Preferential formation of columnar mesophases via peripheral modification of discotic π-systems with immiscible side chain pairs†
Received
4th January 2016
, Accepted 18th January 2016
First published on 19th January 2016
Abstract
When sufficient volume of dodecyl chains are attached at one imide position of a perylenediimide (PDI) or naphthalenediimide (NDI) core and triethyleneglycol (TEG) chains on the other side, the resulting molecules PDIC12/TEG
G0, PDIC12/TEG
G1, and NDIC12/TEG
G0 self-assemble into a rectangular columnar mesophase with p2mg symmetry, forming hydrophobic/hydrophilic nano-segregation of side chains. The driving force of PDIC12/TEG
G0 to form preferentially the rectangular columnar mesophase is given by the immiscibility between the side chain pairs—exclusion of other phases such as cubic, crystalline and amorphous phases, where thermodynamically unstable contacts between hydrophobic and hydrophilic chains considerably take place. In contrast, this preference is less found in the analogous molecules decorated with either dodecyl or TEG chains at both termini. PDIC12/C12
G0 and PDITEG/TEG
G0 form a hexagonal columnar mesophase because of the optimized chain/core volume. However, if the side chain volume grows, PDITEG/TEG
G1 does not form a mesophase but undergoes a soft crystalline–isotropic phase transition, while PDIC12/C12
G1 was revealed to destabilize its columnar mesophase but forms a micellar cubic phase. NDIC12/C12
G0 resulted in a strong crystallization, while NDITEG/TEG
G0 formed amorphous liquid. The molecular design strategy using immiscible side chain pairs potentially enables a variety of π-systems to stack up to form a columnar phase rather than other ordered phases, regardless of the chain/core volume balance.
Introduction
Columnar liquid crystals (LCs) based on discotic π-systems have attracted interest due to their potential use as soft organic semiconductors with unidirectional charge carrier transporting properties.1–3 If the methodology to obtain columnar mesophases with largely π-conjugated cores by rational molecular design strategies is established, we can use various active π-systems as photo and electronic devices. For discotic LC molecules, the major driving force to enable columnar structures via self-assembly is mostly given by the peripheral modification of disc-shaped cores with long paraffinic chains,4–7 where rigid π-systems and flexible side chains are segregated in a nanoscopic scale. Nevertheless, the volume balance between a discotic core and peripheral chains has a remarkable impact on the stable phase structures,8 leading to the formation of other phases such as micelle cubic,9–11 bicontinuous cubic,12–14 crystalline,15,16 and amorphous phases.17,18 Therefore, in order to obtain columnar LC materials, the try-and-error in the synthesis process is required for every discotic π-system such as changing the number and length of chains. Here we demonstrate a potentially general molecular design strategy using an immiscible side chain pair19–26 (Fig. 1) that preferentially induces the formation of a rectangular columnar mesophase rather than other non-columnar phases. As a proof of this strategy, we selected typical n-type semiconducting cores, perylenediimide (PDI)27,28 and naphthalenediimide (NDI),29,30 that are easily available for site-specific introduction of different side chain functionalities.31,32 As an immiscible chain pair, dodecyl (hydrophobic) and triethyleneglycol (hydrophilic) chains were adopted and introduced into the imide positions of the PDI or NDI cores. Since contact between hydrophobic and hydrophilic chains is thermodynamically unfavorable, PDIC12/TEG
G0, a PDI molecule having the immiscible side chain pair (Fig. 1), allows discotic cores to avoid the phase structures containing large intermolecular paraffinic-oxyethylene contacts and thus eventually form a columnar structure with hydrophobic/hydrophilic nano-segregated layers (Fig. 2). Of interest, we found that this preference was also confirmed when the volume balance between the core and side chains is changed as seen in PDIG1 and NDIG0 series (Fig. 1). These results support our concept and propose a potentially general molecular design strategy to realize self-assembled columnar structures from a variety of large planar π-systems.
