DOI:
10.1039/C6TA08670E
(Paper)
J. Mater. Chem. A, 2016,
4, 17749-17756
Preparation of a hybrid Cu2O/CuMoO4 nanosheet electrode for high-performance asymmetric supercapacitors†
Received
6th October 2016
, Accepted 24th October 2016
First published on 24th October 2016
Abstract
Supercapacitors have attracted considerable attention due to their fast charge–discharge ability, excellent rate capability and long-term stability. In this study, a novel electrode composed of Cu2O/CuMoO4 nanosheets directly grown on Ni foam was synthesized via a facile hydrothermal method. The as-prepared electrode exhibits remarkably improved specific and areal capacitance (up to 4264 F g−1 and 9.38 F cm−2 at a current density of 1 A g−1). To the best of our knowledge, the specific capacitance of 4264 F g−1 is the highest in reported studies although the areal capacitance of 9.38 F g−1 is slightly lower than the highest value of 16 F cm−2 when Ni(OH)2/carbon nanotubes on Ni foam was used as the electrode. An asymmetric supercapacitor is fabricated using the Cu2O/CuMoO4 nanosheet electrode as the positive electrode and activated carbon (AC) as the negative electrode. The operation voltage could expand to 1.7 V, at which the energy density can reach 75.1 W h kg−1 with a power density of 420 W kg−1. Additionally, the asymmetric supercapacitor exhibits very high rate capability and good cycling stability. The capacitance remains around 86.6% after 3000 cycles at a current density of 5 A g−1. This indicates that the Cu2O/CuMoO4 nanosheet electrode is promising for applications in renewable energy storage in the future.
1. Introduction
Energy and power demands keep increasing year by year due to the fast development of the world. Traditional energy supply is mainly based on the combustion of fossil fuels which causes many environmental issues. To avoid and address these problems, many researchers have started to develop alternative energy storage and conversion systems. The electrochemical capacitor (EC), also called supercapacitor, as one of the promising new classes of energy storage devices, has attracted significant interest due to its rapid charge–discharge rate, high power density and long life span.1–6 Presently, most commercial supercapacitors are symmetric and carbon material-based, which are known as electric double layer capacitors (EDLCs).7–9 The carbon-based material usually has low energy density which restricts its further application compared to batteries.10,11 Therefore, it is necessary to develop high quality supercapacitors with improved energy density without sacrificing the power density. Asymmetric capacitors are considered as one of the best alternatives because the redox reactions during charge–discharge processes can lead to larger specific capacitance.12,13 In a typical asymmetric supercapacitor, one electrode is derived from carbon-based materials while the other one is made of pseudocapacitive materials. It can combine the advantages of the two types of materials to exhibit high operating potential, and achieve high energy density. The performances of asymmetric supercapacitors are determined by the properties of the specific electrode materials. Therefore, it is important to develop novel pseudocapacitive materials.
Many efforts have been made to explore the pseudocapacitive materials for supercapacitors, and transition-metal oxides/hydroxides are the commonly used materials.14–17 In particular, mixed metal oxides/hydroxides usually offer better electrical performances because of their improved conductivity as well as their synergic effects.18–22 For example, Liu et al. found that CoMoO4–NiMoO4 exhibited higher specific capacitance than CoMoO4 and better rate capability than NiMoO4.23 Similarly, the investigation on Co–Ni double hydroxides has proved that the hybrid hydroxide electrodes significantly outperform either Co(OH)2 or Ni(OH)2 alone.24–26 Nevertheless, the performance of pseudocapacitive materials still suffers from intrinsic poor electric conductivity and insufficient diffusion of the electrolyte into the active materials, namely, a large portion of the active materials are not accessible to the electrolyte to undergo faradaic reactions during the charge–discharge process thus no contribution to the specific capacitance.27 Developing nanostructured materials with a large surface area is regarded as an effective strategy to overcome these issues. In addition, directly growing the active materials on the conductive substrate can reduce internal resistance and make full use of the active materials, therefore improving the capacitance and stability.28–30 Among different oxide materials for supercapacitors, copper oxides have drawn much attention in supercapacitors due to their low cost, environmental benignity and abundance.31–33 Shinde et al. prepared 3D-flower-like CuO on copper foil which exhibited 498 F g−1 at 5 mV s−1.34 Wang et al. measured the properties of CuO nanosheets, and a capacity of 569 F g−1 was obtained at a current density of 5 mA cm−2 in 6.0 M KOH electrolyte.35 However, it is still a big challenge for commercial utilisation of Cu oxide supercapacitors, and more studies should be conducted to achieve the commercial requirements. By combining the aforementioned strategies, it can be deduced that better performance would be obtained if nanostructured copper oxide hybrids can be directly grown on conductive substrates. On the other hand, to the best of our knowledge, the application of CuMoO4 for supercapacitors has not been reported. Herein, Cu2O/CuMoO4 nanosheets directly grown on Ni foam have been synthesized. The as-prepared electrode exhibits high specific and areal capacitance and also displays good rate capability. In addition, excellent electrical performances are obtained in the asymmetric supercapacitor with AC as the negative electrode. An energy density of 75.1 W h kg−1 and a power density of 420 W kg−1 can be achieved with the voltage window at 1.7 V. Taking the fascinating performances and easy fabrication procedures into account, the hybrid Cu2O/CuMoO4 nanosheet electrode is promising for commercial application in high-energy storage in the future.
