Osmium-nitrido corroles as NIR indicators for oxygen sensors and triplet sensitizers for organic upconversion and singlet oxygen generation†
Received
17th March 2016
, Accepted 25th May 2016
First published on 26th May 2016
Abstract
The photophysical properties of nitridoosmium(VI) corroles have been investigated. The complexes exhibit room temperature NIR phosphorescence (λmax 779–795 nm). Long decay times (110–150 μs in solution and 136–183 μs in polystyrene) are responsible for efficient quenching of the emission by oxygen. All the complexes act as efficient sensitizers of singlet oxygen (quantum yields of 1O2 0.76–0.95). Optical oxygen sensors prepared on the basis of nitridoosmium(VI) corroles exhibit high photostability and excellent sensitivity in the physiologically relevant range. The complexes have also been found to be promising sensitizers in triplet–triplet annihilation-based upconversion systems. Finally and not least, simple synthetic accessibility makes these dyes particularly attractive for application as optical materials.
Introduction
Dyes exhibiting room temperature phosphorescence find numerous applications, for example, as emitters in OLEDs,1,2 as probes for sensing3 and imaging,4–6 and as sensitizers for triplet–triplet annihilation-based upconversion.7–10 NIR phosphorescent dyes are of particular interest11 and are used in NIR OLEDs,12–14 and solar concentrators.15 The combination of NIR emission with efficient absorption in the red part of the spectrum not only enables in vivo sensing and imaging of oxygen16–19 and glucose,20 but also makes the dyes promising for application in photon upconversion systems where harvesting of red light is of primary importance. Extended porphyrins, such as tetrabenzoporphyrins21,22 and tetranaphthoporphyrins,23,24 are probably the most common representatives of the dyes showing very strong NIR phosphorescence (phosphorescence quantum yields Φ ∼ 0.1–0.6). Several new classes of NIR phosphorescent dyes such as Pt(II) and Pd(II) complexes with donor–acceptor Schiff bases25 and Pt(II) hybrid complexes with BODIPY dyes26 exhibit moderate phosphorescence quantum yields (1–11%). Other Pt(II) complexes with BODIPY dyes and naphthalene diimide chromophores coordinated via alkynyl linkages, however, exhibited even lower Φ that were well below 1%.27–30 Nevertheless, even in the absence of phosphorescent properties, dyes with high efficiency of inter-system crossing are very interesting as triplet sensitizers in photon upconversion and as singlet oxygen generators in photodynamic therapy.31,32
As porphyrin analogues with a rapidly unfolding chemistry, corroles are of considerable interest in this regard. A wide range of metallocorroles33–36 have been synthesized in recent years and several have witnessed applications as catalysts,37,38 in chemical sensing,39 and in the biomedical arena.40 Many of them exhibit bright fluorescence;41,42 only a few, however, have been shown to possess NIR phosphorescence at room temperature. The latter class of complexes includes iridium corroles,43,44 a gold(III) β-octabromocorrole,45 and aluminium and gallium complexes of β-iodinated corroles.46 A variety of other 5d metallocorroles, including rhenium,47 osmium,48 gold,49–51 and platinum52 corroles, which might be expected to exhibit NIR phosphorescence, have been synthesized in recent years. Given the steric mismatch of a large 5d metal and the constricted N4 cavity of a corrole, many of the syntheses are capricious and low-yielding. The nitridoosmium(VI) corroles, however, are accessible simply and in good yields, prompting us to examine their photophysical properties. Although they exhibit only moderate NIR phosphorescence, the OsN corroles are notable for their exceptional chemical and photochemical stability and exhibit considerable promise as oxygen sensors, triplet sensitizers in upconversion systems, and as singlet oxygen photosensitizers.
Experimental
Materials
The osmium-nitrido corrole complexes were prepared according to a recently reported procedure.48 The annihilators for upconversion experiments, perylene and solvent green 5 (=SG 5, diisobutyl 3,9-perylenedicarboxylate) were obtained from Fluka and ABCR (Karlsruhe, Germany), respectively. 9,10-Dimethylanthracene and methylene blue were acquired from TCI Europe and Sigma-Aldrich, respectively.
Preparation of oxygen sensors
The dye (1 mg), polystyrene (Acros Organics, MW 250
000, 100 mg) and lipophilic titanium dioxide nanoparticles (P170, Kemira, 50 mg) were dissolved/dispersed in chloroform (1.35 g). The “cocktail” obtained was knife-coated onto a polyethylene terephthalate support (Melinex 505, Pütz, Taunusstein, Germany), yielding ∼7 μm thin films after evaporation of the solvent. For acquisition of absorption and emission spectra, a “cocktail” was prepared analogously but with no added titanium dioxide.
