SFX as a low-cost ‘Spiro’ hole-transport material for efficient perovskite solar cells†
Received
5th January 2016
, Accepted 29th February 2016
First published on 2nd March 2016
Abstract
Four spiro[fluorene-9,9′-xanthene] (SFX) derivatives, SFX-TAD, SFX-TCz, SFX-TPTZ and SFX-MeOTAD have been synthesized for use as hole-transport materials and fully characterized by 1H/13C NMR spectroscopy, mass spectrometry, XRD and DSC. Their thermal, optical and electrochemical properties were investigated. The use of different substituents affects the highest occupied molecular orbital (HOMO) energy level proving the versatility of the central core towards the facile and low-cost preparation of spiro-hole-transport materials. Among the synthesized SFX derivatives, SFX-MeOTAD was employed as hole-transporting material (HTM) and the performance tested in perovskite solar cells with device architecture glass/FTO/compact TiO2/mesoporous Al2O3/CH3NH3PbI3−xClx/HTM/Au. SFX-MeOTAD gave a comparable efficiency to spiro-MeOTAD, demonstrating great potential for use of the SFX family as cost effective and versatile hole conductors facilitating commercialization of perovskite solar cells (PSCs).
1 Introduction
Lead halide perovskite was first introduced to photovoltaics as a sensitizer in dye-sensitized solar cells by Miyasaka.1 This material type has since attracted huge attention due to outstanding properties including high extinction coefficient, small exciton binding energy, long charge carrier diffusion length and lifetime. In consequence, 21% certified efficiency by EPFL has been recently achieved.2 The most common structure of perovskite solar cells (PSCs) includes spiro-MeOTAD as a hole conductor but its high price and tedious multistep synthesis hinders progress of this technology towards commercialization. There is therefore a requirement for alternative hole transporting materials (HTMs), which should fulfil the following demands: matching the energy levels of the active layer, forming smooth and stable films, good solubility for solution-processed devices, low-cost production and high hole conductivity.
Many small molecules, polymers and inorganic salts have been explored as HTMs but small molecules tend to be more attractive due to ease of purification, high purity and smaller batch-to-batch variation. The most recent reports on high efficiencies of PSCs with small organic molecules as hole-transporting materials include structures like: methoxydiphenylamine-substituted carbazole (V886),3 triazatruxene (TPDI),4 (KR131),5 [2,2]paracyclophane triarylamine (PCP-TPA),6 azomethine7 and diacetylide-triphenylamines (DATPA).8 Efficiencies comparable with spiro-MeOTAD have been shown and also improvement of thermal stability, simpler preparation methods and total cost of synthesis. In nearly all these cases however, there has been no development of a wider family of materials with differing redox potential, solubility or thermal properties, although this is likely to prove important to enable better-matched combination with differing perovskite materials.
Most of the reported small molecule based HTM materials have a nonplanar 3D architecture, which can reduce electronic coupling and charge recombination,9 provide high thermal stability, high glass transition temperature and favourable solubility. One of the key methods to achieve such properties is the utilization of a central spiro carbon, as demonstrated by the ubiquitous spiro-MeOTAD where two fluorenes are orthogonally interconnected. To overcome the high cost and complexity of spiro-bifluorene synthesis however, in the present study we have focussed our interest on spiro[fluorene-9,9′-xanthene] SFX due to its easy synthesis which was reported as an “Unexpected One-Pot Method…”.10 This core has attracted a lot of attention recently as a starting material for “eco-friendly green organic semiconductors”11 but work has so far been limited mainly to light emitting diodes (OLEDs).12–16 Surprisingly, among the reported structures and their high complexity, no tetraamino substituted derivatives have been described. An additional motivation for our interest in the target molecule was a study by Koene et al.17 showing thermal stability enhancement of asymmetric triaryldiamines for hole transporting layer in OLEDs over the symmetric ones with maintenance, or improvement, of other desired properties.
Herein, we report the facile synthesis of four new SFX-based hole conductors, as shown in Fig. 1, with a variety of HOMO energy levels to achieve redox tuning. Among them, we have so far studied SFX-MeOTAD in detail for application in perovskite solar cells. This material shows high glass transition temperature, high solubility, purely amorphous state and HOMO level alignment almost identical to spiro-MeOTAD. We show that devices employing SFX-MeOTAD show high power conversion efficiency up to 12.4%, compared with 13.0% for spiro-MeOTAD, but with the significant advantage of more than 5 times lower cost of synthesis. Furthermore, the easy synthesis of this series has enabled us to prepare examples of varying redox potential, offering a choice of HTM tailored to other emerging perovskite solar cell materials.
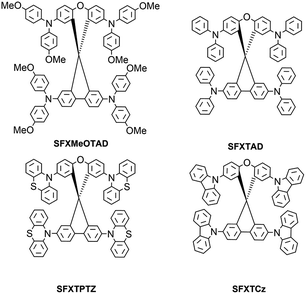 |
| Fig. 1 Chemical structures of SFX based hole conductors. | |
2 Results and discussion
2.1 Synthetic procedures
The synthetic procedure for the preparation of SFX derivatives is shown in Fig. 2. The key step is the synthesis of the central core spiro[fluorene-9,9′-xanthene] (SFX). In contrast to 9,9′-spirobifluorene (SBF), where only multistep protocols were reported18–21 involving Grignard reaction demanding dry solvents, the procedure for SFX is a one-pot, non-solvent synthesis which was reported previously.10 Moreover, reagents (phenol and methanesulfonic acid) are more than 30 times cheaper than 2-bromobiphenyl or 2-iodobiphenyl used in SBF synthesis. Scaling up the reaction to produce about 50 g of SFX only slightly decreased reaction yield from 80% to 77% even though the column purification was replaced by a silica plug followed by recrystallization from ethanol/methanol. Tetrabromination was conducted in excess bromine with high yield of 75% at the scale of 40 g of starting material. The only purification used was a silica plug followed by recrystallization from chloroform/ethanol mixture. Various molecular designs of the new hole conductors based on carbazole, diphenylamine, 4,4′-dimethoxydiphenylamine and phenothiazine motifs were synthesized by the Buchwald–Hartwig amination reaction between 2,2′,7,7′-tetrabromo-spiro(fluorene-9,9′-xanthene) and the respective amine. Final products were isolated with 71%, 56%, 35%, 83% yield for SFX-MeOTAD, SFX-TAD, SFX-TPTZ and SFX-TCz, respectively. Column chromatography was used for all final product purifications except SFX-TPTZ where crystallization from toluene/chlorobenzene was applied. Additionally, two amination conditions were tested for SFX-MeOTAD and the more economic tri(o-tolyl)phosphine and Pd2(dba)3 instead of tri-tert-butylphosphonium tetrafluoroborate and Pd(OAc)2 gave similar reaction yield of about 60% at small scale. Scaling up this reaction with the use of palladium(II) acetate as a catalyst to 7 g of starting SFXTBr increased the reaction yield to 71% and 2.568 g of 4,4′-dimethoxydiphenylamine were recovered. All of the analytical data (1H/13C NMR spectroscopy and mass spectrometry, see ESI†) support the proposed structures. Additionally, solubility of SFX-MeOTAD was found to be more than 300 mg mL−1, similar to spiro-MeOTAD22 but with much faster dissolution than spiro-MeOTAD. Full experimental details and characterization can be found in the experimental section.