2. Experimental section
2.1. Preparation of hybrid Cu2O/CuMoO4 nanosheets on Ni foam
All the reagents were used without further purification. Cu2O/CuMoO4 nanosheets were synthesized by a facile hydrothermal method. Prior to the synthesis, the Ni foam (1 × 1 cm2) was cleaned by ultra-sonication in 2 M HCl solution, ethanol and deionized water for several minutes, respectively. The solution containing 0.05 M Cu(NO3)2 and 0.05 M Na2MoO4 was obtained by dissolving copper(II) nitrate hemi(pentahydrate) and sodium molybdate dihydrate in deionized water under constant stirring. The prepared solution was transferred into a Teflon lined stainless steel autoclave. Ni foam was immersed in the homogeneous solution, and then the autoclave was heated at 160 °C for 15 h and naturally cooled down to room temperature. The Ni foam was taken out and washed with water and ethanol to remove surface ions and molecules, and then followed by drying at 60 °C overnight in a vacuum oven. Finally, the Ni foam with the as-grown hydrate precursors was calcined at 450 °C for 2 h in an argon atmosphere with a heating rate of 1 °C min−1. The mass loading of the active materials was obtained by comparing the weight of the Ni foam before the hydrothermal reaction and after the calcination, which was determined to be around 2.2 mg cm−2. For the sake of comparison, the precursor solution without Na2MoO4 was applied to fabricate electrodes by the same process.
2.2. Assembly of the Cu2O/CuMoO4//AC asymmetric supercapacitor
To fabricate the asymmetric supercapacitor, the as-prepared Cu2O/CuMoO4 electrode was used as the positive electrode and an activated carbon (AC) electrode acted as the negative electrode. The AC electrode was obtained by mixing the activated carbon (Black Pearl 2000, Cabot) and polytetrafluoroethylene at a mass ratio of 95
:
5 in water under constant magnetic stirring. The prepared slurry was spread onto a Ni foam (1 × 1 cm2) and then pressed and dried at 60 °C overnight under vacuum. The mass ratio of Cu2O/CuMoO4 nanosheets and AC was optimized to be around 2.2 and 5.6 mg cm−2. To assemble the full cell, the negative electrode and positive electrode were placed face-to-face into a container in which 2 M KOH was added as the electrolyte.
2.3. Physical characterization
X-ray Diffraction (XRD) was carried out to identify the crystal structures on a Panalytical X'Pert Pro Multi-Purpose Diffractometer (MPD) with Cu Kalpha1 radiation working at 45 kV and 40 mA. Scanning electron microscopy (SEM) (ZEISS SUPRA 55-VP) and transmission electron microscopy (TEM) (JEOL 2100) were applied to observe the morphologies. Energy-dispersive X-ray spectroscopy (EDX) attached to the SEM was employed to analyze the elemental compositions.
2.4. Electrochemical measurements
The electrochemical properties of the as-prepared Cu2O/CuMoO4 and AC electrodes were studied in a conventional three-electrode cell configuration. In particular, the as-obtained electrode (Cu2O/CuMoO4 or AC) was used as the working electrode. The working electrode was soaked in 2 M KOH for 2 days and activated for 1000 cyclic voltammetry (CV) loops before the test. A piece of Pt mesh (1 × 1 cm2) and an Ag/AgCl electrode (sat. KCl) were used as counter and reference electrodes, respectively. The electrochemical performances of the asymmetric supercapacitor were explored in a two-electrode mode in which Cu2O/CuMoO4 acted as the positive electrode while the AC electrode was used as the negative electrode. All the measurements were performed in 2 M KOH solution at room temperature and recorded on a Solartron 1470E multichannel cell test system. The a.c. impedance was recorded at open circuit voltage by using an integrated Solartron 1455 frequency response analyzer at 5 mV bias with a frequency range of 100 kHz to 0.01 Hz.