Measurements
Absorption spectra were acquired on a Cary 50 UV-Vis spectrophotometer (Varian). Emission spectra were measured on a Fluorolog 3 fluorescence spectrometer from Horiba (Japan) equipped with a NIR-sensitive photomultiplier R2658 from Hamamatsu (Japan), all spectra being corrected for the sensitivity of the photomultiplier. The luminescence quantum yields in solution were measured relative to platinum(II) tetraphenyltetrabenzoporphyrin (PtTPTBP, QY 51%).53 The solutions were placed in sealable quartz cells (Hellma Analytics, Mülheim, Germany) and deoxygenated by bubbling argon (5.0, Linde gas, Austria) for 15 min. Absolute quantum yields for the sensor foils were acquired in an integrating sphere from Horiba.
The photostability of the dyes was investigated by irradiating air-saturated dye solutions in toluene (1.4 × 10−5 M) with the light of a high power orange LED array (λmax 590 nm, http://www.led-tech.de) operated at 27 V and 0.6 A. The light was additionally focused through a lens (Edmunds Optics) to result in a photon flux density of ∼10
800 μmol s−1 m−2 or 0.22 W cm−2 as determined with a Li-250A light meter from Li-COR (http://www.licor.com). The cuvette with the solution was shaken after each irradiation period before absorbance measurement.
Luminescence decay times in deoxygenated toluene were measured in the frequency domain using a lock-in amplifier from PreSens (Regensburg, Germany). The solutions were excited with light from a 590 nm LED filtered through a NIR-blocking filter Calflex X (transmittance of 50% at 730 nm; Linos) and the emission was detected with a photodiode equipped with a long-pass RG 780 filter (transmittance of 50% at 780 nm; Schott). A modulation frequency of 916 Hz was used.
The oxygen sensors were characterized in the frequency domain with a two-phase lock-in amplifier (SR830, Stanford Research, Inc., USA). The excitation light was provided by a 455 nm LED (Roithner Lasertechnik, Austria). The light was sinusoidally modulated at 1150 Hz and filtered through a BG-12 band-pass filter (transmission range 300–520 nm; Schott). The emission light (filtered through an RG 780 filter) was recorded by a photomultiplier tube (H5701-02, Hamamatsu, Japan). Gas calibration mixtures were obtained from nitrogen and compressed air using a gas mixing device from MKS (Andover, MA, USA). The temperature was controlled with a ThermoHaake DC50 cryostat (Thermo Fisher Scientific, Inc).
Triplet–triplet annihilation upconversion was studied on Fluorolog 3 fluorescence spectrometer. The anoxic toluene solutions containing 1 × 10−4 M of an OsVIN corrole and 2.5 × 10−4 M of the annihilator were excited at 595 nm (14 nm slits). The intensity dependency of the upconversion emission was acquired with neutral density filters from Schott (NG 11, NG 4, NG 3 and NG 9) with transmission at 595 nm of 77.8%, 34.3%, 9.4% and 4.4%, respectively.
Singlet oxygen quantum yields were determined by a relative method using 9,10-dimethylanthracene (DMA) as singlet oxygen acceptor.54 2.5 mL of solution of DMA (0.28 mM) and a sensitizer (∼15 μM, adjusted for identical absorption of the corroles and the reference dye at the excitation wavelength) in EtOH
:
THF (9
:
1 v/v) were placed in a 1 cm screw-cap quartz cuvette which was irradiated with the light of a xenon lamp using Fluorolog 3 spectrometer (λmax 595 nm, 10 nm slit, photon flux ∼175 μmol s−1 m−2). The solution was stirred during irradiation and the cuvette was shaken before acquisition of the absorption spectra. The curve was constructed as an average of 3 independent bleaching experiments. Quantum yields of singlet oxygen production ΦΔ were determined from the slope of the curve (absorbance at 358 nm vs. time) using methylene blue as a standard (ΦΔ of 0.48 which is the average value from the data reported by Gross et al.54 and Usui et al.55).
Results and discussion
Photophysical properties of OsVIN corroles
The OsVIN corroles studied (see structures depicted in Fig. 1) exhibit strong absorption in the blue and orange parts of the electromagnetic spectrum and room-temperature phosphorescence in the NIR region (Fig. 1 and Table 1). Observe that the spectra are slightly susceptible to substituent effects. Thus, both the Q band maxima and the emission maxima slightly shift bathochromically with increasing electron-donating character of the para-substituent, i.e., Os[TpOCH3PC](N) > Os[TPC](N) > Os[TpCF3PC](N). Importantly for practical applications, the dyes can be excited with bright 590 nm and 605 nm LEDs.