 |
| Fig. 2 Synthetic route for SFX and its derivatives. | |
2.2 Cost analysis
As previously mentioned by Murray et al.,23 large-scale production of solar cells is hindered by the cost of spiro-MeOTAD. In particular, the SBF core and the Pd-catalysed coupling are the main influence on the final price of the material. In Table S1† it can be seen that the cost of materials for the synthesis of SBF, ranging from $35 to $44 per gram, is responsible for about half of the final cost of spiro-MeOTAD. Therefore, the introduction of SFX core as an inexpensive alternative (costing about $1 per gram) cuts the cost of the final material to half and decreases the number of steps from 6 for spiro-MeOTAD to 3 for SFX-MeOTAD (see Fig. S6†). Further optimization and scaling up the synthesis of SFX-MeOTAD gave a final cost of the new hole conductor more than five times lower (17$) [see Tables S2–4†] than the commonly used spiro-MeOTAD (from 92$ to 108$).7 Additionally, the availability of the new cost-effective core for hole transport materials that has similar electronic properties to SBF, can open up more diverse modification with other diphenylamine derivatives beyond 4,4′-dimethoxydiphenylamine23,24 that can benefit device stability or efficiency through tailoring hole-transport materials according to the energy levels of the chosen perovskite.
2.3 Optical and electrochemical properties
The UV-vis absorption spectra of the SFX series in dichloromethane (DCM) are presented in Fig. 3a. All derivatives show an absorption in the UV region. SFX-MeOTAD, SFX-TAD and SFX-TCz exhibit two absorption bands at 307, 367; 307, 379 and 291, 340 nm, respectively whereas SFX-TPTZ shows one broad absorption band with maximum at 310 nm. Photoluminescence spectra are depicted in Fig. 3b.
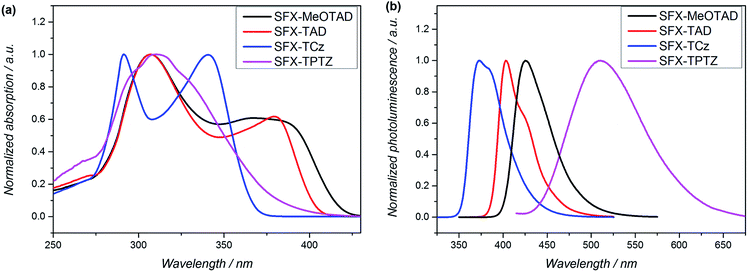 |
| Fig. 3 (a) Normalized UV-visible absorption in DCM at 2 × 10−5 mol mL−1. (b) Photoluminescence in DCM at 5 × 10−6 mol mL−1 of SFX series. | |
The maximum of the emission is red-shifted in the following order: SFX-TCz (372 nm), SFX-TAD (403 nm), SFX-MeOTAD (425 nm) and SFX-TPTZ (509 nm). Optical band gaps shown in Table 1 were estimated from the absorption onset. The maxima of absorption and emission of SFX-MeOTAD are similar to spiro-MeOTAD resulting in the same value of optical band gap for both materials. Stokes shifts were calculated from the difference between the maximum of absorption and emission spectra. The largest Stokes shift was observed for SFX-TPTZ (12
600 cm−1), indicating a greater geometric difference upon relaxation of the excited state compared with the ground state. Additionally, broad absorption and emission bands with planar type structure of the phenothiazine suggest formation of twisted intramolecular charge transfer (TICT) state as is observed for example for 9,9′-binathryl.25 On the other hand, small Stokes shift and narrow bands for SFX-TCz imply that this nonradiative channel is not accessible regardless of the planarity of carbazole and previously-reported26 TICT behaviour for this kind of structure.
Table 1 Electrochemical, photophysical and thermal properties
HTM |
λ
max (nm) |
λ
em
(nm) |
Stokes shiftb (cm−1) |
E
gap
(eV) |
E
ox
(V) |
E
HOMO
(eV) |
E
LUMO
(eV) |
T
g
(°C) |
T
m
(°C) |
Excitation at bold λmax.
The difference between the maximum of absorption and maximum of emissions spectra; in parenthesis is the difference between lower energy absorption bands and maximum of emission spectra.
From the absorption onset.
The half-wave potential from the CV and in parentheses the peak potential from the SWV measurements referenced to ferrocene.
E
HOMO (eV) = −5.1 − (Eox).37
E
LUMO = EHOMO + Egap.
Determined by differential scanning calorimetry (DSC).
|
Spiro-MeOTAD
|
301, 385 |
424 |
9640 (2390) |
2.98 (ref. 34) |
(+0.04) |
−5.14 (−5.15) (ref. 35) |
−2.16 (2.11) (ref. 35) |
122 (125) (ref. 36) |
248 (248) (ref. 36) |
SFX-MeOTAD
|
307, 367 |
425 |
9040 (3720) |
2.96 |
+0.07 (+0.05) |
−5.16 |
−2.20 |
108 |
237 |
SFX-TAD
|
307, 379 |
403 |
7760 (1570) |
3.07 |
+0.23 (+0.26) |
−5.34 |
−2.27 |
118 |
245 |
SFX-TCz
|
291, 340 |
372 |
7480 (2530) |
3.39 |
+0.60 |
−5.70 |
−2.31 |
— |
>270 |
SFX-TPTZ
|
310
|
509 |
12 600 |
3.25 |
+0.27 (+0.32) |
−5.39 |
−2.14 |
— |
>270 |
The highest occupied molecular orbital (HOMO) energy levels of the compounds were estimated from the half-wave potential using ferrocene/ferrocenium as an internal standard in cyclic voltammetry (Fig. 4a) and square-wave voltammetry (Fig. 4b) experiments. The first oxidation potential of SFX-MeOTAD was found to be almost the same as spiro-MeOTAD; therefore the energy of the HOMO levels of both materials are about −5.1 eV. Whereas, SFX-TAD, SFX-TCz and SFX-TPTZ have higher oxidation potentials thus lowering their HOMO levels to −5.34, −5.70 and −5.39 eV respectively.
