DOI:
10.1039/C6SC00011H
(Edge Article)
Chem. Sci., 2016,
7, 3154-3161
High yielding synthesis of 2,2′-bipyridine macrocycles, versatile intermediates in the synthesis of rotaxanes†
Received
2nd January 2016
, Accepted 26th January 2016
First published on 27th January 2016
Abstract
We present an operationally simple approach to 2,2′-bipyridine macrocycles. Our method uses simple starting materials to produce these previously hard to access rotaxane precursors in remarkable yields (typically >65%) across a range of scales (0.1–5 mmol). All of the macrocycles reported are efficiently converted (>90%) to rotaxanes under AT-CuAAC conditions. With the requisite macrocycles finally available in sufficient quantities, we further demonstrate their long term utility through the first gram-scale synthesis of an AT-CuAAC [2]rotaxane and extend this powerful methodology to produce novel Sauvage-type molecular shuttles.
Introduction
The synthesis of mechanically interlocked molecules has progressed significantly since early reports.1,2 This was largely made possible by the development of passive template methodologies, inspired by Sauvage and co-workers' seminal work,3 in which non-covalent interactions direct mechanical bond formation.4 Thus, there exists a range of methodologies for the synthesis of complex interlocked structures in excellent yield in the mechanical bond-forming step.
Despite innovations in the preparation of interlocked molecules,5 the synthesis of the requisite functionalised macrocyclic components is often a challenge that can in turn limit the scalability of their synthesis, hindering their investigation for applications such as molecular machines,6 materials,7 drug delivery agents,8 and catalysts.9 Indeed the synthesis of macrocyclic molecules more generally remains an important area of investigation due to their unusual properties10 combined with the inherent problem of selecting the desired ring closure event over oligomerisation.11 A number of strategies have been developed to overcome this including the use of templating interactions to pre-organise the acyclic precursor for ring closure, the preparation of rigid acyclic precursors with restricted rotational degrees of freedom, and expansion of smaller rings.12 Where this is not possible, the most general approach is to carry out the ring-closing step under high dilution, conditions with significant consequences for the scalability of the process.13
The contrast in efficiency between the synthesis of the macrocycle and the formation of the mechanical bond is perhaps nowhere more acute than in our small bipyridine macrocycle modification14 of Leigh's active template Cu-mediated alkyne-azide cycloaddition15 (AT-CuAAC)16 reaction.17,18 Although AT-CuAAC reactions with small bipyridine macrocycles have been described as “amongst the highest yielding and readily accessible routes to stable interlocked structures”,4c the synthesis of the macrocyclic bipyridine precursor is extremely inefficient, proceeding in just 4–11% yield. Given the proven utility of the AT-CuAAC reaction for the synthesis of functionalised rotaxanes,14a,19 including examples with multiple mechanical bonds,14d,20 stabilised organometallic species,14b stereochemically complex interlocked molecules,14c interlocked catalysts,9g and molecular machines,6a the limitations placed on the application of this powerful methodology by the poor availability of the most efficacious macrocyclic precursors19 is a significant barrier to further developments.
Here we report an operationally simple method for the scalable synthesis of bipyridine macrocycles from readily available substrates that effectively removes this limitation. All of the bipyridine macrocycles reported are efficiently converted to [2]rotaxanes under standard conditions. We demonstrate the long term potential of the AT-CuAAC approach with these now readily available substrates through the gram-scale synthesis of a [2]rotaxane catalyst precursor, and a novel synthesis of Sauvage-type molecular shuttles.
Results and discussion
A new Ni-mediated macrocyclisation
Bipyridine macrocycles suitable for AT-CuAAC reactions such as 2a are typically synthesised via a double Williamson cyclisation of a pre-formed bipyridine precursor.14,16b,21 However, the preferred trans-rotamer of the bipyridine unit22 disfavours the desired cyclisation, leading to low yields, particularly in the case of smaller macrocycles.23 To overcome this, we resolved to form the ligand during the macrocyclisation reaction.
Previous routes to bipyridine macrocycles have made use of the Ni-mediated reductive coupling24 of 2-halo pyridines25 to produce the bipyridine moiety prior to the macrocyclisation step.14,16b Although Ni-mediated couplings have not been employed as a macrocyclisation reaction for the preparation of bipyridine macrocycles for incorporation into interlocked architectures,26 similar reactions have been successful in the key cyclisation step towards cyclic and bicyclic oligoparaphenylenes,27,28 as well as limited examples of bi-phenyl and bi-benzyl natural products.29 Accordingly, dibromo precursor 1a was synthesised from known intermediates in a succinct manner (see ESI†) and, under high dilution, subjected to conditions based on those developed by the groups of Tiecco and Iyoda for the Ni-mediated intermolecular homo-coupling of 2-halo-pyridines.25a,c No reaction was observed (Table 1, entry 1).
Table 1 Optimisation of the Ni-mediated synthesis of macrocycle 2aa
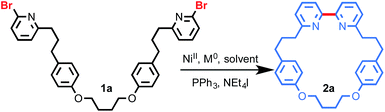
|
Entry |
NiII source |
M0 (equiv.) |
Yieldb |
Reagents and conditions: 0.1 mmol each 1a, NiII, NEt4I, 0.4 mmol PPh3 in total, DMF, 50 °C, 4 h.
Determined by 1H NMR analysis of the crude product.
5 mM conc. of 1a.
0.05 M conc. of 1a.
Pseudo-high dilution (4 h addition, 0.05 M final conc. of 1a).