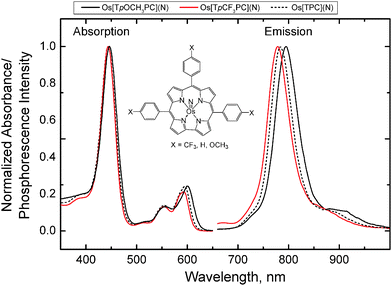 |
| Fig. 1 Absorption and emission spectra of OsVIN corroles in toluene (25 °C, λex 595 nm). | |
Table 1 Photophysical properties of OsVIN corroles in anoxic toluene solutions
Complex |
λ
max(abs), nm |
ε × 10−4, M−1 cm−1 |
λ
max(em), nm |
Φ, % |
τ, 25 °C, μs |
Os[TpOCH3PC](N) |
447, 558, 601 |
8.31, 1.18, 2.14 |
795 |
0.9 |
110 |
Os[TPC](N) |
444, 555, 595 |
9.32, 1.28, 2.27 |
784 |
1.3 |
128 |
Os[TpCF3PC](N) |
444, 554, 593 |
8.02, 1.07, 1.73 |
779 |
1.3 |
150 |
The phosphorescence decay times for all the complexes are rather long (>100 μs) and are intermediate between those of Pt(II) (τ ∼ 50–70 μs) and Pd(II) porphyrins (τ ∼ 300–1000 μs). The shortest phosphorescence lifetime is exhibited by Os[TpOCH3PC](N) and the longest by Os[TpCF3PC](N). This correlates well with the spectral properties of the dyes and the phosphorescence quantum yields. The quantum yields in solution are moderate, but nevertheless considerably higher than for the majority of phosphorescent iridium(III) corroles reported in the literature (<0.01%,43 0.033–1.2%44).
The photostability of dyes, a key parameter for all applications, was investigated via irradiation of 1.4 × 10−5 M solutions of the complexes in air-saturated toluene with the light of a high power orange LED array (photon flux density 10
800 μmol s−1 m−2) whose emission wavelength (λmax 590 nm) almost perfectly matched the Q-bands of the dyes. No visible photodegradation was observed for Os[TpCF3PC](N) (Fig. S1, ESI†) and Os[TPC](N) after 30 min of continuous irradiation, indicating excellent photostability of these two dyes. Os[TpOCH3PC](N) appeared to be slightly less photostable and ∼1.1% dye degradation could be detected under the same conditions (Fig. S2, ESI†), whereas after 6 h of irradiation bleaching of ∼4.9% of the dye was observed. The lower photostability of Os[TpOCH3PC](N) is presumably due to the electron-donating character of the methoxy groups in the para-position of the meso-phenyl substituents, which may favour oxidation of the dye by singlet oxygen.
Oxygen sensing properties of the OsVIN corroles
The phosphorescence of the corroles is almost completely quenched by molecular oxygen in solutions of organic solvents, which makes them potentially suitable for application as oxygen-sensing materials. Accordingly, oxygen sensors were prepared by immobilizing the corroles into polystyrene (1 wt% of the indicator) along with TiO2 nanoparticles used as a light-scattering material to increase brightness. Although many other polymers could be used, polystyrene was chosen for its high chemical stability, good compatibility with the indicator dyes, and for simple comparisons with state-of-the-art materials. The resulting planar sensors exhibit adequate brightness under excitation with a 590 nm orange LED. The positions of the absorption bands are not affected by immobilization and the phosphorescence spectra are also very similar to those obtained in the toluene solution (Fig. S3, ESI† and Table 2). The phosphorescent decay times are slightly higher in polystyrene relative to the solutions (Tables 1 and 2), reflecting less efficient radiationless deactivation in a rigid matrix. The Φ values are approximately 1% and are comparable to the values obtained in solution.
Table 2 Photophysical and sensing properties of the oxygen sensors based on OsVIN corroles
Complex |
λ
max(abs), nm |
λ
max(em), nm |
Φ,a % |
τ
0 at 25 °C, μs |
K
1SV at 25 °C, kPa−1 b |
K
SV at 25 °C, kPa−1 c |
k
q at 25 °C, Pa−1 cm−1 c |
dτ0/dT at 25 °C, % K−1 |
Anoxic conditions.
Decay time Stern–Volmer plots; fit parameters: m = 0.08; f = 0.68 for all optodes.