2.5. Calculations
The specific capacitance of the electrode in three-electrode mode was calculated from the galvanostatic charge–discharge curves according to the following equation:19,36 | 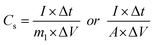 | (1) |
where Cs is the specific capacitance (F g−1 or F cm−2), I is the discharge current (A), Δt is the discharge time (s), m is the mass of the active materials on the Ni foam (g), ΔV is the potential change excluding the IR drop in the discharge step (V), and A is the geometric area of the electrode (cm2). In terms of the specific capacitance of the asymmetric supercapacitor, it was also calculated from the galvanostatic charge–discharge curves with the same formula:37,38 |  | (2) |
where m2 is the total mass of the active materials in positive and negative electrodes (g).
The energy densities and power densities of the asymmetric supercapacitor were obtained by the following equations:37,38
| 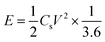 | (3) |
|  | (4) |
where
E is the energy density (W h kg
−1),
Cs is the specific capacitance (F g
−1),
V is the capacitor potential window excluding the IR drop (V),
P is the power density (W kg
−1) and Δ
t is the discharge time (s).
3. Results and discussion
3.1. Physical characterization of the electrode
Fig. 1a–d show the scanning electron microscopy (SEM) images of the Cu2O/CuMoO4 supported on Ni foam at different magnifications. The Ni foam shows a nice 3D porous structure (Fig. S1, ESI†) and its surface is flat and clean after acid treatment (Fig. S1b and c, ESI†). However, the images at low magnification (Fig. 1a and b) indicate that the Ni foam surface becomes rough after the synthesis procedure as plenty of materials are deposited on it, which proves that the applied hydrothermal process can facilitate growing materials on the substrate. From the enlarged pictures (Fig. S1c and d†), it can be seen that nanosheet clusters are uniformly distributed on the surface forming a grass-like morphology. Moreover, the nanosheets are interconnected with each other ultimately producing porous texture. Thus, a large surface area is generated and most of the nanosheet surface could be easily accessed by the electrolyte during the electrochemical test. It should be noted that the electrode was washed and calcined after the hydrothermal process; the overall nanostructure is still well defined, which indicates excellent stability. Fig. S2 (ESI†) shows the surface structures of Cu2O grown on Ni foam when only Cu(NO3)2 was used as the precursor solution. Obviously the surface is covered by grain shaped crystals with the size of around 10 μm. The difference in microstructures between the two samples reveals that the addition of MoO42− can significantly affect the growth process, facilitating the formation of nanosheets.
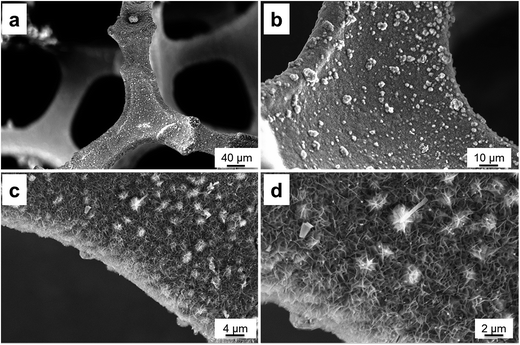 |
| Fig. 1 Typical SEM images of Cu2O/CuMoO4 nanosheets on Ni foam at different magnifications. | |
To identify the elemental compositions of the materials grown on Ni foam, energy-dispersive X-ray spectroscopy (EDX) analysis has been conducted on the electrode and the results are shown in Fig. S3 and S4 (ESI†). The EDX spectrum indicates that the nanosheets are composed of Ni, Cu, Mo, and O elements (Fig. S3a, ESI†), which is also confirmed by the EDX mapping (Fig. S3c–f, ESI†). Besides the Ni signal derived from Ni foam, the results are consistent with those of the raw materials used in the hydrothermal reaction.
The compositions of the grown materials are also identified by X-ray diffraction (XRD). No impurities are detected on the Ni foam substrate except the Ni peaks (Fig. S5a, ESI†). As shown in Fig. 2, the background of the Ni foam is very strong but the diffraction patterns still reveal that the composition of the grown materials is the hybrid of Cu2O, CuMoO4 and Cu as the remaining peaks can be assigned to the standard Cu2O (ICDD 04-013-0188), CuMoO4 (ICD 00-047-0511) and Cu (ICDD: 00-004-0850). In terms of the sample prepared with only Cu(NO3)2 as precursor solution (Fig. S5b, ESI†), Cu2O and Cu were detected. A peak at 2θ ≈ 27.1° was not identified, which needs further investigation. The CuMoO4 phase was not well crystallised, and its existence is further confirmed by transmission electron microscopy (TEM) (Fig. 3). The high-resolution TEM (HRTEM) images with lattice fringes are presented in Fig. 3a and b, and the corresponding Fourier transform patterns (inset of Fig. 3b) demonstrate the interplanar distance around 0.457, 0.379, 0.399, 0.408 and 0.512 nm, which can be indexed to the (110), (201), (
02), (002) and (1
1) planes of CuMoO4. It can be deduced that during the hydrothermal process, a portion of Cu2+ ions are converted into Cu2O and Cu, while the addition of MoO42− ions can simultaneously produce CuMoO4. It is known that Cu would be electro-oxidized to Cu2O in the KOH electrolyte by employing cyclic voltammetry (CV);31,38 therefore the electrolyte accessible Cu on the Ni foam is converted to Cu2O after the activation step while the remaining Cu can function as a part of the current collector to improve the electrode conductivity. The active materials here are therefore denoted as Cu2O/CuMoO4.