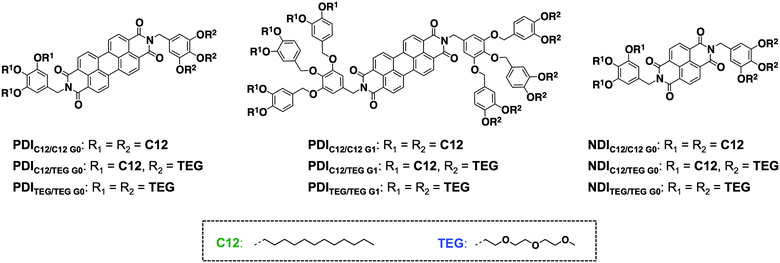 |
| Fig. 1 Molecular structures of the perylenediimide (PDI) and naphthalenediimide (NDI) derivatives with hydrophobic and/or hydrophilic peripheral side chains. | |
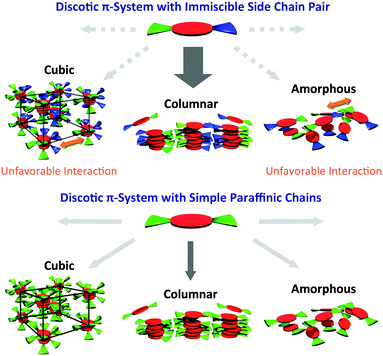 |
| Fig. 2 Conceptual illustrations of the preferential formation of columnar mesophase via peripheral modification of immiscible side chain pairs. The orange arrows indicate thermodynamically unfavourable contacts. | |
Results and discussion
Synthesis
The PDI derivatives PDIC12/TEG
G0, PDITEG/TEG
G0, PDIC12/C12
G1, PDIC12/TEG
G1, and PDITEG/TEG
G1 (Fig. 1) were newly synthesized through the reaction using perylenetetracarboxylic dianhydride and taper-shaped amine compounds having dodecyl or TEG chains (see ESI†). PDIC12/C12
G0 was synthesized according to the reported procedures with a slight modification. The NDI derivatives were also prepared by following the similar synthetic schemes used in the PDI synthesis. Isolation and purification were successfully carried out by column chromatography using silica gel, followed by the recycling preparative size-exclusion chromatography, and then reprecipitation (see ESI†). All the PDI and NDI compounds were fully characterized by 1H and 13C NMR spectroscopy and MALDI-TOF-MS spectrometry (see ESI†).
Liquid crystalline property of G0-PDI derivatives
Differential scanning calorimetry (DSC) revealed that PDIC12/C12
G0 showed a mesophase from −30 to 220 °C in the second cooling process at a rate of 10 °C min−1 (Table 1 and Fig. S1, ESI†). In the mesophase, birefringent textures were observed in polarized optical microscopy (POM) (Fig. 3a) while the mesophase structure was assigned to a columnar hexagonal lattice by means of X-ray diffraction analysis (XRD), both of which are consistent with the previous reports.33,34 From the (100) diffraction peak in the XRD pattern, a lattice parameter a = 30.9 Å at 70 °C was disclosed, and one relatively broad peak was assigned to a π-stacking periodicity of c = 3.4 Å (Fig. 4a). In the solid state below ca. −30 °C, the observed diffraction peaks in its XRD pattern became obviously broad (Fig. S3, ESI†) though the set of the peaks was still assigned to a hexagonal lattice, indicating that the material was in a LC-glass phase35 (Table 1). The diffraction corresponding to the π–π plane was confirmed at all the measured temperatures below the clearing point. In fact, spin-coated thin films of PDIC12/C12
G0 afforded electronic absorption spectra typical of H-type π-stacked PDI aggregates (Fig. 5a),36 displaying a blue shift and broadening of the absorption bands at around 500 nm compared to that in a good solvent such as CHCl3.
Table 1 Phase behaviors with transition temperatures (°C) and enthalpy changes (kJ mol−1) in the second heating/cooling process. Scanning rate was 10 °C min−1. The symbols G, Cr, Colh, Colr, Cub, X, and Iso in the phase behaviors denote liquid crystalline glassy, crystalline, hexagonal columnar, rectangular columnar, micellar cubic, unidentified, and isotropic phases, respectively. Symbol * indicates the observation of clear π–π-stacking periodicity in XRD
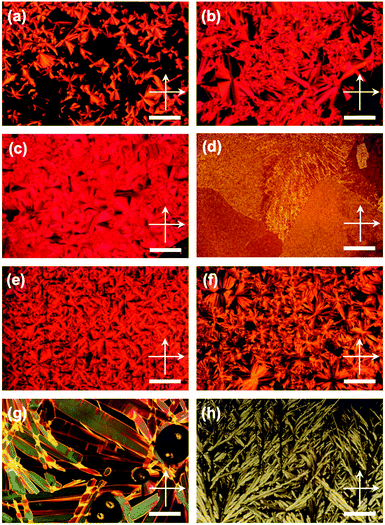 |