Intensity Stern–Volmer plots.
|
Os[TpOCH3PC](N) |
447, 557, 601 |
787 |
0.72 |
136 |
0.91 |
0.40 |
2.9 |
−0.203 |
Os[TPC](N) |
444, 554, 596 |
779 |
0.85 |
158 |
1.03 |
0.45 |
2.9 |
−0.178 |
Os[TpCF3PC](N) |
444, 553, 593 |
771 |
0.95 |
183 |
1.38 |
0.56 |
3.0 |
−0.136 |
Fig. 2 shows a representative calibration plot obtained with phase fluorometry. Whereas the Stern–Volmer plots for fluorescence intensity are almost linear (correlation coefficient r2 0.998), the decay time plots deviate significantly from linearity. Such behaviour is in fact typical for oxygen sensors and very few matrices exhibit near-linear decay time plots. The nonlinear plots can be described with an equation derived from a so-called “two-site model” that assumes localization of the dye in two different environments:56
|  | (1) |
where
f is the fraction of the total emission for the first micro-environment, and
K1SV and
K2SV are the Stern–Volmer constants for each component.
K2SV =
K1SV ×
m.
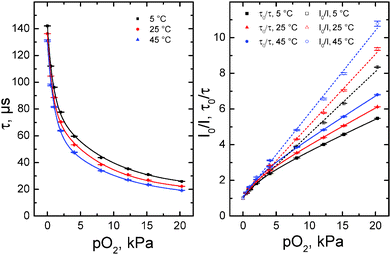 |
| Fig. 2 Decay time (left) and Stern–Volmer plots (right) for the oxygen sensor based on Os[TpOCH3PC](N) in polystyrene. Eqn (1) was used to fit the decay time Stern–Volmer plots, whereas a linear fit was used in case of the intensity plots. | |
Although the two-site model is physically meaningful only for the luminescence intensity, the decay time plots can also be almost perfectly fit with the above equation (r2 0.9999). The Stern–Volmer constants obtained from the fit are summarized in Table 2. Evidently, the sensors are promising for oxygen sensing from hypoxia to air saturation. Consistent with differences in luminescence decay times, the sensitivity is the highest for Os[TpCF3PC](N) and the lowest for Os[TpOCH3PC](N)-based sensors. The sensitivity of the sensor can thus be tailored to a specific application simply by varying the nature of the complex used. Obviously, much more substantial modulation of the sensitivity should be possible by substitution of polystyrene with a polymer with higher/lower oxygen permeability. Table 2 also shows that the bimolecular quenching constant kq = KSV/τ0 is very similar for all the complexes examined.
Fig. 2 evinces temperature cross-talk of the oxygen sensor, a well-known phenomenon that originates from two main factors: (i) thermal quenching of phosphorescence, which is the main error source at low pO2 close to the limit of detection, and (ii) temperature-dependent diffusion and solubility of oxygen in the matrix polymer. Thermal quenching of the OsVIN complexes is relatively small as indicated by the temperature coefficients of τ0 in the range from 0.13 to 0.20% K−1 (Table 2). Notably, popular oxygen indicators such as Pd(II) porphyrins and particularly Ru(II) polypyridyl complexes show significantly higher temperature sensitivity of τ0 (dτ0/dT ∼ 0.33% K−1 (ref. 57) and ∼0.5% K−1,58 respectively). Interestingly, thermal quenching is less efficient in case of Os[TpCF3PC](N) and is the highest for Os[TpOCH3PC](N). This correlates well with the phosphorescence quantum yields obtained at room temperature. As with other oxygen indicators in polystyrene, quenching becomes more efficient at higher temperatures (Fig. 2 and Table S1, ESI†). Despite that the Stern–Volmer constants KSV are different for the three corroles, the bimolecular quenching constants kq are very similar (Table S1, ESI†).
The stability and reversibility of the sensors was investigated by continuous irradiation with a 455 nm LED (Fig. 3), which also reflects their photostability. Since the photostability of the dyes may vary significantly for anoxic conditions and air saturation (due to generation of highly reactive singlet oxygen), we tested it under both conditions. Fig. 3 shows that stability of the optode based on the Os[TpOCH3PC](N) complex is excellent. In fact, the intensity decreases by only 4% after the first 2.5 h of irradiation, and by only 5% after 6.5 h, indicating that bleaching is even slower after initial period. Also, no changes in the luminescence decay time were observed during the duration of the experiment. It may be noted that 1 h of continuous irradiation corresponds to about 200
000 measurement points under standard conditions (integration time 20 ms), emphasizing the suitability of the materials for prolonged measurements as well as for measurements at high light intensity (e.g., in microscopy). Analogously to the optode based on Os[TpOCH3PC](N), the sensors based on the other OsVIN-corroles also showed excellent stability.