THF as solvent. n.r. = no reaction.
|
1c |
NiCl2·6H2O |
Zn (2) |
n.r. |
2d |
NiCl2·6H2O |
Zn (2) |
7% |
3e |
NiCl2·6H2O |
Zn (2) |
37% |
4e |
[Ni(PPh3)2Br2] |
Zn (2) |
60% |
5e,f |
[Ni(PPh3)2Br2] |
Zn (2) |
47% |
6e |
[Ni(PPh3)2Br2] |
Zn (4) |
50% |
7e |
[Ni(PPh3)2Br2] |
Mn (2) |
63% |
8e |
[Ni(PPh3)2Br2] |
Mn (10) |
70% |
Repeating the same reaction at higher concentration (entry 2) led to consumption of 1a to give 2a, albeit in low yield. Combining these observations, slow addition of 1a to the preformed Ni0 species under otherwise identical conditions gave macrocycle 2a in 37% yield (entry 3). A brief screen of reaction conditions revealed that replacing NiCl2·6H2O with NiBr2(PPh3)2 resulted in an increased yield of 2a (entry 4), whereas conducting the reaction in THF, commonly employed in such couplings,25a led to a less efficient reaction (entry 5). Increasing the equivalents of Zn reduced the yield of product, with significant proto-dehalogenation observed in the crude mixture (entry 6). On the hypothesis that this was due to halogen–zinc exchange competing with the desired Ni-mediated process, Mn was substituted for Zn leading to an improved yield (entry 7).30 Finally, increasing the equivalents of Mn (entry 8) to improve the efficiency of reductive steps during the catalytic cycle,24 gave 2a in 70% yield.
Preparative scale synthesis of bipyridine macrocycles
Under our optimised conditions, the preparative scale (2 mmol) reaction of 1a provided macrocycle 2a in an excellent 71% isolated yield (Fig. 1) and so we extended our investigation to a selection of previously reported and novel macrocycles. An ether link proximal to the pyridine unit did not affect the outcome; previously disclosed macrocycles 2b and 2c were produced in excellent yields, demonstrating that, unlike Williamson ether methods, the Ni-mediated cyclisation is relatively insensitive to ring size. Furthermore, when the dichloro analogue of 1b was employed, macrocycle 2b was produced in 56% yield, indicating that 2-chloro pyridines are also viable substrates. The reaction also tolerates heteroatom substitution on the pyridine ring (2d) although in this case an elevated temperature was required due to the lower reactivity of the more electron-rich substrate.31 Similarly, the reaction tolerates 6-aryl-substitution; sterically hindered macrocycle 2e, a smaller analogue of Sauvage's iconic phenanthroline macrocycle,3 was produced in excellent 73% yield.
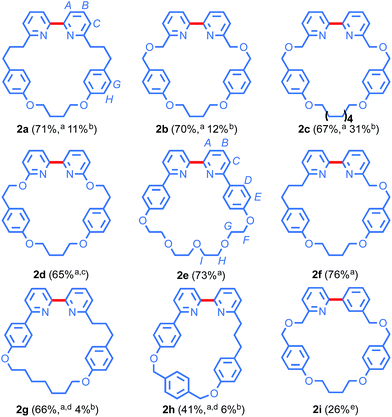 |
| Fig. 1 Synthesis of macrocycles 2 at 2 mmol scale. aReaction conditions as in Table 1, entry 8. bMacrocyclisation yield of the best previous synthesis. cT = 70 °C. dSolvent = DMF–THF (3 : 1). eYield from Br/I precursor. | |
We then extended the Ni-mediated coupling to the synthesis of unsymmetrical bipyridine macrocycles, important intermediates in the synthesis of mechanically chiral molecules.14c Macrocycle 2f, a hybrid of macrocycles 2a and 2b in which the two pyridine moieties are differentiated by a proximal ether unit, was produced in excellent 76% yield. Initial attempts to extend our approach to previously disclosed macrocycle 2g were hampered by the poor solubility of 1g in DMF. This was readily overcome by employing a DMF–THF solvent mixture, leading to 2g in 66% yield, an order of magnitude improvement over the previous synthesis (4%). When the flexible alkyl chain of 2g was replaced by a p-xylyl moiety to give 2h, the yield of the macrocyclisation reaction fell to 41%, presumably due to the increased strain of the smaller, more rigid macrocycle. However, the yield of 2h remains significantly higher than previous syntheses (6%; see ESI†) and demonstrates that the Ni-coupling can be used to generate more challenging structures.
Finally, we extended our approach to an aryl-pyridine cross-coupling. Initial attempts with the corresponding dibromo precursor delivered 2i in a low 16% yield. However, replacing the bromo-benzene moiety with an iodo-benzene starting material raised the yield to 24%, suggesting that there is potential to extend our efficient Ni-mediated ring closure to other biaryl macrocyclic motifs in future.
The Ni-mediated synthesis of bipyridine macrocycles is obviously a dramatic improvement over established procedures in terms of simple reaction yield, by as much as an order of magnitude in some cases. Other key benefits of this methodology include: (i) the Ni-mediated coupling appears relatively insensitive to substrate structure (ring size, pyridine substituents) and tolerates simple modifications (temperature, solvent) where required; (ii) the reaction is capable of producing unsymmetrical bipyridine macrocycles such as 2f–i through a formal intramolecular cross-coupling reaction; (iii) bis-halopyridine precursors 1 are readily available on scale using simple chemistry and commercially available precursors; (iv) isolation of the product is simplified both by the improved impurity profile of the crude product and by the use of pseudo-high dilution conditions.
This latter point renders the Ni-mediated macrocyclisation highly scalable; whereas previous syntheses of small bipyridine macrocycles 2a, 2b, 2g and 2h required between 3 and 9 litres of DMF per mmol of product, only ∼30 ml of DMF is required to produce each mmol of macrocycle using the method presented here. Using James' EMAC measure of reaction efficiency,13 a logarithmic scale taking into account the yield and reaction concentration, this corresponds to an increase in reaction efficiency from EMAC = 3 to 7. Furthermore, the excellent reaction yield is maintained across a range of scales; repeating the synthesis of 2a (Scheme 1) with 5 mmol of 1a delivered 1.6 g of macrocycle 2a (69% isolated yield).