| Fig. 3 Crossed polarized optical micrographs of (a) PDIC12/C12 G0 at 190 °C, (b) PDIC12/TEG G0 at 180 °C, (c) PDITEG/TEG G0 at 160 °C, (d) PDIC12/C12 G1 at 90 °C, (e) PDIC12/TEG G1 at 148 °C, (f) PDITEG/TEG G1 at 93 °C, (g) NDIC12/C12 G0 at 140 °C and (h) NDIC12/TEG G0 at 87 °C. Scale bars indicate 200 μm. | |
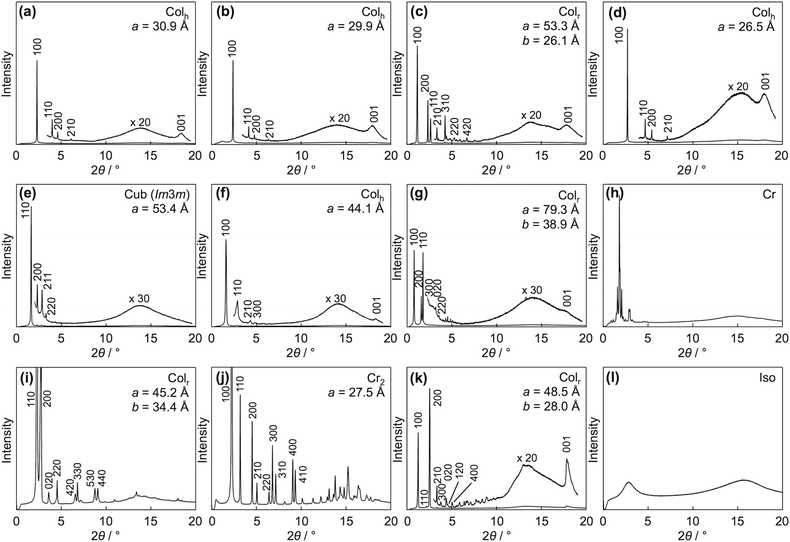 |
| Fig. 4 X-ray diffraction patterns of (a) PDIC12/C12 G0 at 70 °C, (b) PDIC12/TEG G0 at 170 °C, (c) PDIC12/TEG G0 at 90 °C, (d) PDITEG/TEG G0 at 80 °C, (e) PDIC12/C12 G1 at 110 °C, (f) PDIC12/C12 G1 at 50 °C, (g) PDIC12/TEG G1 at 149 °C, (h) PDITEG/TEG G1 at 90 °C, (i) NDIC12/C12 G0 at 90 °C, (j) NDIC12/C12 G0 at 60 °C, (k) NDIC12/TEG G0 at 90 °C, and (l) NDITEG/TEG G0 at 30 °C. | |
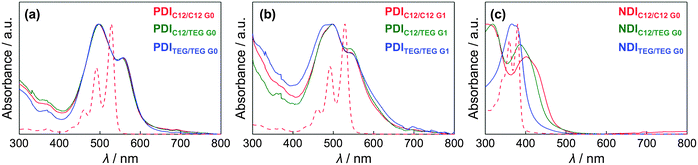 |
| Fig. 5 Absorption spectra of the (a) PDIG0, (b) PDIG1, and (c) NDIG0 derivatives in the film state (solid line) together with the spectra of (a) PDIC12/C12 G0, (b) PDIC12/C12 G1, and (c) NDIC12/C12 G0 in CHCl3 (dashed line) at 25 °C. | |
With these results in mind, the other compounds were characterized in the identical manner. When cooled down from its isotropic melt, PDITEG/TEG
G0 exhibited a phase transition into a mesophase at 168 °C in which the dendritic texture developed in POM (Fig. 3c). The lower clearing point than that of PDIC12/C12
G0 probably originates from the more flexible nature of TEG than that of dodecyl chains, which was often seen in the previous reports.37 In addition, XRD analysis revealed that the observed mesophase was a hexagonal columnar phase with a π–π distance of c = 3.4 Å (Fig. 4d). The lattice parameter a was calculated as 26.5 Å for PDITEG/TEG
G0, which agrees with the shorter chain length of TEG than that of dodecyl. One notable behavior of PDITEG/TEG
G0 is that the phase transition at 46 °C, detected by DSC measurements at 10 °C min−1 upon cooling (Fig. S1, ESI†), was not a LC/glass but LC/crystal transition. For example, the XRD pattern at 20 °C showed several minor but sharp diffraction peaks that did not appear at 80 °C (Fig. S5, ESI†). The contrast was again explained by the flexibility of side chains. Upon cooling from the LC phase, dodecyl chains in PDIC12/C12
G0 have a driving force to interdigitate each other, resulting in the freezing of the hexagonal columnar structure and thus showing broad peaks in XRD. On the other hand, TEG chains have much smaller inter-chain interactions, as evident from the smaller enthalpy values upon the Cr–LC transition (Table 1). Therefore, PDI cores as well as benzyl units in PDITEG/TEG
G0 can crystallize, as if in solution, into their stable packing structure. When focused on the melting points, it was found that the melting point of PDITEG/TEG
G0 (52 °C) was higher than that of PDIC12/C12
G0 (−28 °C). However, this is a delicate issue that strongly relies on the substitution patterns of chains to the core. As discussed above, dodecyl chains represent stronger inter-chain interactions upon freezing (larger ΔH: 13.0 kJ mol−1). At the same time, they show a larger entropy loss (larger ΔS: 26.3 J mol−1) (Table S1, ESI†). Because of this entropy–enthalpy compensation (Fig. S2, ESI†),38 one cannot easily expect the melting point. In the present substitution pattern of the PDIG0 system, higher melting temperature was observed for PDITEG/TEG
G0.