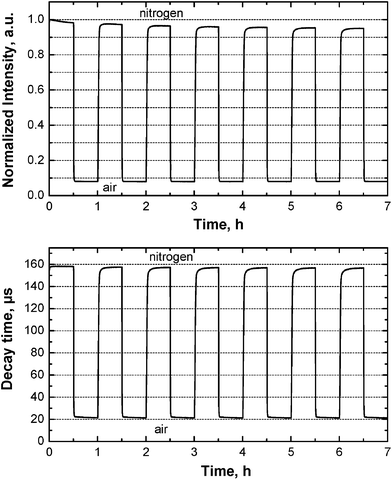 |
| Fig. 3 Intensity (above) and decay time (below) profiles for the oxygen sensor based on Os[TpOCH3PC](N) in polystyrene upon continuous excitation with a 455 nm LED (25 °C). | |
OsVIN corroles as sensitizers in triplet–triplet annihilation-based upconversion
The room-temperature phosphorescence of the OsVIN corroles and the relatively long phosphorescence decay times suggested that these dyes are potentially suitable as sensitizers in triplet–triplet-annihilation-based upconversion systems. Their absorption in the orange part of the spectrum is particularly attractive, since the number of sensitizers available for this part of the spectrum is still limited. Anoxic toluene solutions containing 1 × 10−4 M of an OsVIN corrole as a sensitizer (S) and 2.5 × 10−4 M of an annihilator (A, perylene or SG 5) indeed exhibit intense upconverted emission from the annihilator, which is clearly visible even under excitation with a 590 nm LED (Fig. 4). Under excitation with orange light (λex 595 nm), the following processes occur:
S*(T1) + A(S0) → S(S0) + A*(T1) |
A*(T1) + A*(T1) → A*(S1) + A(S0) |
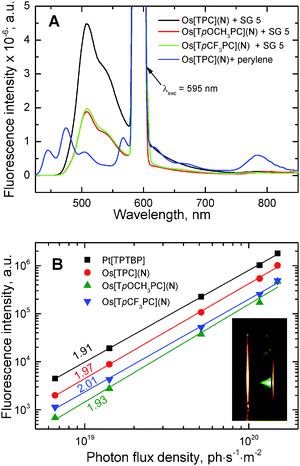 |
| Fig. 4 Panel A: Emission spectra of anoxic toluene solutions containing 1 × 10−4 M of an OsVIN corrole and 2.5 × 10−4 M of annihilator (λexc 595 nm). Panel B: Dependence of the intensity of upconverted fluorescence (λ = 505 nm) on the power density of the excitation light with slopes of the curve indicated. The inset shows a photographic image of anoxic toluene solution containing 1 × 10−4 M of OsVI[TPC](N) and 2.5 × 10−4 M of annihilator SG 5 under excitation with a 590 nm LED. | |
Among the three corroles investigated, Os[TPC](N) exhibits the brightest upconverted emission (Fig. 5A). The quantum yields for the upconverted fluorescence were estimated to be 2.0, 0.9, and 0.8% for Os[TPC](N), Os[TpCF3PC](N) and Os[TpOCH3PC](N), respectively, when using SG 5 as the annihilator, and 0.51, 0.11 and 0.15%, with perylene as annihilator. The significantly lower upconversion quantum yields for perylene relative to SG 5 may be attributed to less efficient triplet–triplet energy transfer from the sensitizer to the annihilator as a result of the relatively high energy of the triplet state of perylene. Indeed, residual phosphorescence from the sensitizer is visible when perylene is used as annihilator whereas it vanishes completely in case of SG 5 (Fig. 4A).
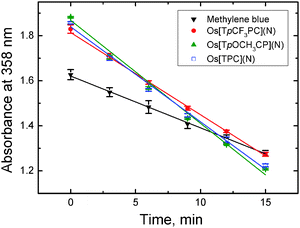 |
| Fig. 5 Degradation profiles of 9,10-dimethylanthracene (0.28 mM) in an air-saturated solution (9 : 1 EtOH/THF) in presence of a sensitizer (∼15 μM) upon excitation with orange light (595 ± 5 nm; photon flux ∼175 μmol s−1 m−2). Absorption spectra for all the systems are provided in ESI† (Fig. S4–S7). | |
For comparison, the upconverted fluorescence quantum yield for the commonly used sensitizer Pt[TPTBP] were estimated to be 3.6 and 1.8%, when using SG 5 and perylene as annihilators, respectively. It should be noted that all the quantum yields are expected to be significantly higher at higher intensities of the excitation light, which were unfortunately not attainable with our experimental set-up. In fact, all the experimental points exhibit a quadratic dependency of the emission intensity on the intensity of the excitation light well below the saturation limit as indicated by the slope of the double logarithmic plots59 close to 2.0 (Fig. 4B).