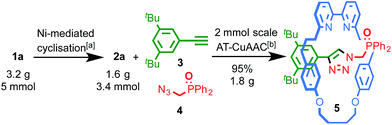 |
| Scheme 1 Large scale macrocyclisation and AT-CuAAC reactions. aConditions as Table 1, entry 8. b[Cu(MeCN)4](PF6), NiPr2Et, CH2Cl2–EtOH (1 : 1), 80 °C, 18 h. | |
Bipyridine macrocycles for the scalable synthesis of [2]rotaxanes in excellent yield
Macrocycles 2a–c and 2g are well precedented in the high yielding AT-CuAAC synthesis of interlocked molecules.9g,14,16b Pleasingly, preliminary investigations confirmed that macrocycles 2d–f and 2h are also excellent rotaxane precursors; under AT-CuAAC conditions with simple alkyne and azide coupling partners, 2d–f and 2h are effectively converted (>90% in all cases) to interlocked products (see ESI†).32
Furthermore, with significant quantities of macrocycles 2 in hand we were able for the first time to demonstrate the scalability of the AT-CuAAC reaction. Previous reports have been limited to small scales (typically 0.025 mmol) by the availability of the macrocycle component. Pleasingly, when the synthesis of interlocked phosphine oxide 5, an advanced precursor of a stimuli-responsive interlocked Au catalyst,9g was carried out at an 80-fold larger scale using 0.96 g of macrocycle 2a (2 mmol) the isolated yield of the interlocked product was increased relative to our previous report, delivering 1.8 g (95%) of 5 in a single synthetic operation.
An efficient AT-CuAAC approach to Sauvage-type molecular shuttles
Now that bipyridine macrocycles are far more readily available their application in more complex mechanically interlocked molecules and molecular machines is a far more attractive proposition. To capitalise on this, we developed a streamlined synthesis of two Sauvage-type molecular shuttles using macrocycles 2a and 2e and compared their behaviour. Such shuttles are usually based on phenanthroline macrocycles and a thread containing a terpyridine and a bipyridine station, assembled using a passive template threading approach.3c Replacing the phenanthroline macrocycle with its bipyridine analogue and the terpyridine and bipyridine stations with bistriazolylpyridine (btp) and monotriazolylpyridine (mtp) units respectively,33,34 greatly facilitates shuttle synthesis using an AT-CuAAC approach. Thus, shuttles 9a and 9e were synthesised in a concise manner from readily available ethynyl pyridine derivatives 6 and 8 through sequential CuAAC couplings of 1,6 bis-azido hexane, delivering 9a and 9e in 73% and 52% isolated yields respectively in the mechanical bond-forming step (Scheme 2).35 The corresponding non-interlocked thread was also synthesised for comparison. With shuttles 9 in hand, we examined their co-conformational behaviour by 1H and ROESY NMR (Fig. 2 and ESI respectively†).
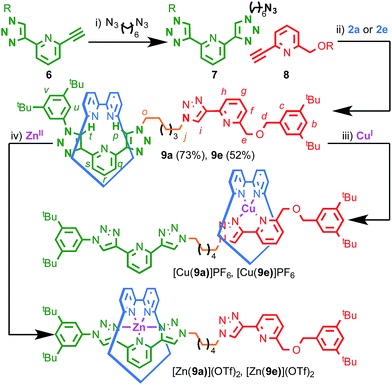 |
| Scheme 2 The AT-CuAAC synthesis and operation of bi-stable molecular shuttles 9. Reagents and conditions: (i) [Cu(MeCN)4](PF6), CH2Cl2, rt; (ii) 2a or 2e [Cu(MeCN)4](PF6), NiPr2Et, CH2Cl2, 80 °C; (iii) [Cu(MeCN)4](PF6), CDCl3; (iv) Zn(OTf)2, CDCl3. R = 3,5-di-tBu-C6H3. | |
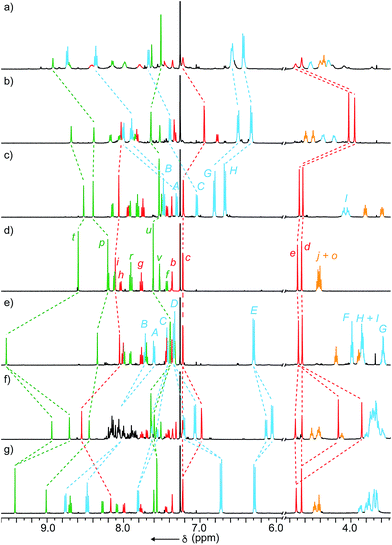 |
| Fig. 2 Partial 1H NMR (500 MHz, CDCl3) of (a) 9a + Zn(OTf)2; (b) 9a + [Cu(MeCN)4](PF6); (c) 9a; (d) non-interlocked thread; (e) 9e; (f) 9e + [Cu(MeCN)4](PF6); (g) 9e + Zn(OTf)2. Selected signals assigned with labels as in Fig. 1 (macrocycle) and Scheme 2 (thread). | |
Analysis of shuttles 9a and 9e by 1H NMR (Fig. 2c and e respectively) suggests that in both cases the macrocycle predominantly occupies the tridentate btp station; significant shifts of thread triazole protons Hp and Ht were observed relative to the non-interlocked thread (Fig. 2d), whereas protons associate with the bidentate mtp unit remain largely unaffected by the formation of the mechanical bond. ROESY NMR analysis supports this assignment with cross-peaks observed between macrocycle protons and Hq, Hr and Hs of the thread. Given the significant shifts of protons Hp and Ht and our previous observation of C–H⋯N hydrogen bonding in AT-CuAAC derived rotaxanes,14a the localisation of the macrocycle over the btp station in rotaxanes 9 is tentatively attributed to the presence of two C–H⋯N hydrogen-bonding interactions compared with only one in the case of the mtp station.