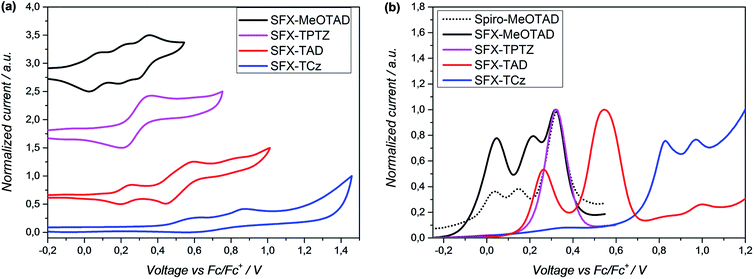 |
| Fig. 4 (a) Cyclic voltammograms (CV) and (b) square-wave voltammetry (SWV) traces of the SFX series and spiro-MeOTAD in DCM solution with supporting electrolyte 0.3 M tetrabutylammonium tetrafluoroborate referenced to ferrocene. | |
From the CV measurements, it can be noted that SFX-MeOTAD exhibits three reversible oxidation peaks and on the SWV graph the comparison to the ‘Spiro’ shows that first and third are at the same potential. Oxidation reversibility of all studied hole conductors was confirmed (see ESI, Fig. S1 and S2†). Overall these results indicate that the hole transfer from MAPbI3−xClx to the new HTM materials should be favourable, except for SFX-TCz. Moreover, the lower HOMO energy level for SFX-TAD and SFX-TPTZ could potentially lead to higher open circuit voltages of the operating devices. At present, poor solubility of these latter materials hinder their application in solar cells, and synthesis of more-soluble analogues is required to test this hypothesis. Table 1 summarizes the optical and electrochemical properties of the materials in comparison to the spiro-MeOTAD.
2.4 XRD analysis
The structures of the new materials have been confirmed conclusively by X-ray diffraction analysis of three of them as shown in Fig. S4.† The compounds were found to crystallize in triclinic space group P
(SFX-MeOTAD), monoclinic C2/c (SFX-TPTZ) and orthorhombic Pbca (SFX-TCz). In Fig. 5, we can see that SFX-MeOTAD forms three types of hydrogen bonds with lengths of 2.522 Å for CHO (xanthene), 2.549 Å and 2.572 Å for OMe–OMe. The dihedral angle between fluorene and xanthene units was measured to be 87.54°, 88.89° and 87.57° respectively for SFX-MeOTAD, SFX-TPTZ and SFX-TCz (see ESI, Fig. S7 and S8†). This is similar to the value found for spiro-MeOTAD (89.94°)27 which is the origin of low intermolecular interactions and therefore the formation of stable amorphous solids. Taking into account that SFX-MeOTAD has the same peripheral groups (i.e. 4,4′-dimethoxydiphenylamine) and crystallizes in P
space group as does spiro-MeOTAD, a similar amorphous state would be expected. On the other hand, for SFX-TPTZ π–π interactions with length of 3.756 Å and for SFX-TCz 3.868 Å and 3.752 Å were observed (Fig. S5†). Which is in agreement with higher melting points and lower solubility for both of materials indicating strong intermolecular interaction.
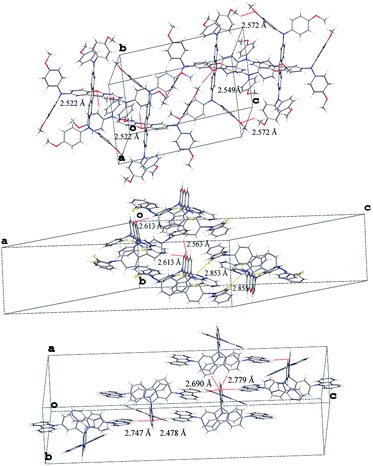 |
| Fig. 5 Crystal packing of SFX-MeOTAD, SFX-TPTZ and SFX-TCz. | |
The X-ray powder diffraction patterns on the bulk samples (as synthesized) were experimentally obtained and compared with the simulated ones from their single-crystal data (Fig. S9†). Importantly, the powder pattern for SFX-MeOTAD showed the presence of only the amorphous state whereas the spiro-MeOTAD pattern indicated some crystallinity in agreement with the simulated pattern. Greater crystallinity in the powder samples was observed for SFX-TPTZ and almost pure crystal form for SFX-TCz. Interestingly, the experimental data for SFX-TAD showed that the introduction of methoxy groups has an influence, as expected, on readily obtaining an amorphous state since some peaks were observed for the unsubstituted derivative. The summary of X-ray crystallographic data for SFX-MeOTAD, SFX-TPTZ and SFX-TCz is given in ESI Table S5.†
2.5 Thermal properties
The results from Differential Scanning Calorimetry (DSC) are shown in ESI (Fig. S3–5†). DSC data show glass transition temperature (Tg) of 108 °C, 122 °C and 118 °C for SFX-MeOTAD, spiro-MeOTAD and SFX-TAD, respectively. The three materials show cold crystallization: SFX-MeOTAD at 197 °C, spiro-MeOTAD at 176 °C and SFX-TAD at 178 °C. For SFX-TPTZ and SFX-TCz neither Tg nor melting point (Tm) up to 270 °C were observed. This may be associated with the strong intermolecular interactions observed by single-crystal X-ray diffraction and the high crystallinity seen in powder diffraction experiments.
Similarly to the powder diffraction results, better packing of SFX-TAD manifests in not only higher Tg but melting point as well, raising it from 237 °C for SFX-MeOTAD to 245 °C for SFX-TAD (although an additional smaller endothermic peak is observed at 221 °C). The experiment conducted on spiro-MeOTAD shows higher melting point of 248 °C. These results confirm the conclusion that SFX-MeOTAD forms stable amorphous glasses and has high thermal stability.