OsVIN corroles as singlet oxygen sensitizers
Singlet oxygen sensitizers are of high interest mostly due to their application in photodynamic therapy of cancer60 but also as singlet oxygen generators for photocatalytic applications61,62 and for disinfection purposes.63 OsVIN corroles might be attractive for these applications due to long-wavelength absorption and high stability. Fig. 5 illustrates the determination of singlet oxygen quantum yields ΦΔ with a chemical method using 9,10-dimethylanthracene as a singlet oxygen acceptor.54 Methylene blue (ΦΔ = 0.48, an average value from the data reported by Gross et al.54 and Usui et al.55) was used as the reference dye. All three complexes were found to be efficient generators of singlet oxygen, with ΦΔ's of 0.95, 0.88 and 0.76 for Os[TpOCH3PC](N), Os[TPC](N) and Os[TpCF3PC](N), respectively. Interestingly, this trend is opposite to that observed for the phosphorescence quantum yields and decay times (Table 1). We may conclude that osmium-nitrido corroles show comparable or higher efficiency of 1O2 sensitization than the metal-free corroles and the respective Ga(III) complexes (ΦΔ 0.34–0.86;64 0.51–0.7765) and are much more powerful 1O2 generators than Ir(III) corroles (ΦΔ 0.09–0.15).43
Conclusions
Osmium(VI)-nitrido corroles represent a new class of NIR phosphorescent materials with relatively long decay times and high photostability. Although the luminescence quantum yields are moderate, they are significantly higher than those of the majority of phosphorescent corroles reported up to date. Furthermore, we have shown that OsVIN corroles can be used as indicators for optical oxygen sensors, as highly efficient singlet oxygen sensitizers, and as triplet sensitizers in organic upconversion systems, where high photostability of the dyes is of primary importance.
Acknowledgements
This project was partly supported by a FRINATEK project no. 231086 of the Research Council of Norway (AG). The authors thank Matthias Schwar (Graz University of Technology) for technical support.
References
- R. C. Evans, P. Douglas and C. J. Winscom, Coord. Chem. Rev., 2006, 250, 2093–2126 CrossRef CAS.
- J. Kalinowski, V. J. Kalinowski, V. Fattori, M. Cocchi and J. A. G. Williams, Coord. Chem. Rev., 2011, 255, 2401–2425 CrossRef CAS.
- Q. Zhao, F. Li and C. Huang, Chem. Soc. Rev., 2010, 39, 3007–3030 RSC.
- M. Schäferling, Angew. Chem., Int. Ed., 2012, 51, 3532–3554 CrossRef PubMed.
- Q. Zhao, C. Huang and F. Li, Chem. Soc. Rev., 2011, 40, 2508–2524 RSC.
- D. B. Papkovsky and T. C. O'Riordan, J. Fluoresc., 2005, 15, 569–584 CrossRef CAS PubMed.
- T. N. Singh-Rachford and F. N. Castellano, Coord. Chem. Rev., 2010, 254, 2560–2573 CrossRef CAS.
- J. Zhao, S. Ji and H. Guo, RSC Adv., 2011, 1, 937–950 RSC.
- J. Zhou, Q. Liu, W. Feng, Y. Sun and F. Li, Chem. Rev., 2015, 115, 395–465 CrossRef CAS PubMed.
- J. Zhao, W. Wu, J. Sun and S. Guo, Chem. Soc. Rev., 2013, 42, 5323–5351 RSC.
- H. Xiang, J. Cheng, X. Ma, X. Zhou and J. J. Chruma, Chem. Soc. Rev., 2013, 42, 6128–6185 RSC.
- C. Borek, K. Hanson, P. I. Djurovich, M. E. Thompson, K. Aznavour, R. Bau, Y. Sun, S. R. Forrest, J. Brooks, L. Michalski and J. Brown, Angew. Chem., Int. Ed., 2007, 46, 1109–1112 CrossRef CAS PubMed.
- J. R. Sommer, R. T. Farley, K. R. Graham, Y. Yang, J. R. Reynolds, J. Xue and K. S. Schanze, ACS Appl. Mater. Interfaces, 2009, 1, 274–278 CAS.
- K. R. Graham, Y. Yang, J. R. Sommer, A. H. Shelton, K. S. Schanze, J. Xue and J. R. Reynolds, Chem. Mater., 2011, 23, 5305–5312 CrossRef CAS.
- M. J. Currie, J. K. Mapel, T. D. Heidel, S. Goffri and M. A. Baldo, Science, 2008, 321, 226–228 CrossRef CAS PubMed.