Next we investigated shuttles 9 in the presence of diamagnetic metal ions CuI and ZnII in order to monitor their co-conformational behaviour by NMR, as previously reported by Sauvage in the case of phenanthroline derived shuttles.3c,36 Addition of [Cu(MeCN)4](PF6) to 9a led to large changes in the 1H NMR (Fig. 2b) consistent with the expected shuttling of the macrocycle to the bidentate mtp station to form the preferred tetradentate coordination site for CuI; protons Hc, Hd and He are shielded significantly and ROESY cross-peaks are observed between mtp triazole proton Hi of the thread and alkyl proton HI of the macrocycle. Replacing CuI with ZnII resulted in a new species consistent with the expected complex in which the macrocycle occupies the btp station, providing a pentadentate binding site for Zn; signals associated with the mtp station return to values similar to that of the thread and cross-peaks are observed between the triazole protons Hp and Ht of the btp station and HI of the macrocycle. Thus it appears that shuttle 9a behaves as a simple bipyridine–mtp/btp analogue of Sauvage's phenanthroline–bipyridine/terpyridine shuttle.3c
The behaviour of shuttle 9e proved to be more complicated. Surprisingly, addition of CuI led to the formation of two new species in an approximate 2
:
1 ratio (Fig. 2f). Two-dimensional exchange spectroscopy (2D-EXSY)37 confirmed that these co-conformational isomers are in slow exchange on the NMR timescale with a unimolecular rate constant at room temperature of the order of 10−3 s−1 corresponding to an activation barrier of ∼21 kcal mol−1. The major co-conformation was assigned as that in which the macrocycle, as initially expected, is localised over the bidentate mtp station, based on the shielding of signals corresponding to He, Hd and Hc, and ROESY cross-peaks between HD and HE of the macrocycle and He and Hd of the thread.
Similar analysis confirmed that the minor co-conformation is that in which the macrocycle unexpectedly coordinates the CuI ion at the nominally tridentate btp station, initially suggestive of a five-coordinate Cu centre. However, Schmittel and co-workers have previously reported the formation of a heteroleptic CuI complex derived from one tridentate and one bidentate ligand in which the metal ion adopts the expected four-coordinate geometry, with the fifth donor not involved in binding to the metal ion.38 Although this phenomenon requires further investigation, we tentatively suggest that similar behaviour may account for the minor co-conformation of [Cu(9a)](PF6), with subtle differences in the secondary interactions (C–H⋯π, π⋯π) between macrocycles 2a or 2e and the thread accounting for the differences observed.
Finally, replacing CuI with ZnII led to a much simpler outcome; a single new species was observed with 1H and ROESY NMR confirming that, in the case of the ZnII complex [Zn(9e)](OTf)2, the macrocycle was located predominantly on the tridentate btp station, as expected (Fig. 2g).
Conclusions
In conclusion we have presented an extremely efficient, scalable and general Ni-mediated method for the synthesis of small bipyridine macrocycles for the AT-CuAAC reaction in high yield from readily available precursors under pseudo high dilution conditions. Although this preliminary study has focussed on macrocycles similar to those used previously in the AT-CuAAC reaction, Ni-mediated couplings are typically tolerant to a wide range of functional groups24b and investigations are currently underway to determine the wider substrate scope. These now readily available macrocycles are proven versatile intermediates for the synthesis of interlocked molecules in excellent yield using both active14,16b,18a–e and passive39 template methods. Given the scalability of both the macrocycle synthesis and the mechanical bond forming step demonstrated here, and the clear potential to extend the approach to more complex molecules, the AT-CuAAC reaction mediated by bipyridine macrocycles clearly has a bright future in the synthesis of interlocked architectures for a variety of applications.
Acknowledgements
We thank Fluorochem for the gift of reagents, the EPSRC National Mass Spectrometry Service for HRMS analysis and the EPSRC (EP/L016621/1), Queen Mary, University of London and the University of Southampton for funding. JEML is a European Commission Marie Skłodowska-Curie Fellow. SMG is a Royal Society Research Fellow. This project has received funding from the European Union’s Horizon 2020 research and innovation programme under the Marie Sklodowska-Curie grant agreement No 660731.
Notes and references
-
(a) E. Wasserman, J. Am. Chem. Soc., 1960, 82, 4433–4434 CrossRef CAS;
(b) I. T. Harrison and S. Harrison, J. Am. Chem. Soc., 1967, 89, 5723–5724 CrossRef CAS.
-
(a) G. Schill and A. Lüttringhaus, Angew. Chem., Int. Ed. Engl., 1964, 3, 546–547 CrossRef;
(b) G. Schill, W. Beckmann and W. Vetter, Angew. Chem., Int. Ed. Engl., 1973, 12, 665–666 CrossRef.
-
(a) C. O. Dietrich-Buchecker, J.-P. Sauvage and J.-P. Kintzinger, Tetrahedron Lett., 1983, 24, 5095–5098 CrossRef CAS;
(b) C. O. Dietrich-Buchecker, J.-P. Sauvage and J. Kern, J. Am. Chem. Soc., 1984, 106, 3043–3045 CrossRef CAS;
(c) R. Dimer, C. Dietrich-buchecker, M. C. Jime and J.-P. Sauvage, Angew. Chem., Int. Ed. Engl., 2000, 1, 3284–3287 Search PubMed;
(d) J.-P. Collin, C. Dietrich-Buchecker, P. Gaviña, M. C. Jimenez-Molero and J.-P. Sauvage, Acc. Chem. Res., 2001, 34, 477–487 CrossRef CAS PubMed.
- For reviews on the synthesis of interlocked molecules see:
(a) M. S. Vickers and P. D. Beer, Chem. Soc. Rev., 2007, 36, 211–225 RSC;
(b) J. F. Stoddart, Chem. Soc. Rev., 2009, 38, 1802–1820 RSC;
(c) J. E. Beves, B. A. Blight, C. J. Campbell, D. A. Leigh and R. T. McBurney, Angew. Chem., Int. Ed., 2011, 50, 9260–9327 CrossRef CAS PubMed;
(d) N. H. Evans and P. D. Beer, Chem. Soc. Rev., 2014, 43, 4658–4683 RSC.