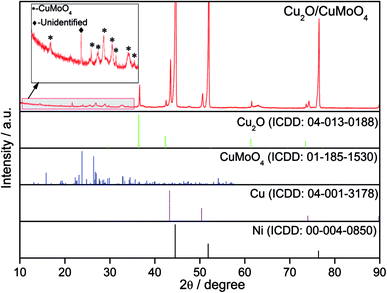 |
| Fig. 2 XRD patterns of the as-synthesized electrode. | |
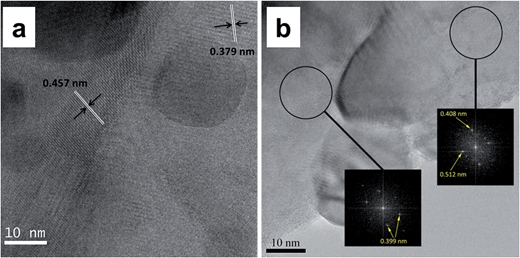 |
| Fig. 3 (a and b) TEM images of Cu2O/CuMoO4 nanosheets and the corresponding Fourier transform patterns (inset of b). | |
3.2. Electrochemical performances of the hybrid Cu2O/CuMoO4 nanosheet electrode
To evaluate the electrochemical performances of the Cu2O/CuMoO4 nanosheet electrode, the synthesized electrode was measured in a three-electrode cell configuration in 2 M KOH aqueous solution. It should be noted that the Ni foam substrate contributes negligible capacitance under the conditions in this work. The CV curves of the bare Ni foam electrode (1 × 1 cm2, calculated as 1 mg), Cu2O grown on Ni foam and hybrid Cu2O/CuMoO4 nanosheet electrode at 1 mV s−1 are compared in Fig. S6 (ESI†), it is obvious that the current density on the bare Ni foam and Cu2O electrodes are far lower than it on the Cu2O/CuMoO4 nanosheet electrode. The CV curves of Cu2O/CuMoO4 at different scan rates from 1 to 20 mV s−1 are shown in Fig. 4a. The appearance of distinct redox peaks between 0 and 0.6 V vs. Ag/AgCl confirms the pseudocapacitive behaviour of the materials and implies good electrochemical reversibility. Obviously, the current density increases with increasing scan rate, while the anodic and cathodic peaks slightly shift towards the more positive and negative potentials respectively, which is supposed to be related to the internal resistance of the electrode38 and limitation of charge transfer kinetics.27Fig. 4b shows the galvanostatic charge–discharge curves at various current densities from 1 to 50 A g−1. The nonlinear charge–discharge profiles further support the pseudocapacitive characteristics of the electrode, which is in good agreement with the CV curves as shown in Fig. 4a. Based on the discharge curves, the corresponding specific and areal capacitances are calculated (Fig. 4c). The specific capacitance is 4264 F g−1 (9.38 F cm−2) at a current density of 1 A g−1, and it decreases to 3121 F g−1 (6.87 F cm−2) when the current density increases to 10 A g−1. To the best of our knowledge, the specific capacitance of 4264 F g−1 is the highest in reported studies although the areal capacitance of 9.38 F g−1 is slightly lower than the highest value of 16 F cm−2 when Ni(OH)2/carbon nanotubes on Ni foam was used as the electrode.39 It is believed that the degradation of capacitance with the increase of the current densities is mainly due to the incremental IR drop and insufficient active material involved in redox reactions at higher current densities.27 However, as the current density keeps increasing, the electrode exhibits remarkable rate capability, namely, the capacitance still remains at 2563 F g−1 (5.64 F cm−2) at a current density as high as 50 A g−1. The capacitance values presented here are much higher than those reported in previous studies using similar materials: Cu2O/Cu nanoneedle arrays (510.2 F g−1, 0.88 F cm−2 at 2.9 A g−1);38 mesoporous NiO nanosheets (2504.3 F g−1, 0.376 F cm−2 at 13.4 A g−1);37 NiMoO4 nanowires (3298 F g−1, 4.94 F cm−2 at 5.33 A g−1);27 MnCo2O4/Ni(OH)2 core–shell nanoflowers (2124 F g−1, 3.19 F cm−2 at 5 A g−1);19 NiCoO2 (508 F g−1 at 0.5 A g−1).40 To further investigate the electrochemical behavior of the Cu2O/CuMoO4 electrode, electrochemical impedance spectroscopy (EIS) measurement is carried out in the frequency range from 100 kHz to 0.01 Hz at open circuit potential with the amplitude at 5 mV. As shown in Fig. 4d, the Nyquist plots of the Cu2O/CuMoO4 electrode are composed of a semicircle in the high-frequency region and a linear component in the low-frequency region. The intercept of the plots at the real axis represents the equivalent series resistance (Rs), including the ionic resistance of the electrolyte, intrinsic resistance of active materials and the contact resistance between the active materials and current collector,41–43 which is determined to be 0.334 Ω (inset of Fig. 4d). In terms of the semicircle, its diameter indicates the charge transfer resistance (Rct) of the system.41–43 The corresponding Rct of the Cu2O/CuMoO4 electrode is only 0.02 Ω, implying the easy and rapid charge transfer on the electrode. In the low-frequency region, the straight line presents the Warburg impedance (Zw),41–43 associated with the diffusion of the electrolyte ions along the Cu2O/CuMoO4 nanosheets. The large slope of the straight line shown in Fig. 4d demonstrates a very small Zw of the electrolyte ion diffusion. The small values of Rs, Rct and Zw suggest that there is a large electro-active surface area and higher electrical conductivity with the Cu2O/CuMoO4 nanosheet, which could extend the reaction zone of the electrode, making more electrode materials available for the charging/discharging process, leading to high capacitance.