2.6 Solar cell studies
In order to investigate the performance of SFX-MeOTAD as hole transport material in perovskite solar cells, the following device architecture was adopted in the present study – glass/FTO/compact TiO2/meso Al2O3/CH3NH3Pb3−xClx/HTM (spiro-MeOTAD or SFX-MeOTAD)/Au. Fig. 6 shows the cross-sectional scanning electron micrograph of a typical device clearly showing the different layers present. To enable reliable comparison between SFX-MeOTAD and spiro-MeOTAD, all PSCs were fabricated in a single continuous study over 12 repeats for each HTM using identical steps and similar concentration of additives [LiTFSI [lithium bis(trifluoromethanesulfonyl)imide] and tBP (tert-butylpyridine)] optimised for the later. Fig. 7 shows the J–V characteristics of the champion devices recorded using 0.05 V s−1 from forward bias to short circuit condition. The table in the inset gives the corresponding photovoltaic parameters of the champion devices. The best cell efficiency of 12.4% was obtained using SFX-MeOTAD whereas 13.0% was observed for PSC using spiro-MeOTAD. The slightly different HOMO and the oxidation potential of SFX-MeOTAD as compared to spiro-OMeTAD results in slightly higher Voc and lower Jsc of the former as compared to the latter. The slightly lower FF on the other hand is related to the high series resistance which in this case would be due to the resistance to transport in the HTM due to slightly different hole conductivity (1.6 × 10−5 S cm−1 and 1.4 × 10−5 S cm−1 for spiro-MeOTAD and SFX-MeOTAD, respectively). The values agree well with the values reported for spiro-MeOTAD in the literature.28 The higher FF for spiro-MeOTAD may also arise from the use of a dopant regime optimised for that material.29Fig. 8 shows the box plots with the mean and standard deviation of the photovoltaic parameters of the devices measured under simulated AM1.5G sunlight at 100 mW cm−2 with J–V curves recorded using 0.05 V s−1 from forward bias to short circuit condition. It is interesting to note the high Jsc values observed for both SFX and spiro based HTMs. The high Jsc values could be possibly due to the reduced number of grain boundaries and low dimensional defects due to larger grains (clearly seen in the SEM micrograph in Fig. 6) and increased crystallinity of the perovskite layer due to the solvent annealing step.30 It is worth noting that the theoretical maximum achievable value for Jsc is 26 mA cm−2 assuming 100% internal quantum efficiency and 5% optical losses in the FTO.31 As expected, PSCs using SFX-MeOTAD showed comparable photovoltaics parameters with the mean and standard deviation PCE = 11.1 ± 0.8%, Jsc = 21.2 ± 2.4 mA cm−2, Voc = 0.96 ± 0.04 V, FF = 55.2 ± 3.9% as compared to those using spiro-MeOTAD with PCE = 11.3 ± 0.9%, Jsc = 21.9 ± 2.4 mA cm−2, Voc = 0.92 ± 0.02 V, FF = 56.4 ± 5.5%. It is important to note that these values correspond to the J–V curves recorded using 0.05 V s−1 from forward bias to short circuit condition. The devices exhibit hysteresis, very common in perovskite solar cells and the box plots with the mean and standard deviation of the average PCE (for the forward and reverse direction) of the devices measured under simulated AM1.5G sunlight at 100 mW cm−2 with J–V curves recorded using 0.05 V s−1 scan speed are shown in the Fig. S11.† PSCs using SFX-MeOTAD showed comparable average PCE with the mean and standard deviation PCE = 9.2 ± 0.9%, as compared to those using spiro-MeOTAD with average PCE = 9.5 ± 0.6%. The corresponding photovoltaics parameters for the scan direction from short circuit to forward bias condition are given in the ESI (Fig. S10†). The lower PCE values observed for the scan direction when measured from short circuit to forward bias condition are mainly due to the loss in the FF.
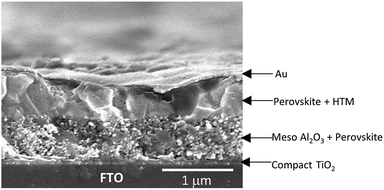 |
| Fig. 6 Cross-sectional SEM image of a typical CH3NH3PbI3−xClx based PSC. | |
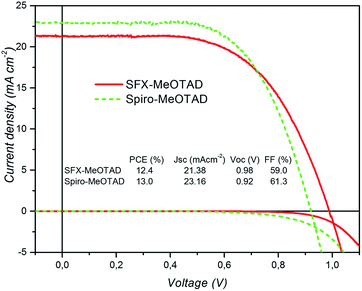 |
| Fig. 7
J–V curves (light and dark) of the champion PSCs with SFX-MeOTAD, and spiro-MeOTAD HTMS recorded using 0.05 V s−1 from forward bias to short circuit. | |
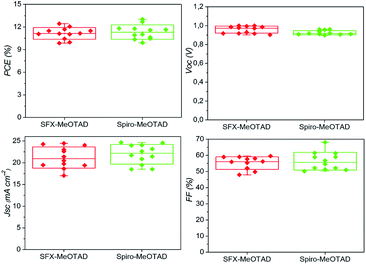 |
| Fig. 8 Box plot of photovoltaic parameters of PSCs with SFX-MeOTAD (left), and spiro-MeOTAD (right) HTMS recorded using 0.05 V s−1 from forward bias to short circuit. | |
3 Conclusions
In conclusion, we have synthesised four novel hole conductors using the SFX core for the first time in triarylamine-based materials. This approach significantly reduced the cost of the final material, namely SFX-MeOTAD, compared with the commonly-used analogue compound, spiro-MeOTAD. The synthesized materials have been characterized using spectroscopic methods, electrochemistry, single crystal and powder diffraction and differential scanning calorimetry. It has been shown that HOMO energy level can be easily modified but at present solubility in the case of SFX-TCz, SFX-TPTZ and SFX-TAD was too low to test these materials in the solar cell devices. Therefore, the material demonstrated for application in perovskite solar cells was SFX-MeOTAD, based on its high solubility, highly amorphous state and appropriate HOMO energy level alignment. Comparable tests of the devices' performance confirmed this choice showing a high efficiency of 12.4% for SFX-MeOTAD and 13.0% for the reference spiro-MeOTAD when measured from the forward bias to short circuit, with average efficiencies for these almost identical. Further investigations on SFX-based hole transporting materials are being continued in our laboratory mainly focused on highly processable derivatives with a variety of oxidation potentials. We are confident that this will lead to an improvement in the device efficiency and allow easy tailoring of HTMs to a particular device structure.
4 Experimental
4.1 Materials and synthesis
All reagents were purchased from either Sigma-Aldrich or AlfaAesar and they were used as received without further purification unless otherwise stated. All preparations were carried out using standard Schlenk line and air-sensitive chemistry techniques under nitrogen atmosphere. Toluene was dried using a solvent purification system. Column chromatography was carried out using Silica 60A (particle size 35–70 μm, Fisher, UK) as the stationary phase, and TLC was performed on pre-coated silica gel plates (0.25 mm thick, 60 F254, Merck, Germany) and observed under UV light.
