydrolyzed urine, respectively. The high concentration of nutrients remaining in solution is favorable from the nutrient recovery perspective.
4.2 Effect of waste water composition
Fresh urine, hydrolyzed urine, and wastewater effluent have varying solution chemistry with different pH values and ionic strengths. Hydrolyzed urine (I = 0.47 mol L−1) has the highest ionic strength followed by fresh urine (I = 0.15 mol L−1) and finally wastewater effluent (I = 0.0004 mol L−1). Generally, an increase in ionic strength favors adsorption and electrostatic interactions are reduced due to screening effects.65,66 As a result, the greatest adsorption should occur in hydrolyzed urine, however, this trend does not hold for pharmaceutical or nutrient removal. The trends show that there is no specific pattern for pharmaceutical adsorption based on the ionic strength, but, in fact, the effect of solution chemistry is multifaceted with dependence on both pharmaceutical and biochar characteristics.
The two-factor ANOVA statistical analysis as shown in Table S5† displays the p-values for factor ii which was conducted for the statistically significant difference between waste water compositions for each pharmaceutical, excluding CTP at 40 mg L−1 dose. The results show that there was a statistically significant difference between fresh urine, hydrolyzed urine and wastewater effluent for pharmaceutical removal by biochar. These findings indicate that although a conclusive trend based on ionic strength was not established, the waste water composition does influence adsorption by biochar.
4.3 Pharmaceutical and nutrient adsorption
The phosphorus species and 4 of the pharmaceuticals (DCF, NPX, IBP, ASA) carry a net negative charge. Urea in fresh urine, ammonia in hydrolyzed urine, and CBM are neutral. Finally, ammonium in hydrolyzed urine and CTP in all compositions are positively charged. Consistent for all waste waters, the biochars adsorb both pharmaceuticals and nutrients. The adsorption of pharmaceuticals is due to the nonpolar moieties of the pharmaceuticals that further promote adsorption through van der Waals interactions, specifically π–π effects. A previous literature report has found that if the charge of the compound is comparable, e.g. DCF and H2PO4−, the nonpolar portion of the organic compound enhances selectivity.67 Polarity is not the same as polarizability, which takes into account the electron distribution as well as the chemical structure of molecules. Previous studies have developed linear free energy relationships that show the hydrophobicity of a compound exhibiting higher affinity for the hydrophobic organic surfaces,17,68 however, since many of these pharmaceuticals have ionizable fractions, the hydrophobicity of these compounds is pH-dependent as indicated by the octanol–water distribution coefficient (log
D).8,69 The octanol–water distribution coefficient (log
D) takes into consideration the Kow, pKa, and pH which account for the difference in log
D values. The trends for hydrophobicity (log
D) accounting for ionization of pharmaceutical functional groups at different pH values are shown in Fig. S1.† The trend of increasing hydrophobicity in fresh urine follows the order: ASA < PCM < CTP < NPX < IBP < CBM < DCF. In the wastewater effluent, the trend of increasing hydrophobicity is ASA < NPX < PCM < IBP < DCF < CTP < CBM. For hydrolyzed urine, the trend of increasing hydrophobicity is ASA < IBP < PCM < NPX < DCF < CBM < CTP. The log
D calculations were completed as prescribed in previous research.70 The specific results are further explained in the ESI.† Understanding the hydrophobicity of the pharmaceuticals gives insight into the van der Waals interactions between aromatic rings and the biochar surface that dictate removal.
The results in Tables S1, S2 and S6–S9† show the pharmaceutical and nutrient loading on the 4 biochars and activated carbon. The results show that the biochars adsorb both pharmaceuticals and nutrients, where the phosphate and nitrogen loadings (mg g−1) are greater than the pharmaceutical loading. This is due to the urine matrix with high concentrations of nutrients in contrast to low concentrations of pharmaceuticals that results in low percent removal and high percent removal, respectively. Nonetheless, these findings indicate that the diverse properties of biochar allow for removal of both nutrients and pharmaceuticals via van der Waals interactions, electrostatic interactions and hydrogen bonding.