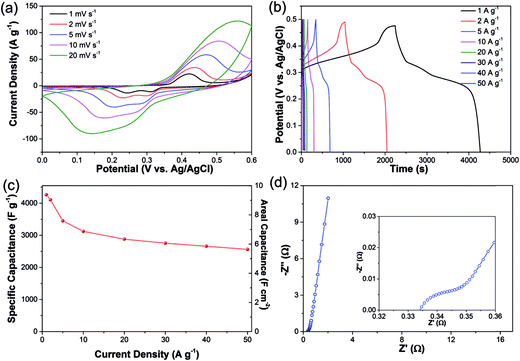 |
| Fig. 4 (a) CV curves of the Cu2O/CuMoO4 nanosheet electrode at different scan rates. (b) Galvanostatic charge–discharge curves of the Cu2O/CuMoO4 electrode at various current densities. (c) Specific and areal capacitances at various current densities. (d) Nyquist plots of the Cu2O/CuMoO4 electrode recorded at open circuit potential in the frequency range of 100 kHz to 0.01 Hz. The inset of (d) shows the impedance in the high-frequency region. | |
The dramatic high capacitance and outstanding rate capability of the Cu2O/CuMoO4 hybrid electrode can be attributed to its synthesis technique, nanostructure, and the possible synergistic effect from Cu2O and CuMoO4. The active materials are directly grown on the Ni foam current collector without any binders and conductive additives, which greatly reduces the contact resistance and improves the electrical conductivity of each nanosheet, leading to excellent conductivity of the electrode. Additionally, the specific surface area is significantly expanded with the nanosheets, which not only increases the electrolyte/electrode contact areas but also creates more active sites for rapid redox reactions with the anions and water molecules, enhancing the utilization of the Cu2O/CuMoO4. Moreover, the nanostructure could effectively facilitate the transport of electrolyte ions and electrons, and simultaneously, it is compatible with larger volume changes during the fast surface-dependent faradaic processes.
3.3. Electrochemical performances of the Cu2O/CuMoO4//AC asymmetric supercapacitor
An asymmetric supercapacitor was assembled using the prepared Cu2O/CuMoO4 electrode as the positive electrode and the AC electrode as the negative electrode for further investigating the performances of the Cu2O/CuMoO4 nanosheet electrode under practical conditions. The electrochemical performance of AC presents a typical feature of carbon-based materials (see Fig. S7, ESI†). In order to find the optimal working potential, a series of CV measurements in different voltage windows were conducted at 5 mV s−1. As seen from Fig. 5a, the operating windows are stable from 1.0 to 1.7 V. When it reaches 1.8 V, the polarization trend becomes very obvious, which means that severe water electrolysis happens. Therefore the best operating voltage window is determined to be 1.7 V. Fig. 5b presents the CV performances of the asymmetric supercapacitor at different scan rates ranging from 1 to 20 mV s−1. Unlike the single Cu2O/CuMoO4 or AC electrode, the asymmetric supercapacitor shows quasi-rectangular CV curves in all scans, which means that the capacitance is derived from both electric double-layer capacitance and pseudocapacitance. The operation voltage of 1.7 V here should be dependent on this remarkable synergy effect. The galvanostatic charge–discharge curves of the asymmetric supercapacitor were recorded at the current densities from 0.5 to 20 A g−1 (Fig. 5c). It can be seen that the discharge curves are nonlinear especially at low current densities, indicating the double contribution of electric double-layer capacitance and pseudocapacitance, which is consistent with the CV results. Based on the total mass of the positive and negative electrodes, the specific capacitance at various current densities is plotted in Fig. 5d. The calculated capacitances are 191, 156, 114, 92, 83.7, 79.8 and 75.1 F g−1 at current densities of 0.5, 1, 2, 5, 10, 15 and 20 A g−1, respectively. It can be found that the as-fabricated asymmetric supercapacitor reveals excellent rate capability from 5 to 20 A g−1. To further investigate the durability of the asymmetric supercapacitor, its cycling performance was recorded at 5 A g−1 (Fig. 5e). The specific capacitance still retains 86.6% of the initial value even after 3000 cycles, which demonstrates excellent electrochemical stability. It is believed the decline of the capacitance is caused by the destruction of the electrode film under numerous rapid redox reactions.19 This is also evidenced by first and last ten charge–discharge curves (Fig. S8, ESI†).