4.2 Chemical characterization
NMR spectra were recorded on Bruker (400, 500 and 600 MHz) spectrometers for solutions in benzene-d6, CDCl3 and methylene chloride-d2. Chemical shifts are reported in parts per million. Chemical shift multiplicities are reported as s: singlet, d: doublet, t: triplet, q: quartet, quint: quintet, and m: multiplet. Mass spectra (EI, ESI) were recorded with Xevo QTOF (Waters) high resolution, accurate mass tandem mass spectrometer equipped with Atmospheric Solids Analysis Probe (ASAP) and Bruker MicroToF 2. Elemental analyses were carried out by Stephen Boyer of the Science Centre, London Metropolitan University using a Carlo Erba CE1108 Elemental Analyser.
4.3 Electrochemical characterization
All cyclic voltammetry measurements were carried out in freshly distilled CH2Cl2 using 0.3 M [TBA][BF4] electrolyte in a three-electrode system, with each solution being purged with N2 prior to measurement. The working electrode was a Pt disk. The reference electrode was Ag/AgCl and the counter electrode was a Pt rod. All measurements were made at room temperature using a mAUTOLAB Type III potentiostat, driven by the electrochemical software GPES. Cyclic voltammetry (CV) measurements used scan rates of 0.1 V s−1; square wave voltammetry (SWV) was carried out at a step potential of 0.004 V, square wave amplitude of 0.025 V, and a square wave frequency of 25 Hz (SFX-MeOTAD, SFX-TAD), 15 (SFX-TPTZ, spiro-MeOTAD) and 10 Hz (SFX-TCz), giving a scan rate of 0.04 V s−1. Ferrocene was used as the internal standard in each measurement.
4.4 Optical characterization
Solution UV-visible absorption spectra were recorded using a Jasco V-670 UV/vis/NIR spectrophotometer controlled with SpectraManager software. Photoluminescence (PL) spectra were recorded with a Fluoromax-3 fluorimeter controlled by the ISAMain software. All samples were measured in a 1 cm cell at room temperature with dichloromethane as solvent. Concentration of 2 × 10−5 and 5 × 10−6 mol mL−1 were used for solution UV/visible and PL, respectively.
4.5 Thermal characterization
Differential scanning calorimetry (DSC) was performed on NETZSCH STA 449F1 at a scan rate of 10 K min−1 under nitrogen atmosphere in DSC/TG aluminium pan. The measurement range was 25 °C to 270 °C.
4.6 Crystallographic details
Powder diffraction was performed on a Bruker Discover D8 with CuKα1/2 source and a scintillation detector.
Crystallographic data were collected using Agilent Technologies SuperNova with Cu Kα (l ¼1.54178 A) radiation at 120 K. Single colourless block-shaped crystals of SFX-MeOTAD were recrystallised from a mixture of DCM, methanol and acetone by solvent layering. Single colourless block-shaped crystals of SFX-TPTZ were recrystallised from a mixture of DCM and hexane by solvent layering. Single colourless prism-shaped crystals of SFX-TCz were recrystallised from a mixture of hexane and DCM by solvent layering. A suitable crystal of SFX-MeOTAD (0.40 × 0.08 × 0.03), SFX-TPTZ (0.41 × 0.09 × 0.04) and SFX-TCz (0.19 × 0.16 × 0.10) were selected and mounted on a MITIGEN holder in Paratone oil on a Rigaku Oxford Diffraction SuperNova diffractometer. The crystal was kept at T = 120.0 K during data collection. Using Olex2 (Dolomanov et al., 2009), the structure was solved with the ShelXS (Sheldrick, 2008) structure solution program, using the direct methods solution method. The model was refined with version of ShelXL (Sheldrick, 2008) using least squares minimisation.
A summary of data collection and structure refinement is reported in Table S5.† The crystal structures were deposited at CCDC with deposition number 1443037, 1443041 and 1443040 for SFX-MeOTAD, SFX-PTZ and SFX-TCz respectively.
4.7 Synthesis and characterization
Spiro[fluorene-9,9′-xanthene] (SFX).
A mixture of fluorenone (35.740 g, 198.324 mmol, 1 equiv.), phenol (186.643 g, 19.83 mol, 10 equiv.), and methane sulfonic acid (MeSO3H, d = 1.48 g mL−1, 51.48 mL, 79.33 mol, 4 equiv.) was heated at 150 °C under nitrogen for 24 h. The reaction mixture was then slowly added into water (1 L) and extracted with dichloromethane (750 mL). Organic layer was washed with 5% NaOH (300 mL) three times. Then it was filtered through silica plug (120 g) washed with DCM (100 mL). Concentrated on rotary evaporator to give beige solid which was suspended in EtOH (650 mL) and washed with EtOH (3 × 100 mL) and MeOH (100 mL) to afford colorless solid of SFX (50.753 g, 77%).
1H NMR (400 MHz, CDCl3, ppm): δ = 7.87 (d, J = 7.6 Hz, 2H), 7.45 (ddd, J = 7.6 Hz, J = 6.4 Hz, J = 2.2 Hz, 2H), 7.32–7.20 (m, 8H), 6.85 (ddd, J = 7.1 Hz, J = 2.3 Hz, 2H), 6.51 (m, 1H), 6.47 (d, J = 1.3 Hz, 1H).
2,2′,7,7′-Tetrabromo-spiro(fluorene-9,9′-xanthene) (SFX-TBr).
To a solution of spiro[fluorene-9,9′-xanthene] (40 g, 0.120 mol) in dichloromethane (400 mL) under nitrogen bromine (49.3 mL, 0.963 mol) was added. The resulting mixture was stirred at room temperature for 14 hours. After this time the 1H NMR spectroscopy confirmed forming expected product with no substrate or by-products. The solution was poured on 0.5 L of water, 500 mL of DCM was added and it was washed with saturated sodium thiosulfate (50 g). The organic layer was separated, dried over magnesium sulfate and concentrated on rotary evaporator to about 700 mL. Precipitated in MeOH (500 mL), filtrated and washed MeOH (100 mL) to give white powder of crude product 60.703 g (78%). It was purified by crystallization in 1.6 L of chloroform/ethanol mixture (1
:
1) to give 58.638 g (75%) of white crystalline solid.
Elemental analysis (%). Calculated for C25H12Br4O: C 46.34, H 1.87. Result: C 46.32, H 1.81.
1H NMR (500 MHz, CDCl3) δ 7.66 (s, 1H), 7.65 (s, 1H), 7.55 (dd, J = 8.2, 1.7 Hz, 2H), 7.34 (dd, J = 8.8, 2.4 Hz, 2H), 7.22 (d, J = 1.7 Hz, 2H), 7.13 (s, 1H), 7.11 (s, 1H), 6.43 (d, J = 2.3 Hz, 2H).