5 Conclusions
Results from the batch tests showed that unactivated biochars can remove more than 90% of pharmaceuticals at high biochar doses (on the order of 10 g L−1) while maintaining less than 20% co-removal of phosphate and nitrogen species found in urine and wastewater. The outcomes of this work advance the knowledge in three specific areas: the chemistry of pharmaceuticals, the physical–chemical interactions between biochar and pharmaceuticals, and subsequent nutrient and pharmaceutical adsorption in urine using biochar. The adsorption of pharmaceuticals and nutrients by biochar in urine cannot be attributed to a single factor, e.g., surface area or pharmaceutical structure, but rather a combination of multiple superposing factors, e.g., pharmaceutical chemical structure and hydrophobicity, biochar surface area, pyrolysis temperature and biopolymer makeup, and waste water pH and chemistry. Biochar is a non-engineered material, therefore, the unique combination of biochar properties, e.g., biopolymer makeup and pyrolysis temperature, results in varied surface characteristics such as pore size, surface area and functionality that allow adsorption of pharmaceuticals and nutrients. The hydrophobicity of a pharmaceutical varies with solution chemistry and has a direct relationship with the adsorption by biochar, where increasing hydrophobicity results in greater removal by biochar. As a result, urine chemistry should be considered when targeting removal of different pharmaceuticals. Adsorption of pharmaceuticals with varying degrees of aromaticity and functional groups suggests that other pharmaceuticals with comparable structures will also be removed from solution by biochar. The similar performance by biochar in different waste water compositions shows the flexibility of biochar to be applied in different treatment settings, e.g., immediately for treatment of fresh urine, after storage for treatment of hydrolyzed urine, or after transport to a central location for treatment of wastewater effluent. The removal of both nutrients and pharmaceuticals suggests that biochar can act as an alternative sorbent to result in a micropollutant-free nutrient product for agriculture.
Acknowledgements
This publication is based upon work supported by the National Science Foundation, NSF CAREER grant CBET-1150790. Any opinions, findings, conclusions or recommendations expressed in this publication are those of the authors and do not necessarily reflect the views of NSF. We thank Lewis Bamboo for providing the bamboo biochar as well as Padmini Persaud for assistance with laboratory experiments and Regina Rodriguez for conducting biochar analysis. This article was improved by the comments of two thoughtful anonymous reviewers.
References
- J. Wilsenach and M. van Loosdrecht, Water Sci. Technol., 2003, 48, 103–110 CAS.
- A. Jelic, M. Gros, A. Ginebreda, R. Cespedes-Sanchez, F. Ventura, M. Petrovic and D. Barcelo, Water Res., 2011, 45, 1165–1176 CrossRef CAS PubMed.
- J. Lienert, T. Burki and B. I. Escher, Water Sci. Technol., 2007, 56, 87–96 CrossRef CAS PubMed.
- J. A. Wilsenach, C. A. Schuurbiers and M. C. van Loosdrecht, Water Res., 2007, 41, 458–466 CrossRef CAS PubMed.
-
K. M. Udert, 2002, DOI:10.3929/ethz-a-004541820.
- O. Paltiel, G. Fedorova, G. Tadmor, G. Kleinstern, Y. Maor and B. Chefetz, Environ. Sci. Technol., 2016, 50, 4476–4482 CrossRef CAS PubMed.
- R. Zhang, P. Sun, T. H. Boyer, L. Zhao and C. H. Huang, Environ. Sci. Technol., 2015, 49, 3056–3066 CrossRef CAS PubMed.
- K. A. Landry, P. Sun, C. H. Huang and T. H. Boyer, Water Res., 2015, 68, 510–521 CrossRef CAS PubMed.
- M. Maurer, W. Pronk and T. A. Larsen, Water Res., 2006, 40, 3151–3166 CrossRef CAS PubMed.