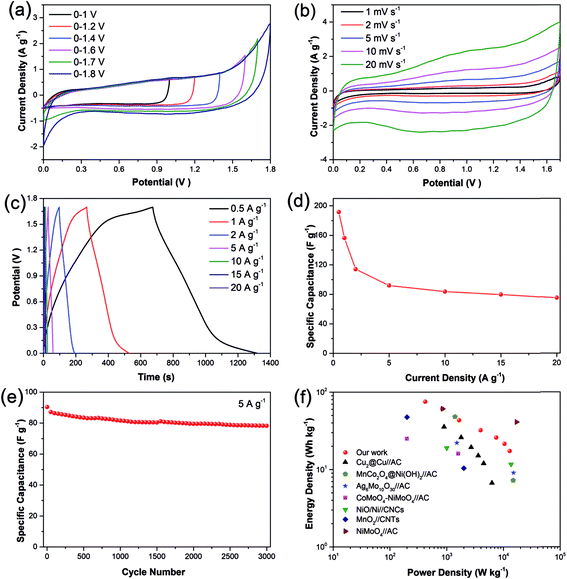 |
| Fig. 5 Electrochemical properties of the Cu2O/CuMoO4//AC asymmetric supercapacitor: (a) CV curves in various voltage windows at 5 mV s−1; (b) CV curves with a voltage window of 0–1.7 V at various scan rates; (c) galvanostatic charge–discharge curves at various current densities; (d) specific capacitance at various current densities. (e) Cycling performances of the asymmetric supercapacitor for 3000 cycles at 5 A g−1; (f) Ragone plots of our supercapacitor, compared with some other supercapacitors reported previously (CNCs: carbon nanocages and CNTs: carbon nanotubes). | |
The Ragone plots of the asymmetric supercapacitor derived from the discharge curve based on eqn (3) and (4) are displayed in Fig. 5f. The maximum energy density can be determined to be 75.1 W h kg−1 at the average power density of 420 W kg−1. It can still maintain 21.5 W h kg−1 even at a high power density of 10
435 W kg−1. It is found that the performance of the Cu2O/CuMoO4//AC asymmetric supercapacitor is better than that of many previously reported systems, such as Cu2O@Cu//AC,38 MnCo2O4@Ni(OH)2//AC,19 Ag6Mo10O30//AC,44 CoMoO4–NiMoO4//AC,23 NiO/Ni//CNCs45 and MnO2//CNTs46 but a higher energy density at 41.1 W h kg−1 was achieved with the power density of 17
002 W kg−1 by a NiMoO4//AC system.20
To further probe the stability of the hybrid electrode, the sample after the stability test was observed by SEM. Fig. 6 presents the SEM images after 3000 charge–discharge cycles at 5 A g−1; it can be clearly seen that the physical microstructures of the electrode maintain very well, which are still in nanosheets. As no notable changes can be found on the electrode before and after the cycling measurements, it proves the good stability of the hybrid electrode.
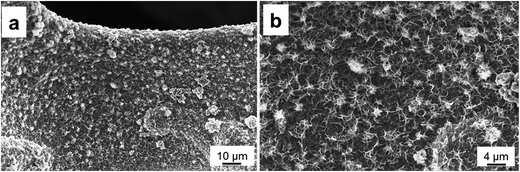 |
| Fig. 6 SEM images of the Cu2O/CuMoO4 nanosheet electrode after charge–discharge for 3000 cycles at a current density of 5 A g−1. | |
4. Conclusions
In summary, we synthesized a hybrid Cu2O/CuMoO4 nanosheet electrode via a facile hydrothermal method and it exhibits remarkably improved specific and areal capacitance (up to 4264 F g−1 and 9.38 F cm−2 at a current density of 1 A g−1). To the best of our knowledge, the specific capacitance of 4264 F g−1 is the highest in reported studies although the areal capacitance of 9.38 F g−1 is slightly lower than the highest value of 16 F cm−2 when Ni(OH)2/carbon nanotubes on Ni foam was used as the electrode. In addition, the electrode presents good rate capability; specifically, the capacitance still retains 2563 F g−1 (5.64 F cm−2) at a current density as high as 50 A g−1. Moreover, an asymmetric supercapacitor is fabricated using the Cu2O/CuMoO4 nanosheet electrode as the positive electrode and AC as the negative electrode. The operation voltage of the asymmetric supercapacitor could achieve 1.7 V, at which the energy density is 75.1 W h kg−1 with a power density of 420 W kg−1. The asymmetric supercapacitor also displays excellent rate capability and cycling stability. The capacitance remains around 86.6% after 3000 cycles at a current density of 5 A g−1. Considering the fascinating performances of Cu2O/CuMoO4 nanosheet electrodes and easy fabrication procedures, the electrodes are promising for applications in renewable energy storage.