- E. Roussakis, Z. Li, A. J. Nichols and C. L. Evans, Angew. Chem., Int. Ed., 2015, 54, 8340–8362 CrossRef CAS PubMed.
- R. I. Dmitriev and D. B. Papkovsky, Cell. Mol. Life Sci., 2012, 69, 2025–2039 CrossRef CAS PubMed.
- C. Staudinger and S. M. Borisov, Methods Appl. Fluoresc., 2015, 3, 042005 CrossRef PubMed.
- A. Y. Lebedev, A. V. Cheprakov, S. Sakadžić, D. A. Boas, D. F. Wilson and S. A. Vinogradov, ACS Appl. Mater. Interfaces, 2009, 1, 1292–1304 CAS.
- B. Nacht, C. Larndorfer, S. Sax, S. M. Borisov, M. Hajnsek, F. Sinner, E. J. W. List-Kratochvil and I. Klimant, Biosens. Bioelectron., 2015, 64, 102–110 CrossRef CAS PubMed.
- S. A. Vinogradov and D. F. Wilson, J. Chem. Soc., Perkin Trans. 2, 1995, 103–111 RSC.
- O. S. Finikova, A. V. Cheprakov, I. P. Beletskaya, P. J. Carroll and S. A. Vinogradov, J. Org. Chem., 2004, 69, 522–535 CrossRef CAS PubMed.
- V. V. Rozhkov, M. Khajehpour and S. A. Vinogradov, Inorg. Chem., 2003, 42, 4253–4255 CrossRef CAS PubMed.
- O. S. Finikova, A. V. Cheprakov, P. J. Carroll and S. A. Vinogradov, J. Org. Chem., 2003, 68, 7517–7520 CrossRef CAS PubMed.
- S. M. Borisov, R. Saf, R. Fischer and I. Klimant, Inorg. Chem., 2013, 52, 1206–1216 CrossRef CAS PubMed.
- W. Wu, J. Zhao, H. Guo, J. Sun, S. Ji and Z. Wang, Chem. – Eur. J., 2012, 18, 1961–1968 CrossRef CAS PubMed.
- W. Wu, L. Liu, X. Cui, C. Zhang and J. Zhao, Dalton Trans., 2013, 42, 14374–14379 RSC.
- F. Zhong, A. Karatay, L. Zhao, J. Zhao, C. He, C. Zhang, H. G. Yaglioglu, A. Elmali, B. Küçüköz and M. Hayvali, Inorg. Chem., 2015, 54, 7803–7817 CrossRef CAS PubMed.
- Y. Liu, W. Wu, J. Zhao, X. Zhang and H. Guo, Dalton Trans., 2011, 40, 9085–9089 RSC.
- L. Liu, S. Guo, J. Ma, K. Xu, J. Zhao and T. Zhang, Chem. – Eur. J., 2014, 20, 14282–14295 CrossRef CAS PubMed.
- A. Kamkaew, S. H. Lim, H. B. Lee, L. V. Kiew, L. Y. Chung and K. Burgess, Chem. Soc. Rev., 2013, 42, 77–88 RSC.
- J. Zhao, K. Xu, W. Yang, Z. Wang and F. Zhong, Chem. Soc. Rev., 2015, 44, 8904–8939 RSC.
- I. Aviv and Z. Gross, Chem. Commun., 2007, 1987–1999 RSC.
- K. E. Thomas, A. B. Alemayehu, J. Conradie, C. M. Beavers and A. Ghosh, Acc. Chem. Res., 2012, 45, 1203–1214 CrossRef CAS PubMed.
- H. L. Buckley and J. Arnold, Dalton Trans., 2015, 44, 30–36 RSC.
-
J. H. Palmer, in Molecular Electronic Structures of Transition Metal Complexes I, ed. D. M. P. Mingos, P. Day and J. P. Dahl, Springer, Berlin Heidelberg, 2011, pp. 49–89 Search PubMed.
- L. Simkhovich and Z. Gross, Tetrahedron Lett., 2001, 42, 8089–8092 CrossRef CAS.
- I. Aviv-Harel and Z. Gross, Chem. – Eur. J., 2009, 15, 8382–8394 CrossRef CAS PubMed.
- L. Tortora, G. Pomarico, S. Nardis, E. Martinelli, A. Catini, A. D'Amico, C. Di Natale and R. Paolesse, Sens. Actuators, B, 2013, 187, 72–77 CrossRef CAS.
- I. Aviv-Harel and Z. Gross, Coord. Chem. Rev., 2011, 255, 717–736 CrossRef CAS.