- For reviews on the applications of mechanically interlocked molecules see:
(a) K. Ito, Curr. Opin. Solid State Mater. Sci., 2010, 14, 28–34 CrossRef CAS;
(b) X. Ma and H. Tian, Chem. Soc. Rev., 2010, 39, 70–80 RSC;
(c) R. S. Forgan, J.-P. Sauvage and J. F. Stoddart, Chem. Rev., 2011, 111, 5434–5464 CrossRef CAS PubMed;
(d) V. N. Vukotic and S. J. Loeb, Chem. Soc. Rev., 2012, 41, 5896–5906 RSC;
(e) E. A. Neal and S. M. Goldup, Chem. Commun., 2014, 50, 5128–5142 RSC;
(f) S. F. M. van Dongen, S. Cantekin, J. A. A. W. Elemans, A. E. Rowan and R. J. M. Nolte, Chem. Soc. Rev., 2014, 43, 99–122 RSC;
(g) S. Erbas-Cakmak, D. A. Leigh, C. T. McTernan and A. L. Nussbaumer, Chem. Rev., 2015, 115, 10081–10206 CrossRef CAS PubMed;
(h) M. Xue, Y. Yang, X. Chi, X. Yan and F. Huang, Chem. Rev., 2015, 115, 7398–7501 CrossRef CAS PubMed.
- Selected recent examples of interlocked molecular machines:
(a) B. Lewandowski, G. De Bo, J. W. Ward, M. Papmeyer, S. Kuschel, M. J. Aldegunde, P. M. E. Gramlich, D. Heckmann, S. M. Goldup, D. M. D'Souza, A. E. Fernandes and D. A. Leigh, Science, 2013, 339, 189–193 CrossRef CAS PubMed;
(b) S. F. M. van Dongen, J. Clerx, K. Nørgaard, T. G. Bloemberg, J. J. L. M. Cornelissen, M. A. Trakselis, S. W. Nelson, S. J. Benkovic, A. E. Rowan and R. J. M. Nolte, Nat. Chem., 2013, 5, 945–951 CrossRef CAS PubMed;
(c) G. Ragazzon, M. Baroncini, S. Silvi, M. Venturi and A. Credi, Nat. Nanotechnol., 2014, 10, 70–75 CrossRef PubMed;
(d) C. Cheng, P. R. McGonigal, S. T. Schneebeli, H. Li, N. A. Vermeulen, C. Ke and J. F. Stoddart, Nat. Nanotechnol., 2015, 10, 547–553 CrossRef CAS PubMed.
- Selected reviews and recent examples of interlocked materials:
(a) T. Takata, Polym. J., 2006, 38, 1–20 CrossRef CAS;
(b) M. J. Frampton and H. L. Anderson, Angew. Chem., Int. Ed., 2007, 46, 1028–1064 CrossRef CAS PubMed;
(c) A. Harada, Y. Takashima and H. Yamaguchi, Chem. Soc. Rev., 2009, 38, 875–882 RSC;
(d) F. L. Thorp-Greenwood, A. N. Kulak and M. J. Hardie, Nat. Chem., 2015, 7, 526–531 CrossRef CAS PubMed;
(e) M. Franz, J. A. Januszewski, D. Wendinger, C. Neiss, L. D. Movsisyan, F. Hampel, H. L. Anderson, A. Görling and R. R. Tykwinski, Angew. Chem., Int. Ed., 2015, 54, 6645–6649 CrossRef CAS PubMed.
- Selected examples of interlocked drug delivery systems:
(a) A. Fernandes, A. Viterisi, F. Coutrot, S. Potok, D. A. Leigh, V. Aucagne and S. Papot, Angew. Chem., 2009, 121, 6565–6569 CrossRef;
(b) M. W. Ambrogio, C. R. Thomas, Y.-L. Zhao, J. I. Zink and J. F. Stoddart, Acc. Chem. Res., 2011, 44, 903–913 CrossRef CAS PubMed;
(c) R. Barat, T. Legigan, I. Tranoy-Opalinski, B. Renoux, E. Péraudeau, J. Clarhaut, P. Poinot, A. E. Fernandes, V. Aucagne, D. A. Leigh and S. Papot, Chem. Sci., 2015, 6, 2608–2613 RSC.
- Selected examples of interlocked catalysts:
(a) J. Berná, M. Alajarín and R.-A. Orenes, J. Am. Chem. Soc., 2010, 132, 10741–10747 CrossRef PubMed;
(b) V. Blanco, A. Carlone, K. D. Hänni, D. A. Leigh and B. Lewandowski, Angew. Chem., Int. Ed., 2012, 51, 5166–5169 CrossRef CAS PubMed;
(c) C. B. Caputo, K. Zhu, V. N. Vukotic, S. J. Loeb and D. W. Stephan, Angew. Chem., Int. Ed., 2013, 52, 960–963 CrossRef CAS PubMed;
(d) V. Blanco, D. A. Leigh, U. Lewandowska, B. Lewandowski and V. Marcos, J. Am. Chem. Soc., 2014, 136, 15775–15780 CrossRef CAS PubMed;
(e) V. Blanco, D. A. Leigh, V. Marcos, J. A. Morales-Serna and A. L. Nussbaumer, J. Am. Chem. Soc., 2014, 136, 4905–4908 CrossRef CAS PubMed;
(f) J. Beswick, V. Blanco, G. De Bo, D. A. Leigh, U. Lewandowska, B. Lewandowski and K. Mishiro, Chem. Sci., 2015, 6, 140 RSC;
(g) M. Galli, J. E. M. Lewis and S. M. Goldup, Angew. Chem., Int. Ed., 2015, 54, 13545–13549 CrossRef CAS PubMed.