PDIC12/TEG
G0 showed two mesophases in DSC at 18–112 and 112–191 °C upon 10 °C min−1 cooling (Table 1, Fig. 3b and Fig. S1, ESI†). XRD measurements revealed that the higher-temperature mesophase was assigned to a hexagonal columnar phase with a lattice parameter of e.g. a = 29.9 Å and c = 3.5 Å at 170 °C (Fig. 4b). In contrast, the lower-temperature mesophase was featured by its characteristic structural symmetry. For example, the (100) peak, usually not detected in common rectangular phases with high symmetry, was observed as seen in Fig. 4c. After complete assignment of all the clear diffraction peaks, we found that the LC phase at 170 °C was a rectangular columnar lattice with p2mg symmetry,22,23 where the lattice parameters of a and b are 53.3 and 26.1 Å at 90 °C, respectively. This specific structure can be explained as a result of side-chain immiscibility.19–26
Namely, because of the enthalpic gain, dodecyl chains prefer to organize with themselves and vice versa for the TEG chains. Thus, to minimize the contact between dodecyl and TEG domains, PDIC12/TEG
G0 molecules stack up to form the columnar structure that further integrates into alternate immiscible nano-scale layers (Fig. 6). Consequently, a rectangular columnar LC phase with p2mg symmetry is realized. This mesophase structure, upon elevating the temperature, undergoes phase transition into the hexagonal columnar mesophase at 113 °C, which is probably due to the partially mixed dodecyl and TEG chains that lead to the higher-symmetry phase (Fig. 6). The above possible mechanism was also supported by another PDI derivative carrying dodecyl and semifluoroalkyl chains reported recently.39,40 We believe that the combined use of two immiscible chains as peripheral substituents generally enables a variety of π-conjugated motifs to form columnar mesophases. It should be noted that both PDIC12/TEG
G0 and PDITEG/TEG
G0 displayed absorption spectra similar to that of PDIC12/C12
G0 in their spin-coated films (Fig. 5). We concluded that the actual π-stacking geometry of PDI units within a columnar structure is not essentially different among the three materials. We should mention the previous work by Thelakkat et al.,32 where the modification by a pair of two-blanched immiscible chains results in the formation of hexagonal columnar mesophases. It suggested the importance of core/chain volume balances on the selection of hexagonal/rectangular geometry.26
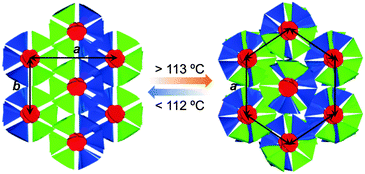 |
| Fig. 6 Schematic illustrations of molecular arrangements in rectangular and hexagonal columnar phases observed in PDIC12/TEG G0 with a unit cell. The red discs and green/blue triangular prisms indicate PDI cores and hydrophobic/hydrophilic chain parts, respectively. | |
Liquid crystalline properties of G1-PDI derivatives
Then our motivation moved on to the acceptable range of the proposed effect by immiscible side chains. We wondered that which type of mesophases appeared if the volume of the side chains get larger or smaller. For this purpose, PDIG1 series were prepared (Fig. 1). PDIC12/C12
G1 exhibited two mesophases at −3–90 °C and 90–186 °C on second cooling (Table 1 and Fig. S1, ESI†). However, no optical texture was appeared in POM above ca. 90 °C, even though the isotropic melt of PDIC12/C12
G1 was cooled down. The fact possibly reflects the presence of an optically-isotropic but structurally-ordered mesophase. By means of XRD analysis, PDIC12/C12
G1 at 110 °C gave a set of diffractions at 2θ = 1.64, 2.32, 2.85 and 3.28° that are not assignable to common columnar phases (Fig. 4e). In fact, the higher-temperature mesophase was assigned to a micellar cubic phase with the Im3m symmetry. Considering the lattice parameter a = 53.4 Å at that temperature, the probable molecular arrangement is composed of several stacks of PDI cores that are covered with a shell of dodecyl chains (Fig. 2 and 7). The reason of the formation of the micellar cubic phase is mostly the large volume of side chains. Nevertheless, when the temperature was decreased below 90 °C to reduce the contribution of the entropy term, PDIC12/C12
G1 formed a hexagonal columnar phase (Fig. 4f and Fig. S6, ESI†), which is indicative of the π-stacking capability among PDI chromophores (Fig. 5). Accordingly, birefringent textures developed at 90 °C under crossed polarized conditions (Fig. 3d and Fig. S12, ESI†). The large hysteresis of this first-order phase transition, confirmed by DSC measurements (Fig. S1, ESI†), suggests that the structural transition from the micellar cubic to the hexagonal columnar phase takes a certain period of time to allow the huge volume of side chains to move and orient properly. Upon further cooling, PDIC12/C12
G1, like PDIC12/C12
G0, adopted a LC-glass state at a temperature below ca. 0 °C (Table 1 and Fig. S1, S6, ESI†). This phase transition is mostly driven by a freeze of alkyl chains. The reason of higher transition temperature of PDIC12/C12
G1 than that of PDIC12/C12
G0 is attributed to the crowded environment of dodecyl chains. Upon melting of the chains along the glass-LC transition, dodecyl chains get a smaller degree of freedom as compared to the case of PDIC12/C12
G0. Namely, the entropic gain per single chain is smaller for PDIC12/C12
G1, whereas the enthalpic penalty may be comparable (Table S1, ESI†), resulting in a higher transition temperature (TG-LC = ΔHG-LC/ΔSG-LC) for PDIC12/C12
G1.