13C NMR (126 MHz, CDCl3) δ 155.54, 149.97, 137.59, 132.22, 130.40, 129.06, 124.81, 122.90, 121.97, 119.17, 116.21, 53.88.
MS (EI): m/z (%) = 643.8 [18], 645.8 [68], 647.8 [M+, 100], 649.8 [66], 650.8 [17].
2,2′,7,7′-Tetrakis(N,N-di(4-methoxyphenyl)amino)-spiro(fluorene-9,9′-xanthene) (SFX-MeOTAD).
Method A.
A mixture of 2,2′,7,7′-tetrabromo-spiro(fluorene-9,9′-xanthene) (0.200 g, 0.309 mmol), 4,4′-dimethoxydiphenylamine (0.425 g, 1.852 mmol), Pd2(dba)3 (0.057 g, 0.062 mmol), tri(o-tolyl)phosphine (0.113 g, 0.371 mmol), sodium tert-butoxide (0.178 g, 1.852 mmol) were added to a oven dried Schlenk tube and dried under vacuum for 2 hours. Dry toluene (4 mL) was added and the mixture was stirred under nitrogen at 110 °C for 20 hours. The solution was diluted with toluene filtered through Celite and extracted with water. The organic layer was dried over anhydrous sodium sulfate, filtered and the solvent was remove on rotary evaporator. The residue was purified by column chromatography (SiO2, eluent: acetone/hexane 1/8, 1/5, 1/4, v/v) and then precipitated from a solution of acetone into an excess of methanol. The precipitate was filtered off and washed with methanol to give 0.232 g (60%) as a yellowish solid.
Method B.
A mixture of 2,2′,7,7′-tetrabromo-spiro(fluorene-9,9′-xanthene) (7 g, 10.803 mmol), 4,4′-dimethoxydiphenylamine (12.384 g, 54.014 mmol), Pd(OAc)2 (0.048 g, 0.216 mmol), sodium tert-butoxide (6.227 g, 64.818 mmol) and tri-tert-butylphosphonium tetrafluoroborate (0.125 g, 0.432 mmol) were added to a oven dried Schlenk tube and dried under vacuum for 2 hours. Dry toluene (100 g mL) was added and the mixture was stirred under nitrogen at 110 °C for 20 hours. The solution was diluted with toluene (250 mL) filtered through silica plug (26 g) and washed with AcOEt (300 mL). It was extracted with water and organic phase was dried over anhydrous magnesium sulfate, filtrated and concentrated on rotary evaporator. The residue was purified by column chromatography (SiO2, 26 g, eluent: acetone/hexane 5.5 L/1.1 L, 4 L/1 L, 2.7 L/0.9 L) and then precipitated from a solution of acetone (750 mL) into methanol (1.5 L). Then concentrated on rotary evaporator to 0.5 L and the precipitate was filtered off and washed with methanol (300 mL) to give 9.540 g (71%) as a yellowish solid. 2.568 g of 4,4′-dimethoxydiphenylamine was recovered from the first fractions of column.
Elemental analysis (%). Calculated for C81H68N4O9: C 78.37, H 5.52, N 4.51. Result: C 78.40, H 5.43, N 4.46.
1H NMR (500 MHz, benzene-d6) δ 3.28 (s, 12H), 3.31 (s, 12H), 6.65–6.71 (m, 8H), 6.73–6.77 (m, 8H), 6.82 (dd, J = 8.8, 2.7 Hz, 2H), 6.86 (d, J = 2.6 Hz, 2H), 6.88–6.94 (m, 10H), 6.97 (d, J = 8.8 Hz, 2H), 7.00–7.05 (m, 8H), 7.09 (d, J = 8.3 Hz, 2H), 7.42 (d, J = 2.1 Hz, 2H).
13C NMR (126 MHz, benzene-d6) δ 55.0, 55.0, 114.9, 115.1, 118.0, 118.9, 120.4, 122.0, 122.1, 123.9, 125.3, 126.3, 127.2, 128.0, 128.2, 128.4, 133.4, 141.8, 142.1, 144.5, 147.8, 148.5, 155.2, 155.7, 156.2.
MS (ESI): m/z (%) = 1241.5 [(M + H)+, 100].
2,2′,7,7′-Tetrakis(N,N-diphenylamino)-spiro(fluorene-9,9′-xanthene) (SFX-TAD).
The same protocol as for SFX-MeOTAD (Method B).
Product was obtained as a colourless solid 0.388 g (56%).
Elemental analysis (%). Calculated for C73H52N4O: C 87.57, H 5.23, N 5.60. Result: C 87.53, H 5.18, N 5.68.
1H NMR (601 MHz, methylene chloride-d2) δ = 6.38 (d, J = 2.6, 2H), 6.84–7.01 (m, 32H), 7.14–7.21 (m, 16H), 7.39 (d, J = 8.2, 2H).
13C NMR (151 MHz, methylene chloride-d2) δ 54.0, 54.7, 118.3, 120.7, 121.3, 122.6, 123.2, 123.2, 124.4, 124.7, 125.5, 125.6, 126.9, 129.6, 129.7, 134.6, 143.1, 147.9, 148.0, 148.2, 148.3, 156.8.
MS (ESI): m/z (%) = 1000.41 [(M)+, 100].
2,2′,7,7′-Tetrakis(N-phenothiazylo)-spiro(fluorene-9,9′-xanthene) (SFX-TPTZ).
The same protocol as for SFX-MeOTAD (Method B) except product was purified by crystallization toluene/chlorobenzene mixture.
Product was obtained as a beige solid 0.300 g (35%).
Elemental analysis (%). Calculated for C73H44N4OS4: C 78.19, H 3.95, N 5.00. Result: C 78.08, H 4.00, N 5.13.
1H NMR (601 MHz, methylene chloride-d2) δ = 5.92 (dd, J = 8.3, 1.2, 4H), 6.02 (dd, J = 8.3, 1.2, 4H), 6.41 (ddd, J = 8.3, 7.3, 1.6, 4H), 6.55 (ddd, J = 8.2, 7.4, 1.6, 4H), 6.72 (m, 10H), 6.96 (ddd, J = 8.9, 7.6, 1.5, 8H), 7.26 (dd, J = 8.7, 2.5, 2H), 7.40–7.43 (m, 4H), 7.50 (d, J = 8.7, 2H), 8.03–8.05 (m, 2H).
13C NMR (151 MHz, methylene chloride-d2) δ 54.0, 55.1, 115.8, 116.3, 120.0, 120.3, 120.5, 123.0, 123.1, 123.6, 126.4, 127.1, 127.1, 127.8, 128.4, 130.6, 132.0, 132.5, 136.8, 139.2, 142.1, 144.6, 144.8, 151.2, 158.3, 206.9.