- For selected recent examples of macrocycles for medicinal, materials, catalytic and sensing applications see:
(a) T. Ema, D. Tanida and T. Sakai, J. Am. Chem. Soc., 2007, 129, 10591 CrossRef CAS PubMed;
(b) K. Sato, Y. Itoh and T. Aida, J. Am. Chem. Soc., 2011, 133, 13767 CrossRef CAS PubMed;
(c) S. Dawn, M. B. Dewal, D. Sobransingh, M. C. Paderes, A. C. Wibowo, M. D. Smith, J. A. Krause, P. J. Pellechia and L. S. Shimizu, J. Am. Chem. Soc., 2011, 133, 7032 CrossRef PubMed;
(d) Z. Liu, M. Frasconi, J. Lei, Z. J. Brown, Z. Zhu, D. Cao, J. Iehl, G. Liu, A. C. Fahrenbach, Y. Y. Botros, O. K. Farha, J. T. Hupp, C. A. Mirkin and J. F. Stoddart, Nat. Commun., 2013, 4, 1855 CrossRef PubMed;
(e) A. K. Yudin, Chem. Sci., 2015, 6, 30 RSC.
- For a recent review on the synthesis of macrocycles see: V. Martí-Centelles, M. D. Pandey, M. I. Burguete and S. V. Luis, Chem. Rev., 2015, 115, 8736–8834 CrossRef PubMed.
- For selected examples of the high yielding synthesis of macrocycles using various combinations of these strategies see:
(a) T. Ogoshi, T. Aoki, K. Kitajima, S. Fujinami, T. Yamagishi and Y. Nakamoto, J. Org. Chem., 2011, 76, 328–331 CrossRef CAS PubMed;
(b) S. Guieu, A. K. Crane and M. J. MacLachlan, Chem. Commun., 2011, 47, 1169–1171 RSC;
(c) J. Xia, J. W. Bacon and R. Jasti, Chem. Sci., 2012, 3, 3018–3021 RSC;
(d) S. Lee, C.-H. Chen and A. H. Flood, Nat. Chem., 2013, 5, 704–710 CrossRef CAS PubMed;
(e) A.-C. Bédard, S. Régnier and S. K. Collins, Green Chem., 2013, 15, 1962–1966 RSC;
(f) M. Lisbjerg, B. M. Jessen, B. Rasmussen, B. E. Nielsen, A. Ø. Madsen and M. Pittelkow, Chem. Sci., 2014, 5, 2647–2650 RSC;
(g) T. Boinski, A. Cieszkowski, B. Rosa and A. Szumna, J. Org. Chem., 2015, 80, 3488–3495 CrossRef CAS PubMed;
(h) S. Mangold and R. H. Grubbs, Chem. Sci., 2015, 6, 4561–9 RSC;
(i) C. Kitsiou, J. J. Hindes, P. I'Anson, P. Jackson, T. C. Wilson, E. K. Daly, H. R. Felstead, P. Hearnshaw and W. P. Unsworth, Angew. Chem., Int. Ed., 2015, 54, 15794–15798 CrossRef CAS PubMed.
- For a recent review that discusses the challenges of high dilution synthesis from a medicinal chemistry perspective, including the development of the EMAC measure of synthetic efficiency, see: J. C. Collins and K. James, Med. Chem. Commun., 2012, 3, 1489–1495 RSC.
-
(a) H. Lahlali, K. Jobe, M. Watkinson and S. M. Goldup, Angew. Chem., Int. Ed., 2011, 50, 4151–4155 CrossRef CAS PubMed;
(b) J. Winn, A. Pinczewska and S. M. Goldup, J. Am. Chem. Soc., 2013, 135, 13318–13321 CrossRef CAS PubMed;
(c) R. J. Bordoli and S. M. Goldup, J. Am. Chem. Soc., 2014, 136, 4817–4820 CrossRef CAS PubMed;
(d) E. A. Neal and S. M. Goldup, Chem. Sci., 2015, 6, 2398–2404 RSC.
-
(a) C. W. Tornøe, C. Christensen and M. Meldal, J. Org. Chem., 2002, 67, 3057–3064 CrossRef;
(b) V. V. Rostovtsev, L. G. Green, V. V. Fokin and K. B. Sharpless, Angew. Chem., Int. Ed., 2002, 41, 2596–2599 CrossRef CAS.
-
(a) V. Aucagne, K. D. Hänni, D. A. Leigh, P. J. Lusby and D. B. Walker, J. Am. Chem. Soc., 2006, 128, 2186–2187 CrossRef CAS PubMed;
(b) V. Aucagne, J. Berna, J. D. Crowley, S. M. Goldup, K. D. Hänni, D. A. Leigh, P. J. Lusby, V. E. Ronaldson, A. M. Z. Slawin, A. Viterisi and D. B. Walker, J. Am. Chem. Soc., 2007, 129, 11950–11963 CrossRef CAS PubMed.
- For a review of the AT concept see: J. D. Crowley, S. M. Goldup, A.-L. Lee, D. A. Leigh and R. T. McBurney, Chem. Soc. Rev., 2009, 38, 1530–1541 RSC.