- W. Pronk, M. Biebow and M. Boller, Environ. Sci. Technol., 2006, 40, 2414–2420 CrossRef CAS PubMed.
- W. Pronk, H. Palmquist, M. Biebow and M. Boller, Water Res., 2006, 40, 1405–1412 CrossRef CAS PubMed.
- W. Pronk, S. Zuleeg, J. Lienert, B. Escher, M. Koller, A. Berner, G. Koch and M. Boller, Water Sci. Technol., 2007, 56, 219–227 CrossRef CAS PubMed.
- C. J. Corwin and R. S. Summers, J. - Am. Water Works Assoc., 2012, 104, 43–44 CrossRef CAS.
-
J. Kearns, T. Anh, N. Reents, K. Shimabuku, R. Mahoney, R. Summers and D. Knapp, WEDC International Conference, 2014 Search PubMed.
- F. J. García-Mateos, R. Ruiz-Rosas, M. D. Marqués, L. M. Cotoruelo, J. Rodríguez-Mirasol and T. Cordero, Chem. Eng. J., 2015, 279, 18–30 CrossRef.
- C. Jung, J. Park, K. H. Lim, S. Park, J. Heo, N. Her, J. Oh, S. Yun and Y. Yoon, J. Hazard. Mater., 2013, 263(Pt 2), 702–710 CrossRef CAS PubMed.
- L. L. Ji, W. Chen, L. Duan and D. Q. Zhu, Environ. Sci. Technol., 2009, 43, 2322–2327 CrossRef CAS PubMed.
- M. Sparrevik, T. Saloranta, G. Cornelissen, E. Eek, A. M. Fet, G. D. Breedveld and I. Linkov, Environ. Sci. Technol., 2011, 45, 4235–4241 CrossRef CAS PubMed.
- A. Brennan, E. Moreno Jimenez, J. A. Alburquerque, C. W. Knapp and C. Switzer, Environ. Pollut., 2014, 193, 79–87 CrossRef CAS PubMed.
- S. Meyer, B. Glaser and P. Quicker, Environ. Sci. Technol., 2011, 45, 9473–9483 CrossRef CAS PubMed.
- R. Azargohar and A. K. Dalai, Appl. Biochem. Biotechnol., 2006, 131, 762–773 CrossRef CAS PubMed.
- M. Ahmad, A. U. Rajapaksha, J. E. Lim, M. Zhang, N. Bolan, D. Mohan, M. Vithanage, S. S. Lee and Y. S. Ok, Chemosphere, 2014, 99, 19–33 CrossRef CAS PubMed.
-
J. Lehmann and S. Joseph, Biochar for environmental management: science and technology, Routledge, 2012 Search PubMed.
- R. Chintala, J. Mollinedo, T. E. Schumacher, S. K. Papiernik, D. D. Malo, D. E. Clay, S. Kumar and D. W. Gulbrandson, Microporous Mesoporous Mater., 2013, 179, 250–257 CrossRef CAS.
- J. Ren, N. Li, L. Li, J. K. An, L. Zhao and N. Q. Ren, Bioresour. Technol., 2015, 178, 119–125 CrossRef CAS PubMed.
- A. Taghizadeh-Toosi, T. J. Clough, R. R. Sherlock and L. M. Condron, Plant Soil, 2012, 353, 73–84 CrossRef CAS.
- Y. Yao, B. Gao, M. Inyang, A. R. Zimmerman, X. Cao, P. Pullammanappallil and L. Yang, Bioresour. Technol., 2011, 102, 6273–6278 CrossRef CAS PubMed.
- M. Zhang, B. Gao, Y. Yao, Y. W. Xue and M. Inyang, Chem. Eng. J., 2012, 210, 26–32 CrossRef CAS.
- M. G. Pillai, P. Simha and A. Gugalia, J. Environ. Chem. Eng., 2014, 2, 46–55 CrossRef CAS.