MS (ESI): m/z (%) = 1120.24 [(M)+, 30].
2,2′,7,7′-Tetrakis(N,N-carbazolo)-spiro(fluorene-9,9′-xanthene) (SFX-TCz).
The same protocol as for SFX-MeOTAD (Method B).
Product was obtained as a colourless solid 0.571 g (83%).
Elemental analysis (%). Calculated for C73H44N4O: C 88.28, H 4.47, N 5.64. Result: C 88.14, H 4.54, N 5.51.
1H NMR (500 MHz, methylene chloride-d2) δ = 6.95 (dd, J = 2.1, 0.8, 2H), 7.15 (dt, J = 8.1, 0.9, 4H), 7.19–7.40 (m, 20H), 7.45–7.51 (m, 4H), 7.63 (dd, J = 8.0, 1.9, 2H), 7.68 (m, J = 1.65, 2H), 7.99 (dd, J = 8.0, 0.6, 2H), 8.08 (ddd, J = 7.7, 1.4, 0.7, 4H), 8.15 (dt, J = 7.7, 1.0, 4H).
13C NMR (126 MHz, methylene chloride-d2) δ 54.0, 54.9, 109.8, 110.2, 119.4, 120.4, 120.7, 120.8, 120.9, 122.7, 123.6, 124.0, 124.7, 124.8, 126.6, 126.6, 127.7, 128.0, 128.5, 133.6, 138.5, 138.6, 141.3, 141.4, 150.4, 158.0.
MS (ESI): m/z (%) = 992.35 [(M)+, 30].
4.8 Perovskite solar cell fabrication and characterisation
The mesoporous Al2O3 based perovskite (CH3NH3PbI3−xClx) solar cells have been fabricated as reported in literature32,33 with slight modification.30 Briefly, fluorine-doped tin oxide coated glass substrates (FTO, 7 Ω sq−1, Solaronix) were etched with zinc powder and HCl (4 M aqueous solution) to give the desired electrode patterning. The patterned substrates were cleaned with Hellmanex (2% by volume in de-ionized water) and then sequentially ultrasonicated in de-ionized water, acetone, and methanol. Oxygen plasma cleaning was then used to remove the last traces of organics. A 50 nm blocking layer of TiO2 was then deposited by spin coating (speed 2000 rpm, 2000 rpm s−1 ramp) a weakly acidic solution of titanium isopropoxide in ethanol (350 μL in 5 mL ethanol with 0.013 M HCl), and annealed at 500 °C for 30 min. A 400 nm of mesoporous Al2O3 layer was deposited by spin-coating (speed 2500 rpm, ramp 2500 rpm s−1, time 60 s) a colloidal dispersion of <50 nm Al2O3 nanoparticles (Sigma-Aldrich, CAS number 702129) in isopropanol (1
:
2 by volume), followed by drying at 150 °C for 10 min to remove any residual isopropanol. The perovskite was deposited in nitrogen filled glovebox (with <30% relative humidity) by spin coating (speed 2000 rpm, ramp 2000 rpm s−1) a 40 wt% DMF solution of methylammonium iodide (synthesized as reported in literature) and PbCl2 (3
:
1 molar ratio). The substrate is then heated to 100 °C for 2 hours on a hotplate in nitrogen filled glovebox after DMF solvent annealing. The hole-transport layer was deposited by spin coating an 8.5 vol% spiro-MeOTAD (or SFX-MeOTAD) in chlorobenzene solution with the standard additives 0.8 mM tert-butylpyridine and 20 mM lithium bis(trifluoromethanesulfonyl)imide (from 0.61 M acetonitrile solution). Gold electrodes (∼50 nm) were then deposited by evaporation under high vacuum through a shadow mask.
The current–voltage (I–V) curves were measured (Potentiostat/Galvanostat, Metrohm Autolab B.V.) under simulated AM 1.5 sunlight at 100 mW cm−2 irradiance generated by a Class AAA small collimated Beam Solar simulator (SF300A, Sciencetech Inc.), with the intensity calibrated by Si reference cell. The mismatch factor between the simulated sunlight and the actual solar spectrum is not corrected. The solar cells were masked with a metal aperture to define the active area, typically 0.06–0.09 cm−2. Hitachi 470011 cold field-emission scanning electron microscope has been utilised to do the cross-section SEM of the PSCs.
The hole conductivity measurements have been carried out using two probe method for the spiro-MeOTAD and SFX-MeOTAD films [with standard additives of 0.8 mM tert-butylpyridine and 20 mM lithium bis(trifluoromethanesulfonyl)imide (from 0.61 M acetonitrile solution)] deposited on p = doped Si wafer with SiO2 (100 nm) coating and electrode consisting of Cr (5 nm) and Au (50 nm) 1 mm in width and separated by 0.03 mm.
Acknowledgements
We thank the EPSRC Supersolar Hub, and the EPSRC Apex project (EP/M023532/1) for financial support (Open Data: http://dx.doi.org/10.7488/ds/1353). We thank the University of Edinburgh for funding the diffractometer purchase.
Notes and references
- A. Kojima, K. Teshima, Y. Shirai and T. Miyasaka, J. Am. Chem. Soc., 2009, 131, 6050–6051 CrossRef CAS PubMed.
-
M. Grätzel and A. Hagfeldt, http://www.dyesol.com/media/wysiwyg/Documents/2015-asx-announcements/2015-12-08-DYE0397_-_EPFL_achieves_21_efficiency.pdf.
- P. Gratia, A. Magomedov, T. Malinauskas, M. Daskeviciene, A. Abate, S. Ahmad, M. Grätzel, V. Getautis and M. K. Nazeeruddin, Angew. Chem., Int. Ed., 2015, 54, 11409–11413 CrossRef CAS PubMed.
- F. Zhang, X. Yang, M. Cheng, J. Li, W. Wang, H. Wang and L. Sun, J. Mater. Chem. A, 2015, 3, 24272–24280 CAS.
- K. Rakstys, A. Abate, M. I. Dar, P. Gao, V. Jankauskas, G. Jacopin, E. Kamarauskas, S. Kazim, S. Ahmad, M. Grätzel and M. K. Nazeeruddin, J. Am. Chem. Soc., 2015, 137, 16172–16178 CrossRef CAS PubMed.
- S. Park, J. H. Heo, C. H. Cheon, H. Kim, S. H. Im and H. J. Son, J. Mater. Chem. A, 2015, 3, 24215–24220 CAS.
- M. L. Petrus, T. Bein, T. J. Dingemans and P. Docampo, J. Mater. Chem. A, 2015, 3, 12159–12162 CAS.