- For selected examples of other AT bond forming reactions and their applications see:
(a) J. D. Crowley, K. D. Hänni, A.-L. Lee and D. A. Leigh, J. Am. Chem. Soc., 2007, 129, 12092–12093 CrossRef CAS PubMed;
(b) J. Berná, J. D. Crowley, S. M. Goldup, K. D. Hänni, A.-L. Lee and D. A. Leigh, Angew. Chem., Int. Ed., 2007, 46, 5709–5757–13 CrossRef PubMed;
(c) J. Berná, S. M. Goldup, A.-L. Lee, D. A. Leigh, M. D. Symes, G. Teobaldi and F. Zerbetto, Angew. Chem., Int. Ed., 2008, 47, 4392–4396 CrossRef PubMed;
(d) J. D. Crowley, K. D. Hänni, D. A. Leigh and A. M. Z. Slawin, J. Am. Chem. Soc., 2010, 132, 5309–5314 CrossRef CAS PubMed;
(e) J. D. Crowley, S. M. Goldup, N. D. Gowans, D. A. Leigh, V. E. Ronaldson and A. M. Z. Slawin, J. Am. Chem. Soc., 2010, 132, 6243–6248 CrossRef CAS PubMed;
(f) S. Saito, E. Takahashi and K. Nakazono, Org. Lett., 2006, 8, 5133–5136 CrossRef CAS PubMed;
(g) N. Weisbach, Z. Baranová, S. Gauthier, J. H. Reibenspies and J. A. Gladysz, Chem. Commun., 2012, 48, 7562–7564 RSC;
(h) M. J. Langton, J. D. Matichak, A. L. Thompson and H. L. Anderson, Chem. Sci., 2011, 2, 1897–1901 RSC;
(i) R. Hayashi, K. Wakatsuki, R. Yamasaki, Y. Mutoh, T. Kasama and S. Saito, Chem. Commun., 2014, 50, 204–206 RSC;
(j) M. Franz, J. A. Januszewski, D. Wendinger, C. Neiss, L. D. Movsisyan, F. Hampel, H. L. Anderson, A. Görling and R. R. Tykwinski, Angew. Chem., Int. Ed., 2015, 54, 6645–6649 CrossRef CAS PubMed;
(k) M. J. Langton, Y. Xiong and P. D. Beer, Chem.–Eur. J., 2015, 21, 18910–18914 CrossRef CAS PubMed.
- Small pyridine macrocycles are also been applied in the synthesis of functionalised [2]rotaxanes using the AT-CuAAC reaction, albeit in lower yield than their bipyridine analogues:
(a) A. Noor, S. C. Moratti and J. D. Crowley, Chem. Sci., 2014, 5, 4283–4290 RSC;
(b) A. Noor, W. K. C. Lo, S. C. Moratti and J. D. Crowley, Chem. Commun., 2014, 50, 7044–7047 RSC.
- S. M. Goldup, D. A. Leigh, P. R. McGonigal, V. E. Ronaldson and A. M. Z. Slawin, J. Am. Chem. Soc., 2010, 132, 315–320 CrossRef CAS PubMed.
- For selected examples of bipyridine macrocycles for other applications see:
(a) E. C. Constable, M. S. Khan, J. Lewis, M. C. Liptrot and P. R. Raithby, Inorg. Chim. Acta, 1991, 181, 207–212 CrossRef CAS;
(b) R. B. Hopkins, J. S. Albert, D. Van Engen and A. D. Hamilton, Bioorg. Med. Chem., 1996, 4, 1121–1128 CrossRef CAS PubMed;
(c) Z. Wang, A. E. Martell, R. J. Motekaitis and J. H. Reibenspies, Inorg. Chim. Acta, 2000, 300–302, 378–383 CrossRef CAS;
(d) A. Bencini, A. Bianchi, C. Giorgi, V. Fusi, A. Masotti and P. Paoletti, J. Org. Chem., 2000, 65, 7686–7689 CrossRef CAS PubMed;
(e) A. Bencini, A. Bianchi, P. Fornasari, C. Giorgi, P. Paoletti and B. Valtancoli, Polyhedron, 2002, 21, 1329–1335 CrossRef CAS;
(f) A. Puglisi, M. Benaglia, R. Annunziata and A. Bologna, Tetrahedron Lett., 2003, 44, 2947–2951 CrossRef CAS;
(g) P.-L. Ng, C.-S. Lee, H.-L. Kwong and A. S. C. Chan, Inorg. Chem. Commun., 2005, 8, 769–772 CrossRef CAS;
(h) C.-S. Lee, P.-F. Teng, W.-L. Wong, H.-L. Kwong and A. S. C. Chan, Tetrahedron, 2005, 61, 7924–7930 CrossRef CAS;
(i) I. Nasso, C. Galaup, F. Havas, P. Tisnès, C. Picard, S. Laurent, L. Vander Elst and R. N. Muller, Inorg. Chem., 2005, 44, 8293–8305 CrossRef CAS PubMed;
(j) G. Bechara, N. Leygue, C. Galaup, B. Mestre and C. Picard, Tetrahedron Lett., 2009, 50, 6522–6525 CrossRef CAS.
- C. Kaes, A. Katz and M. W. Hosseini, Chem. Rev., 2000, 100, 3553–3590 CrossRef CAS PubMed.
- In a homologous series, yields of 31%, 28%, 23% and 12% were obtained for 32-, 30-, 28- and 26-membered rings respectively.14a.
- The Ni-mediated reductive coupling of aryl halides was first reported by Semmelhack and co-workers
(a) M. F. Semmelhack, P. M. Helquist and L. D. Jones, J. Am. Chem. Soc., 1971, 93, 5908–5910 CrossRef CAS For an excellent recent review of Ni-mediated coupling reactions see
(b) B. M. Rosen, K. W. Quasdorf, D. A. Wilson, N. Zhang, A.-M. Resmerita, N. K. Garg and V. Percec, Chem. Rev., 2011, 111, 1346–1416 CrossRef CAS PubMed.
- For selected examples of the Ni-mediated reductive dimerisation of 2-halo-pyridines see:
(a) M. Tiecco, L. Testaferri, M. Tingoli, D. Chianelli and M. Montanucci, Synthesis, 1984, 736–738 CrossRef CAS;
(b) J.-C. Chambron and J.-P. Sauvage, Tetrahedron, 1987, 43, 895–904 CrossRef CAS;
(c) M. Iyoda, H. Otsuka, K. Sato, N. Nisato and M. Oda, Bull. Chem. Soc. Jpn., 1990, 63, 80–87 CrossRef CAS;
(d) N. E. Leadbeater and S. M. Resouly, Tetrahedron Lett., 1999, 40, 4243–4246 CrossRef CAS;
(e) K. W. R. de Franca, M. Navarro, E. Leonel, M. Durandetti and J.-Y. Nedelec, J. Org. Chem., 2002, 67, 1838–1842 CrossRef CAS PubMed;
(f) S. Ishikawa, T. Hamada, K. Manabe and S. Kobayashi, Synthesis, 2005, 2176–2182 CAS;
(g) G. Ricci, R. Ruzziconi and E. Giorgio, J. Org. Chem., 2005, 70, 1011–1018 CrossRef CAS PubMed;
(h) J. Peng, X. Liu and Y. Kishi, Tetrahedron Lett., 2011, 52, 2172–2175 CrossRef CAS;
(i) L. Liao, X. Kong and X. Duan, J. Org. Chem., 2014, 79, 777–782 CrossRef CAS PubMed.