-
L. Cui, J. Yan, L. Li, G. Quan, C. Ding, T. Chen, C. Yin, J. Gao and Q. Hussain, Does Biochar Alter the Speciation of Cd and Pb in Aqueous Solution?, 2014 Search PubMed.
- D. Mohan, A. Sarswat, Y. Sik Ok and C. U. Pittman, Bioresour. Technol., 2013, 191–202 Search PubMed.
- D. Mohan, R. Sharma, V. K. Singh, P. Steele and C. U. Pittman, Ind. Eng. Chem. Res., 2012, 51, 900–914 CrossRef CAS.
- D. Mohan, A. Sarswat, Y. S. Ok and C. U. Pittman Jr., Bioresour. Technol., 2014, 160, 191–202 CrossRef CAS PubMed.
- S. Ramola, T. Mishra, G. Rana and R. K. Srivastava, Environ. Monit. Assess., 2014, 186, 9023–9039 CrossRef CAS PubMed.
- X. Tan, Y. Liu, G. Zeng, X. Wang, X. Hu, Y. Gu and Z. Yang, Chemosphere, 2015, 125, 70–85 CrossRef CAS PubMed.
- Y. Yao, B. Gao, H. Chen, L. Jiang, M. Inyang, A. R. Zimmerman, X. Cao, L. Yang, Y. Xue and H. Li, J. Hazard. Mater., 2012, 209–210, 408–413 CrossRef CAS PubMed.
- M. Essandoh, B. Kunwar, C. U. Pittman, D. Mohan and T. Mlsna, Chem. Eng. J., 2015, 265, 219–227 CrossRef CAS.
- M. S. Kinch, A. Haynesworth, S. L. Kinch and D. Hoyer, Drug Discovery Today, 2014, 19, 1033–1039 CrossRef CAS PubMed.
- Title 22, 60320.200, California Department of Public Health, Regulations Related to Recycled Water, 2014 Search PubMed.
- J. Lienert, K. Gudel and B. I. Escher, Environ. Sci. Technol., 2007, 41, 4471–4478 CrossRef CAS PubMed.
- M. Ronteltap, M. Maurer and W. Gujer, Water Res., 2007, 41, 1859–1868 CrossRef CAS PubMed.
- K. M. Udert and M. Wachter, Water Res., 2012, 46, 453–464 CrossRef CAS PubMed.
- J. A. O'Neal and T. H. Boyer, Water Res., 2013, 47, 5003–5017 CrossRef PubMed.
- G. T. Seo, Y. Suzuki and S. Ohgaki, Desalination, 1996, 106, 39–45 CrossRef CAS.
- K. A. Landry and T. H. Boyer, Water Res., 2013, 47, 6432–6444 CrossRef CAS PubMed.
- M. M. Sena, Z. F. Chaudhry, C. H. Collins and R. J. Poppi, J. Pharm. Biomed. Anal., 2004, 36, 743–749 CrossRef CAS PubMed.
-
US Environmental Protection Agency, Methods for chemical analysis of water and wastes, EPA-600/4-79-020, Washington, DC, 1979, 430 pp. Search PubMed.
- K. M. Udert, T. A. Larsen, M. Biebow and W. Gujer, Water Res., 2003, 37, 2571–2582 CrossRef CAS PubMed.
- R. C. Pettersen, Adv. Chem. Ser., 1984, 207, 57–126 CrossRef CAS.
- B. Cagnon, X. Py, A. Guillot, F. Stoeckli and G. Chambat, Bioresour. Technol., 2009, 100, 292–298 CrossRef CAS PubMed.
- W. M. Daud and W. S. Ali, Bioresour. Technol., 2004, 93, 63–69 CrossRef CAS PubMed.
- C. I. Czimczik, C. M. Preston, M. W. I. Schmidt, R. A. Werner and E.-D. Schulze, Org. Geochem., 2002, 33, 1207–1223 CrossRef CAS.