- A. Ghosh and M. Ravikanth, Chem. – Eur. J., 2012, 18, 6386–6396 CrossRef CAS PubMed.
- J. J. Weaver, K. Sorasaenee, M. Sheikh, R. Goldschmidt, E. Tkachenko, Z. Gross and H. B. Gray, J. Porphyrins Phthalocyanines, 2004, 08, 76–81 CrossRef CAS.
- W. Sinha, L. Ravotto, P. Ceroni and S. Kar, Dalton Trans., 2015, 44, 17767–17773 RSC.
- J. H. Palmer, A. C. Durrell, Z. Gross, J. R. Winkler and H. B. Gray, J. Am. Chem. Soc., 2010, 132, 9230–9231 CrossRef CAS PubMed.
- E. Rabinovich, I. Goldberg and Z. Gross, Chem. – Eur. J., 2011, 17, 12294–12301 CrossRef CAS PubMed.
- J. Vestfrid, I. Goldberg and Z. Gross, Inorg. Chem., 2014, 53, 10536–10542 CrossRef CAS PubMed.
- R. F. Einrem, K. J. Gagnon, A. B. Alemayehu and A. Ghosh, Chem. – Eur. J., 2016, 22, 517–520 CrossRef CAS PubMed.
- A. B. Alemayehu, K. J. Gagnon, J. Terner and A. Ghosh, Angew. Chem., Int. Ed., 2014, 53, 14411–14414 CrossRef CAS PubMed.
- A. B. Alemayehu and A. Ghosh, J. Porphyrins Phthalocyanines, 2011, 15, 106–110 CrossRef CAS.
- K. E. Thomas, A. B. Alemayehu, J. Conradie, C. Beavers and A. Ghosh, Inorg. Chem., 2011, 50, 12844–12851 CrossRef CAS PubMed.
- K. E. Thomas, C. M. Beavers and A. Ghosh, Mol. Phys., 2012, 110, 2439–2444 CrossRef CAS.
- A. B. Alemayehu, H. Vazquez-Lima, C. M. Beavers, K. J. Gagnon, J. Bendix and A. Ghosh, Chem. Commun., 2014, 50, 11093–11096 RSC.
- S. M. Borisov, G. Nuss, W. Haas, R. Saf, M. Schmuck and I. Klimant, J. Photochem. Photobiol., A, 2009, 201, 128–135 CrossRef CAS.
- E. Gross, B. Ehrenberg and F. M. Johnson, Photochem. Photobiol., 1993, 57, 808–813 CrossRef CAS PubMed.
- Y. Usui, H. Koike and Y. Kurimura, Bull. Chem. Soc. Jpn., 1987, 60, 3373–3378 CrossRef CAS.
- E. R. Carraway, J. N. Demas, B. A. DeGraff and J. R. Bacon, Anal. Chem., 1991, 63, 337–342 CrossRef CAS.
- S. M. Borisov, G. Nuss and I. Klimant, Anal. Chem., 2008, 80, 9435–9442 CrossRef CAS PubMed.
- S. M. Borisov and I. Klimant, Anal. Chem., 2007, 79, 7501–7509 CrossRef CAS PubMed.
- A. Monguzzi, J. Mezyk, F. Scotognella, R. Tubino and F. Meinardi, Phys. Rev. B: Condens. Matter Mater. Phys., 2008, 78, 195112 CrossRef.
- D. E. J. G. J. Dolmans, D. Fukumura and R. K. Jain, Nat. Rev. Cancer, 2003, 3, 380–387 CrossRef CAS PubMed.
- S. Hecht and J. M. J. Fréchet, J. Am. Chem. Soc., 2001, 123, 6959–6960 CrossRef CAS.
- J. Sun, J. Zhao, H. Guo and W. Wu, Chem. Commun., 2012, 48, 4169–4171 RSC.
- L. Villén, F. Manjón, D. García-Fresnadillo and G. Orellana, Appl. Catal., B, 2006, 69, 1–9 CrossRef.
- W. Shao, H. Wang, S. He, L. Shi, K. Peng, Y. Lin, L. Zhang, L. Ji and H. Liu, J. Phys. Chem. B, 2012, 116, 14228–14234 CrossRef CAS PubMed.
- B. Ventura, A. Degli Esposti, B. Koszarna, D. T. Gryko and L. Flamigni, New J. Chem., 2005, 29, 1559–1566 RSC.
Footnote |
† Electronic supplementary information (ESI) available. See DOI: 10.1039/c6tc01126h |
|
This journal is © The Royal Society of Chemistry 2016 |
Click here to see how this site uses Cookies. View our privacy policy here.