Acknowledgements
One of the authors (Du) thanks the University of Warwick for a PhD studentship.
References
- J. Bae, M. K. Song, Y. J. Park, J. M. Kim, M. Liu and Z. L. Wang, Angew. Chem., Int. Ed., 2011, 50, 1683–1687 CrossRef CAS PubMed.
- N. Yu, H. Yin, W. Zhang, Y. Liu, Z. Tang and M. Q. Zhu, Adv. Energy Mater., 2016, 6 DOI:10.1002/aenm.201501458.
- J. Wu, X. Gao, H. Yu, T. Ding, Y. Yan, B. Yao, X. Yao, D. Chen, M. Liu and L. Huang, Adv. Funct. Mater., 2016, 26, 6114–6120 CrossRef CAS.
- G. Wang, L. Zhang and J. Zhang, Chem. Soc. Rev., 2012, 41, 797–828 RSC.
- J. R. Miller and P. Simon, Science, 2008, 321, 651–652 CrossRef CAS PubMed.
- J. Liu, L. Zhang, H. B. Wu, J. Lin, Z. Shen and X. W. D. Lou, Energy Environ. Sci., 2014, 7, 3709–3719 CAS.
- Y. Zhu, S. Murali, M. D. Stoller, K. Ganesh, W. Cai, P. J. Ferreira, A. Pirkle, R. M. Wallace, K. A. Cychosz and M. Thommes, Science, 2011, 332, 1537–1541 CrossRef CAS PubMed.
- P. Chen, J.-J. Yang, S.-S. Li, Z. Wang, T.-Y. Xiao, Y.-H. Qian and S.-H. Yu, Nano Energy, 2013, 2, 249–256 CrossRef CAS.
- J.-S. M. Lee, T.-H. Wu, B. M. Alston, M. E. Briggs, T. Hasell, C.-C. Hu and A. I. Cooper, J. Mater. Chem. A, 2016, 4, 7665–7673 CAS.
- P. Simon, Y. Gogotsi and B. Dunn, Science, 2014, 343, 1210–1211 CrossRef CAS PubMed.
- Z. Yu, L. Tetard, L. Zhai and J. Thomas, Energy Environ. Sci., 2015, 8, 702–730 CAS.
- Z.-S. Wu, W. Ren, D.-W. Wang, F. Li, B. Liu and H.-M. Cheng, ACS Nano, 2010, 4, 5835–5842 CrossRef CAS PubMed.
- Y.-Z. Su, K. Xiao, N. Li, Z.-Q. Liu and S.-Z. Qiao, J. Mater. Chem. A, 2014, 2, 13845–13853 CAS.
- Z. Lu, Z. Chang, W. Zhu and X. Sun, Chem. Commun., 2011, 47, 9651–9653 RSC.
- T. Brezesinski, J. Wang, S. H. Tolbert and B. Dunn, Nat. Mater., 2010, 9, 146–151 CrossRef CAS PubMed.
- M. Huang, Y. Zhang, F. Li, L. Zhang, Z. Wen and Q. Liu, J. Power Sources, 2014, 252, 98–106 CrossRef CAS.
- L. Yu, B. Guan, W. Xiao and X. W. D. Lou, Adv. Energy Mater., 2015, 5 DOI:10.1002/aenm.201500981.
- L. Bao, J. Zang and X. Li, Nano Lett., 2011, 11, 1215–1220 CrossRef CAS PubMed.
- Y. Zhao, L. Hu, S. Zhao and L. Wu, Adv. Funct. Mater., 2016, 26, 4085–4093 CrossRef CAS.
- S. Peng, L. Li, H. B. Wu, S. Madhavi and X. W. D. Lou, Adv. Energy Mater., 2015, 5 DOI:10.1002/aenm.201401172.
- D. Cai, D. Wang, B. Liu, L. Wang, Y. Liu, H. Li, Y. Wang, Q. Li and T. Wang, ACS Appl. Mater. Interfaces, 2014, 6, 5050–5055 CAS.