- A. Abate, M. Planells, D. J. Hollman, V. Barthi, S. Chand, H. J. Snaith and N. Robertson, Phys. Chem. Chem. Phys., 2015, 17, 2335–2338 RSC.
- Y. Song, S. Lv, X. Liu, X. Li, S. Wang, H. Wei, D. Li, Y. Xiao and Q. Meng, Chem. Commun., 2014, 50, 15239–15242 RSC.
- L.-H. Xie, F. Liu, C. Tang, X.-Y. Hou, Y.-R. Hua, Q.-L. Fan and W. Huang, Org. Lett., 2006, 8, 2787–2790 CrossRef CAS PubMed.
- M. Sun, R. Xu, L. Xie, Y. Wei and W. Huang, Chin. J. Chem., 2015, 33, 815–827 CrossRef CAS.
- M. Pietraszkiewicz, M. Maciejczyk, I. D. W. Samuel and S. Zhang, J. Mater. Chem. C, 2013, 1, 8028–8032 RSC.
- X. Zhao, Y. Wu, N. Shi, X. Li, Y. Zhao, M. Sun, D. Ding, H. Xu and L. Xie, Chin. J. Chem., 2015, 33, 955–960 CrossRef CAS.
- N. Cocherel, C. Poriel, L. Vignau, J.-F. Bergamini and J. Rault-Berthelot, Org. Lett., 2010, 12, 452–455 CrossRef CAS PubMed.
- J.-F. Gu, G.-H. Xie, L. Zhang, S.-F. Chen, Z.-Q. Lin, Z.-S. Zhang, J.-F. Zhao, L.-H. Xie, C. Tang, Y. Zhao, S.-Y. Liu and W. Huang, J. Phys. Chem. Lett., 2010, 1, 2849–2853 CrossRef CAS.
- Z. Chu, D. Wang, C. Zhang, F. Wang, H. Wu, Z. Lv, S. Hou, X. Fan and D. Zou, Synth. Met., 2012, 162, 614–620 CrossRef CAS.
- B. E. Koene, D. E. Loy and M. E. Thompson, Chem. Mater., 1998, 10, 2235–2250 CrossRef CAS.
- B. A. Kamino, B. Mills, C. Reali, M. J. Gretton, M. A. Brook and T. P. Bender, J. Org. Chem., 2012, 77, 1663–1674 CrossRef CAS PubMed.
- W.-F. Jiang, H.-L. Wang, A.-G. Wang and Z.-Q. Li, Synth. Commun., 2008, 38, 1888–1895 CrossRef CAS.
- J. Pei, J. Ni, X.-H. Zhou, X.-Y. Cao and Y.-H. Lai, J. Org. Chem., 2002, 67, 4924–4936 CrossRef CAS PubMed.
- K. Zhang, L. Wang, Y. Liang, S. Yang, J. Liang, F. Cheng and J. Chen, Synth. Met., 2012, 162, 490–496 CrossRef CAS.
- I. K. Ding, J. Melas-Kyriazi, N. Le Cevey-Ha, K. G. Chittibabu, S. M. Zakeeruddin, M. Grätzel and M. D. McGehee, Org. Electron., 2010, 11, 1217–1222 CrossRef CAS.
- A. Murray, J. M. Frost, C. Hendon, C. D. Molloy, D. Carbery and A. Walsh, Chem. Commun., 2015, 51, 8935–8938 RSC.
- N. Joong Jeon, H. Geun Lee, Y. Chan Kim, J. Seo, J. Hing Noh, J. Lee and S. Il Seok, J. Am. Chem. Soc., 2014, 7837 CrossRef PubMed.
- W. Rettig, Appl. Phys. B: Photophys. Laser Chem., 1988, 45, 145–149 CrossRef.
- J. Catalán, C. Díaz, V. López, P. Pérez and R. M. Claramunt, J. Phys. Chem., 1996, 100, 18392–18398 CrossRef.
- P. Ganesan, K. Fu, P. Gao, I. Raabe, K. Schenk, R. Scopelliti, J. Luo, L. H. Wong, M. Grätzel and M. K. Nazeeruddin, Energy Environ. Sci., 2015, 8, 1986–1991 CAS.
- H. J. Snaith and M. Grätzel, Appl. Phys. Lett., 2006, 89, 262114 CrossRef.
- A. Abate, D. R. Staff, D. J. Hollman, H. J. Snaith and A. B. Walker, Phys. Chem. Chem. Phys., 2014, 16, 1132–1138 RSC.
- Z. Xiao, Q. Dong, C. Bi, Y. Shao, Y. Yuan and J. Huang, Adv. Mater., 2014, 26, 6503–6509 CrossRef CAS PubMed.
- N. Ahn, D.-Y. Son, I.-H. Jang, S. M. Kang, M. Choi and N.-G. Park, J. Am. Chem. Soc., 2015, 137, 8696–8699 CrossRef CAS PubMed.
- J. M. Ball, M. M. Lee, A. Hey and H. J. Snaith, Energy Environ. Sci., 2013, 6, 1739 CAS.
- M. M. Lee, J. Teuscher, T. Miyasaka, T. N. Murakami and H. J. Snaith, Science, 2012, 338, 643–647 CrossRef CAS PubMed.
- A. Krishna, D. Sabba, H. Li, J. Yin, P. P. Boix, C. Soci, S. G. Mhaisalkar and A. C. Grimsdale, Chem. Sci., 2014, 5, 2702 RSC.
- Y. Song, S. Lv, X. Liu, X. Li, S. Wang, H. Wei, D. Li, Y. Xiao and Q. Meng, Chem. Commun., 2014, 50, 15239–15242 RSC.
- T. Leijtens, I.-K. Ding, T. Giovenzana, J. T. Bloking, M. D. McGehee and A. Sellinger, ACS Nano, 2012, 6, 1455–1462 CrossRef CAS PubMed.
- C. M. Cardona, W. Li, A. E. Kaifer, D. Stockdale and G. C. Bazan, Adv. Mater., 2011, 23, 2367–2371 CrossRef CAS PubMed.
Footnote |
† Electronic supplementary information (ESI) available: Additional electrochemical measurements of SFX derivatives, DSC curves, cost estimation, extended crystallographic table and X-ray structures, 1H and 13C NMR, COSY, NOESY and HSQC spectra for all synthesized compounds. CCDC 1443037, 1443041 and 1443040. For ESI and crystallographic data in CIF or other electronic format see DOI: 10.1039/c6ta00110f |
|
This journal is © The Royal Society of Chemistry 2016 |
Click here to see how this site uses Cookies. View our privacy policy here.