- J. F. Li, Y. M. Li, Y. L. Wu and M. Y. Zheng, J. Hazard. Mater., 2014, 280, 450–457 CrossRef CAS PubMed.
-
T. K. Rout, Master's of Technology, National Institute of Technology, 2013 Search PubMed.
- ASTM D6851-02(2011) Standard Test Method for Determination of Contact pH with Activated Carbon, ASTM International, West Conshohocken, PA, 2011, DOI:10.1520/D6851-02R11.
- G. O. El-Sayed, M. M. Yehia and A. A. Asaad, Water Resources and Industry, 2014, 7–8, 66–75 CrossRef.
- Y. N. Sun, B. Gao, Y. Yao, J. N. Fang, M. Zhang, Y. M. Zhou, H. Chen and L. Y. Yang, Chem. Eng. J., 2014, 240, 574–578 CrossRef CAS.
- S. Wang, B. Gao, A. R. Zimmerman, Y. Li, L. Ma, W. G. Harris and K. W. Migliaccio, Chemosphere, 2015, 134, 257–262 CrossRef CAS PubMed.
- L. Ji, F. Liu, Z. Xu, S. Zheng and D. Zhu, Environ. Sci. Technol., 2010, 44, 3116–3122 CrossRef CAS PubMed.
- M. Keiluweit, P. S. Nico, M. G. Johnson and M. Kleber, Environ. Sci. Technol., 2010, 44, 1247–1253 CrossRef CAS PubMed.
- S. Kloss, F. Zehetner, A. Dellantonio, R. Hamid, F. Ottner, V. Liedtke, M. Schwanninger, M. H. Gerzabek and G. Soja, J. Environ. Qual., 2012, 41, 990–1000 CrossRef CAS PubMed.
- A. Mukherjee, A. R. Zimmerman and W. Harris, Geoderma, 2011, 163, 247–255 CrossRef CAS.
- T. M. Huggins, A. Haeger, J. C. Biffinger and Z. J. Ren, Water Res., 2016, 94, 225–232 CrossRef CAS PubMed.
-
W. Stumm and J. J. Morgan, Aquatic chemistry: chemical equilibria and rates in natural waters, Wiley, 1996 Search PubMed.
- Y. Q. Hu, T. Guo, X. S. Ye, Q. Li, M. Guo, H. N. Liu and Z. J. Wu, Chem. Eng. J., 2013, 228, 392–397 CrossRef CAS.
- C. Moreno-Castilla, Carbon, 2004, 42, 83–94 CrossRef CAS.
- P. Li and A. K. SenGupta, React. Funct. Polym., 2004, 60, 27–39 CrossRef CAS.
-
R. P. Schwarzenbach, P. M. Gschwend and D. M. Imboden, Environmental Organic Chemistry, John Wiley & Sons, 2005 Search PubMed.
- J. R. Domínguez, T. González, P. Palo and E. M. Cuerda-Correa, Desalination, 2011, 269, 231–238 CrossRef.
- C. T. Jafvert, J. C. Westall, E. Grieder and R. P. Schwarzenbach, Environ. Sci. Technol., 1990, 24, 1795–1803 CrossRef CAS.
-
ChemSpider, ACD Percepta Labs Search PubMed.
-
US EPA, Estimation Programs Interface Suite™ for Microsoft® Windows, v 4.11, United States Environmental Protection Agency, Washington, DC, USA, 2014 Search PubMed.
-
ChemAxon, Chemicalize Search PubMed.
- A. Sendrowski and T. H. Boyer, Desalination, 2013, 322, 104–112 CrossRef CAS.
- M. Ronteltap, M. Maurer and W. Gujer, Water Res., 2007, 41, 977–984 CrossRef CAS PubMed.
Footnote |
† Electronic supplementary information (ESI) available. See DOI: 10.1039/c6ew00224b |
|
This journal is © The Royal Society of Chemistry 2017 |
Click here to see how this site uses Cookies. View our privacy policy here.