- L. Huang, D. Chen, Y. Ding, Z. L. Wang, Z. Zeng and M. Liu, ACS Appl. Mater. Interfaces, 2013, 5, 11159–11162 CAS.
- M.-C. Liu, L.-B. Kong, C. Lu, X.-J. Ma, X.-M. Li, Y.-C. Luo and L. Kang, J. Mater. Chem. A, 2013, 1, 1380–1387 CAS.
- X. Xiong, D. Ding, D. Chen, G. Waller, Y. Bu, Z. Wang and M. Liu, Nano Energy, 2015, 11, 154–161 CrossRef CAS.
- H. Chen, L. Hu, M. Chen, Y. Yan and L. Wu, Adv. Funct. Mater., 2014, 24, 934–942 CrossRef CAS.
- Y. Cheng, H. Zhang, C. V. Varanasi and J. Liu, Energy Environ. Sci., 2013, 6, 3314–3321 CAS.
- D. Guo, P. Zhang, H. Zhang, X. Yu, J. Zhu, Q. Li and T. Wang, J. Mater. Chem. A, 2013, 1, 9024–9027 CAS.
- M. Yu, J. Chen, J. Liu, S. Li, Y. Ma, J. Zhang and J. An, Electrochim. Acta, 2015, 151, 99–108 CrossRef CAS.
- X. Yu, B. Lu and Z. Xu, Adv. Mater., 2014, 26, 1044–1051 CrossRef CAS PubMed.
- J. Jiang, J. Liu, W. Zhou, J. Zhu, X. Huang, X. Qi, H. Zhang and T. Yu, Energy Environ. Sci., 2011, 4, 5000–5007 CAS.
- C. Dong, Q. Bai, G. Cheng, B. Zhao, H. Wang, Y. Gao and Z. Zhang, RSC Adv., 2015, 5, 6207–6214 RSC.
- A. Pendashteh, M. F. Mousavi and M. S. Rahmanifar, Electrochim. Acta, 2013, 88, 347–357 CrossRef CAS.
- K. Wang, C. Zhao, S. Min and X. Qian, Electrochim. Acta, 2015, 165, 314–322 CrossRef CAS.
- S. K. Shinde, D. P. Dubal, G. S. Ghodake and V. Fulari, RSC Adv., 2015, 5, 4443–4447 RSC.
- G. Wang, J. Huang, S. Chen, Y. Gao and D. Cao, J. Power Sources, 2011, 196, 5756–5760 CrossRef CAS.
- Q. Lu, M. W. Lattanzi, Y. Chen, X. Kou, W. Li, X. Fan, K. M. Unruh, J. G. Chen and J. Q. Xiao, Angew. Chem., 2011, 123, 6979–6982 CrossRef.
- G. Cheng, W. Yang, C. Dong, T. Kou, Q. Bai, H. Wang and Z. Zhang, J. Mater. Chem. A, 2015, 3, 17469–17478 CAS.
- C. Dong, Y. Wang, J. Xu, G. Cheng, W. Yang, T. Kou, Z. Zhang and Y. Ding, J. Mater. Chem. A, 2014, 2, 18229–18235 CAS.
- Z. Tang, C. H. Tang and H. Gong, Adv. Funct. Mater., 2012, 22, 1272–1278 CrossRef CAS.
- Y. Xu, J. Wei, L. Tan, J. Yu and Y. Chen, J. Mater. Chem. A, 2015, 3, 7121–7131 CAS.
- X. Zhang, Y. Zhao and C. Xu, Nanoscale, 2014, 6, 3638–3646 RSC.
- S. E. Moosavifard, J. Shamsi, S. Fani and S. Kadkhodazade, RSC Adv., 2014, 4, 52555–52561 RSC.
- M.-C. Liu, L. Kang, L.-B. Kong, C. Lu, X.-J. Ma, X.-M. Li and Y.-C. Luo, RSC Adv., 2013, 3, 6472–6478 RSC.
- V. Kumar, S. Matz, D. Hoogestraat, V. Bhavanasi, K. Parida, K. Al-Shamery and P. S. Lee, Adv. Mater., 2016, 28, 6966–6975 CrossRef CAS PubMed.
- H. Lai, Q. Wu, J. Zhao, L. Shang, H. Li, R. Che, Z. Lyu, J. Xiong, L. Yang and X. Wang, Energy Environ. Sci., 2016, 9, 2053–2060 CAS.
- H. Jiang, C. Li, T. Sun and J. Ma, Nanoscale, 2012, 4, 807–812 RSC.
Footnote |
† Electronic supplementary information (ESI) available: SEM image, EDS analysis, CV and charge/discharge curves and other additional supporting data. See DOI: 10.1039/c6ta08670e |
|
This journal is © The Royal Society of Chemistry 2016 |