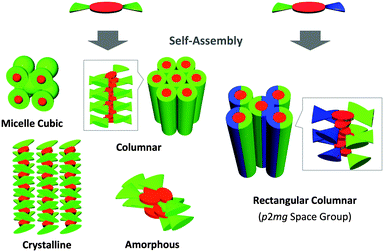 |
| Fig. 7 Summary of phase behaviors depending on side-chain substitution patterns demonstrated for PDI/NDI systems in this study. The orange, green, and blue parts indicate π-conjugated cores and hydrophobic and hydrophilic chains, respectively. | |
On the other hand, PDITEG/TEG
G1 showed a simple DSC curve basically containing one phase transition upon both heating and cooling processes (Table 1 and Fig. S1, ESI†). Although birefringent textures appeared in POM at 93 °C (Fig. 3f), the lower-temperature phase is not liquid crystalline. For example, the obtained XRD pattern at 90 °C includes several notable diffraction peaks (Fig. 4h and Fig. S8, ESI†) that however were not assignable to simple columnar, lamellar, or cubic phases. We gave up clarifying the detailed phase structure and denoted it as an unidentified crystalline phase. In this solid phase, PDITEG/TEG
G1 contains π-stacked aggregates, which was proved by absorption spectral study in the film state (Fig. 5b). The absence of LC phase for PDITEG/TEG
G1 is most likely due to the bulky substituents with too fluidic TEG chains. TEG chains in this crowded environment do not receive the entropic gain enough for stabilizing the LC phase as a thermodynamically stable phase. This issue is delicate and difficult to discuss in a general manner.
PDIC12/TEG
G1 showed two mesophases at −3–147 °C and 147–154 °C (Table 1 and Fig. S1, ESI†). Upon cooling from its isotropic melt, PDIC12/TEG
G1 at 148 °C afforded birefringent focal-conic textures in POM, indicative of a columnar mesophase (Fig. 3e). Indeed, XRD measurements disclosed that the dominant diffraction peaks were assigned to (100), (200), and (110) in a rectangular lattice with the p2mg symmetry, where the lattice parameters of a and b are 79.3 and 38.9 Å at 149 °C, respectively (Fig. 4g). Moreover, though the XRD patterns involved unidentified crystal-like peaks upon cooling in this mesophase (Fig. S7, ESI†), still (100), (200) and (110) peaks in a rectangular lattice mainly appeared. Taking into account the π-stacking interactions among PDI units (Fig. 5), PDIC12/TEG
G1 was found to behave similar to PDIC12/TEG
G0 because of the modification with immiscible side chains. One distinct difference is the absence of a hexagonal columnar phase. If the PDIC12/TEG
G1 molecules move significantly in translational or rotational directions, the mixing of large volume of dodecyl and TEG chains takes place, leading to a considerable enthalpic penalty. Therefore, PDIC12/TEG
G1 maintains the rectangular columnar structure even upon elevating the temperature. Unfortunately, we could not obtain information of a higher-temperature mesophase at 147–154 °C (on cooling) despite the detailed VT-XRD measurements. The other interesting matter to note is that XRD patterns in the temperature region of the Colr phase showed broad diffraction at 2θ = ca. 14 and 16° (Fig. S7, ESI†). These diffraction peaks strongly indicate the halo of dodecyl and TEG chains, respectively, which proves the presence of nanoscale immiscible domains.
Through the above experimental results, the peripheral modification of immiscible side chain pairs seems to extend the acceptable range of core/chain volume balance for columnar LC formation, whose driving force was given by the unstabilization of other possible mesophase candidates (Fig. 2 and 7).
Liquid crystalline properties of G0-NDI derivatives
For tuning the core/chains volume balance, there is another approach using smaller π-conjugated motifs than PDI without changing the G0-type peripheral units. For this purpose, we synthesized NDIG0 series (Fig. 1) and investigated their phase transition behaviors and structural information. Based on the DSC curves scanned at a rate of 10 °C min−1, NDIC12/C12
G0 has three solid and one isotropic liquid phases (Table 1 and Fig. S1, ESI†). Upon cooling from its isotropic melt, NDIC12/C12
G0 obviously crystallizes at 140 °C (Fig. 3g). The typical XRD patterns indicate the high crystallinity of all the three solid phases, though we only denote the highest-temperature mesophase as Colr (Fig. 4i, j and Fig. S9, ESI†). In addition, judging from the XRD patterns (Fig. S9, ESI†), the diffraction peak corresponding to the π-stacking periodicity, if any, was quite weak.
In contrast, NDITEG/TEG
G0 forms isotropic liquid even at a room temperature. Its POM images are dark and only broad peaks were observed in its XRD patterns (Fig. 4l), while its DSC curves are almost planar (Fig. S1, ESI†). Considering the behavior of NDIC12/C12
G0 and NDITEG/TEG
G0, the π-stacking capability of the NDI system is so weak that the properties of side chains are dominant for the whole molecule—NDIC12/C12
G0 strongly crystallizes due to the interdigitation of dodecyl chains while NDITEG/TEG
G0 is an isotropic liquid due to the flexibility of TEG chains.
Similar to PDIC12/TEG
G0 and PDIC12/TEG
G1, NDIC12/TEG
G0 forms a rectangular columnar phase with the p2mg symmetry, which was confirmed by the presence of the 100 diffraction peak in XRD (Fig. 4k). Meanwhile, some hysteresis was observed in its DSC curves (Fig. S1, ESI†) and minor but sharp diffractions were appeared in XRD (Fig. 4k and Fig. S10, ESI†), both of which imply the crystalline nature of the materials. However, NDIC12/TEG
G0 has characteristic features different from those of NDIC12/C12
G0. NDIC12/TEG
G0 in a glass cell showed fan-shaped textures at 140 °C in POM (Fig. 3h). Furthermore, it displayed a clear π-stacking periodicity of c = 3.46 Å in XRD patterns (Fig. 4k and Fig. S10, ESI†). Overall, immiscible chains play a role in forcing the NDI core to stack up in a columnar fashion, affording p2mg rectangular columnar structures. When looking at the thermodynamic parameters, different behaviors of NDI series are obvious. By plotting the entropy and enthalpy values upon the LC–Iso phase transition (Fig. S2, ESI†), the entropy–enthalpy compensation was confirmed. However, the linear correlation is different between the PDI and NDI compounds having mesophases. The fact implies the large contribution of inter-chain interactions to the Colr–Iso transitions that is not commonly observed for LC–Iso transitions. However, what we would like to emphasize here is that the immiscible side chain pairs work to develop the columnar mesophase with a periodically-stacked π-system in both LC (PDIC12/TEG
G0 and PDIC12/TEG
G1) and crystalline (NDIC12/TEG
G0) cases.
Conclusions
Motivated by our previous findings,22,23 where the modification with a immiscible side chain pair makes a π-system form a columnar liquid crystalline phase, here we have investigated the effect of immiscible side chain pairs on the mesomorphism of discotic π-systems in a more systematic and general way. We prepared two types of discotic cores, PDI and NDI, two types of peripheral units, three-branched G0- and six-branched G1-type dendronized chains, and immiscible chain pairs, alkyl and TEG chains. After recognizing that the combination of PDI and G0 chains, PDIC12/C12
G0, PDIC12/TEG
G0, and PDITEG/TEG
G0, all form columnar mesophases, we studied PDIG1 and NDIG0 systems having larger volumes of side chains to the cores. As a result, when the side chain volume becomes much larger, the derivatives having one type of (dodecyl or TEG) peripheral chains adopt micellar cubic, crystalline, or isotropic liquid phases. In sharp contrast, all three compounds having the immiscible side chain pair form rectangular columnar mesophases involving π-stacked arrays. The dominant reason of the observed preferential formation of columnar phases is the consequence of the exclusion of other phases, where a thermodynamically unfavorable contact between hydrophobic and hydrophilic chains considerably takes place. The molecular design strategy demonstrated here would extend the acceptable range of core/chain volume balance for columnar LC formation and would enable a variety of π-conjugated motifs to form columnar mesophases by side-chain directed assembly.
Acknowledgements
This work was supported by a Grant-in-Aid for Young Scientists (B) (26810049) from the Japan Society for the Promotion of Science. We thank Prof. Dr Takuzo Aida and Mr Yoshiki Shibuya in The Univ. of Tokyo for DSC measurements and Dr Akinori Saeki in Osaka Univ. for helpful discussions. The synchrotron radiation experiments were performed at BL44B241 in SPring-8 with the approval of RIKEN (Proposal No. 20130021).
Notes and references
- D. Adam, P. Schuhmacher, J. Simmerer and L. Häussling, Nature, 1994, 371, 141 CrossRef CAS
.
- K. Ohta, K. Hatsusaka, M. Sugibayashi, M. Ariyoshi, K. Ban, F. Maeda, R. Naito and K. Nishizawa, Mol. Cryst. Liq. Cryst., 2003, 397, 325 CAS
.
- C. Simpson, J. Wu, M. Watson and K. Mullen, J. Mater. Chem., 2004, 14, 494 RSC
.
- S. Laschat, A. Baro, N. Steinke, F. Giesselmann, C. Haegele, G. Scalia, R. Judele, E. Kapatsina, S. Sauer, A. Schreivogel and M. Tosoni, Angew. Chem., Int. Ed., 2007, 46, 4832 CrossRef CAS PubMed
.
- S. Sergeyev, W. Pisula and Y. H. Geerts, Chem. Soc. Rev., 2007, 36, 1902 RSC
.
- W. Pisula, M. Zorn, J. Y. Chang, K. Müllen and R. Zentel, Macromol. Rapid Commun., 2009, 30, 1179 CrossRef CAS PubMed
.
- T. Kato, T. Yasuda, Y. Kamikawa and M. Yoshio, Chem. Commun., 2009, 729 RSC
.
- B. M. Rosen, D. A. Wilson, C. J. Wilson, M. Peterca, B. C. Won, C. Huang, L. R. Lipski, X. Zeng, G. Ungar, P. A. Heiney and V. Percec, J. Am. Chem. Soc., 2009, 131, 17500 CrossRef CAS PubMed
.
- P. Sakya, J. M. Seddon, R. H. Templer, R. J. Mirkin and G. J. T. Tiddy, Langmuir, 1997, 13, 3706 CrossRef CAS
.
- K. Borisch, S. Diele, P. Goring, H. Kresse and C. Tschierske, J. Mater. Chem., 1998, 8, 529 RSC
.
- T. Yasuda, H. Ooi, J. Morita, Y. Akama, K. Minoura, M. Funahashi, T. Shimomura and T. Kato, Adv. Funct. Mater., 2009, 19, 411 CrossRef CAS
.
- S. N. Chvalun, M. A. Shcherbina, A. N. Yakunin, J. Blackwell and V. Percec, Polym. Sci., Ser. A, 2007, 49, 158 CrossRef
.
- S. Coco, C. Cordovilla, B. Donnio, P. Espinet, M. J. Garcia-Casas and D. Guillon, Chem. – Eur. J., 2008, 14, 3544 CrossRef CAS PubMed
.
- M. A. Alam, J. Motoyanagi, Y. Yamamoto, T. Fukushima, J. Kim, K. Kato, M. Takata, A. Saeki, S. Seki, S. Tagawa and T. Aida, J. Am. Chem. Soc., 2009, 131, 17722 CrossRef CAS PubMed
.
- G. C. Shearman, G. Yahioglu, J. Kirstein, L. R. Milgrom and J. M. Seddon, J. Mater. Chem., 2009, 19, 598 RSC
.
- Q. Xiao, T. Sakurai, T. Fukino, K. Akaike, Y. Honsho, A. Saeki, S. Seki, K. Kato, M. Takata and T. Aida, J. Am. Chem. Soc., 2013, 135, 18268 CrossRef CAS PubMed
.
- A. Nowak-Kŕol, D. Gryko and D. T. Gryko, Chem. – Asian J., 2010, 5, 904 CrossRef PubMed
.
- S. Maruyama, K. Sato and H. Iwahashi, Chem. Lett., 2010, 39, 714 CrossRef CAS
.
- C. Tschierske, J. Mater. Chem., 2001, 11, 2647 RSC
.
- M. Lehmann, Chem. – Eur. J., 2009, 15, 3638 CrossRef CAS PubMed
.
- X. Feng, V. Marcon, W. Pisula, M. R. Hansen, J. Kirkpatrick, F. Grozema, D. Andrienko, K. Kremer and K. Müllen, Nat. Mater., 2009, 8, 421 CrossRef CAS PubMed
.
- T. Sakurai, K. Shi, H. Sato, K. Tashiro, A. Osuka, A. Saeki, S. Seki, S. Tagawa, S. Sasaki, H. Masunaga, K. Osaka, M. Takata and T. Aida, J. Am. Chem. Soc., 2008, 130, 13812 CrossRef CAS PubMed
.
- T. Sakurai, K. Tashiro, Y. Honsho, A. Saeki, S. Seki, A. Osuka, A. Muranaka, M. Uchiyama, J. Kim, S. Ha, K. Kato, M. Takata and T. Aida, J. Am. Chem. Soc., 2011, 133, 6537 CrossRef CAS PubMed
.
- M.-C. Yeh, Y.-L. Su, M.-C. Tzeng, C. W. Ong, T. Kajitani, H. Enozawa, M. Takata, Y. Koizumi, A. Saeki, S. Seki and T. Fukushima, Angew. Chem., Int. Ed., 2013, 52, 1031 CrossRef CAS PubMed
.
- S. Yagai, S. Okamura, Y. Nakano, M. Yamauchi, K. Kishikawa, T. Karatsu, A. Kitamura, A. Ueno, D. Kuzuhara, H. Yamada, T. Seki and H. Ito, Nat. Commun., 2013, 5, 5013 Search PubMed
.
- C. Tschierske, Angew. Chem., Int. Ed., 2013, 52, 8828 CrossRef CAS PubMed
.
- F. Würthner, C. R. Saha-Möller, B. Fimmel, S. Ogi, P. Leowanawat and D. Schmidt, Chem. Rev., 2015, 115 DOI:10.1021/acs.chemrev.5b00188
.
- F. Würthner, C. Thalacker, S. Diele and C. Tschierske, Chem. – Eur. J., 2001, 7, 2245 CrossRef
.
- S.-L. Suraru and F. Würthner, Angew. Chem., Int. Ed., 2014, 53, 7428 CrossRef CAS PubMed
.
- N. Sakai, J. Mareda, E. Vauthey and S. Matile, Chem. Commun., 2010, 46, 4225 RSC
.
- E. Schwartz, V. Palermo, C. E. Finlayson, Y.-S. Huang, M. B. L. Otten, A. Liscio, S. Trapani, I. Gonzalez-Valls, P. Brocorens, J. J. L. M. Cornelissen, K. Peneva, K. Müllen, F. C. Spano, A. Yartsev, S. Westenhoff, R. H. Friend, D. Beljonne, R. J. M. Nolte, P. Samori and A. E. Rowan, Chem. – Eur. J., 2009, 15, 2536 CrossRef CAS PubMed
.
- A. Wicklein, A. Lang, M. Muth and M. Thelakkat, J. Am. Chem. Soc., 2009, 131, 14442 CrossRef CAS PubMed
.
- J. van Herrikhuyzen, A. Syamakumari, A. P. H. J. Schenning and E. W. Meijer, J. Am. Chem. Soc., 2004, 126, 10021 CrossRef CAS PubMed
.
- V. Percec, M. Peterca, T. Tadjiev, X. Zeng, G. Ungar, P. Leowanawat, E. Aqad, M. R. Imam, B. M. Rosen, U. Akbey, R. Graf, S. Sekharan, D. Sebastiani, H. W. Spiess, P. A. Heiney and S. D. Hudson, J. Am. Chem. Soc., 2011, 133, 12197 CrossRef CAS PubMed
.
- L.-Y. Chen, T.-H. Ke, C.-C. Wu, T.-C. Chao, K.-T. Wong and C.-C. Chang, Appl. Phys. Lett., 2007, 91, 163509 CrossRef
.
- S. Yagai, T. Seki, T. Karatsu, A. Kitamura and F. Würthner, Angew. Chem., Int. Ed., 2007, 47, 3367 CrossRef PubMed
.
- G. J. Clarkson, N. B. McKeown and K. E. Treacher, J. Chem. Soc., Perkin Trans. 1, 1995, 1817 RSC
.
- A. A. Levchenko, C. K. Yee, A. N. Parikh and A. Navrotsky, Chem. Mater., 2005, 17, 5428 CrossRef CAS
.
- Y. Tsutsui, T. Sakurai, K. Kato, M. Takata and S. Seki, J. Photopolym. Sci. Technol., 2015, 28, 583 CrossRef CAS
.
- T. Sakurai, Y. Tsutsui, W. Choi and S. Seki, Chem. Lett., 2015, 44, 1401 CrossRef
.
- K. Kato, R. Hirose, M. Takemoto, S. Ha, J. Kim, M. Higuchi, R. Matsuda, S. Kitagawa and M. Takata, AIP Conf. Proc., 2010, 1234, 875 CrossRef CAS
.
Footnote |
† Electronic supplementary information (ESI) available: Detailed synthetic procedures of materials, UV-vis spectra, temperature dependent XRD patterns and DSC thermograms. See DOI: 10.1039/c6tc00021e |
|
This journal is © The Royal Society of Chemistry 2016 |