- For medium-ring cyclic bipyridines synthesised under reductive coupling conditions see:
(a) C. Botteghi, A. Schionato and O. De Lucchi, Synth. Commun., 1991, 21, 1819–1823 CrossRef CAS;
(b) B. Milani, E. Alessio, G. Mestroni, E. Zangrando, L. Randaccio and G. Consiglio, J. Chem. Soc., Dalton Trans., 1996, 1021–1029 RSC;
(c) J. Durand, E. Zangrando, C. Carfagna and B. Milani, Dalton Trans., 2008, 2171–2182 RSC;
(d) T. R. Kelly, Y. Lee and R. J. Mears, J. Org. Chem., 1997, 62, 2774–2781 CrossRef CAS PubMed.
- For a recent review of CPP chemistry see: S. E. Lewis, Chem. Soc. Rev., 2015, 44, 2221–2304 RSC.
- For selected examples see:
(a) K. Matsui, Y. Segawa, T. Namikawa, K. Kamada and K. Itami, Chem. Sci., 2013, 4, 84–88 RSC;
(b) K. Matsui, Y. Segawa and K. Itami, J. Am. Chem. Soc., 2014, 136, 16452–16458 CrossRef CAS PubMed;
(c) E. Kayahara, V. K. Patel and S. Yamago, J. Am. Chem. Soc., 2014, 136, 2284–2287 CrossRef CAS PubMed;
(d) Y. Ishii, S. Matsuura, Y. Segawa and K. Itami, Org. Lett., 2014, 16, 2174–2176 CrossRef CAS PubMed;
(e) T. Kuwabara, J. Orii, Y. Segawa and K. Itami, Angew. Chem., Int. Ed., 2015, 54, 9646–9649 CrossRef CAS PubMed;
(f) Y. Segawa, T. Kuwabara, K. Matsui, S. Kawai and K. Itami, Tetrahedron, 2015, 71, 4500–13 CrossRef CAS.
- For a recent review see:
(a) D. C. Harrowven and S. L. Kostiuk, Nat. Prod. Rep., 2012, 29, 223–242 RSC . For selected examples see: ;
(b) M. Iyoda, M. Sakaitani, H. Otsuka and M. Oda, Tetrahedron Lett., 1985, 26, 4777–4780 CrossRef CAS;
(c) K. C. Nicolaou, X.-J. Chu, J. M. Ramanjulu, S. Natarajan, S. Brase, F. Rubsam and C. N. C. Boddy, Angew. Chem., Int. Ed. Engl., 1997, 36, 1539–1540 CrossRef CAS;
(d) A. Carbonnelle, E. G. Zamora, R. Beugelmans and G. Roussi, Tetrahedron Lett., 1998, 39, 4471–4472 CrossRef CAS;
(e) B. H. Lipshutz, P. Müller and D. Leinweber, Tetrahedron Lett., 1999, 40, 3677–3680 CrossRef CAS;
(f) A. Berthelot, S. Piguel, G. Le Dour and J. Vidal, J. Org. Chem., 2003, 68, 9835–9838 CrossRef CAS PubMed;
(g) N. Basse, S. Piguel, D. Papapostolou, A. Ferrier-Berthelot, N. Richy, M. Pagano, P. Sarthou, J. Sobczak-Thépot, M. Reboud-Ravaux and J. Vidal, J. Med. Chem., 2007, 50, 2842–2850 CrossRef CAS PubMed.
- Mn has previously been shown not to undergo direct halogen metal exchange processes under similar conditions. See: D. A. Everson, R. Shrestha and D. J. Weix, J. Am. Chem. Soc., 2010, 132, 920–921 CrossRef CAS PubMed.
- At 50 °C a significant quantity of the dimeric product was isolated and characterised. See ESI†.
- In the case of 2d an excess of half-threads was required. In the case of more rigid, hindered macrocycle 2h a less sterically hindered benzylic azide was required. See ESI for full details†..
- For recent reviews on triazole-based ligands see:
(a) H. Struthers, T. L. Mindt and R. Schibli, Dalton Trans., 2010, 39, 675 RSC;
(b) J. D. Crowley and D. A. McMorran, Top. Heterocycl. Chem., 2012, 28, 31 CAS;
(c) B. Schulze and U. S. Schubert, Chem. Soc. Rev., 2014, 43, 2522 RSC;
(d) J. P. Byrne, J. A. Kitchen and T. Gunnlaugsson, Chem. Soc. Rev., 2014, 43, 5302 RSC.
- For selected examples of pyridyl–triazole coordination complexes see:
(a) Y. Li, J. C. Huffman and A. H. Flood, Chem. Commun., 2007, 2692 RSC;
(b) M. Obata, A. Kitamura, A. Mori, C. Kameyama, J. A. Czaplewska, R. Tanaka, I. Kinoshita, T. Kusumoto, H. Hashimoto, M. Harada, Y. Mikata, T. Funabiki and S. Yano, Dalton Trans., 2008, 3292 RSC;
(c) D. Schweinfurth, K. I. Hardcastle and U. H. F. Bunz, Chem. Commun., 2008, 2203 RSC;
(d) D. Schweinfurth, R. Pattacini, S. Strobel and B. Sarkar, Dalton Trans., 2009, 9291 RSC;
(e) B. Happ, C. Friebe, A. Winter, M. D. Hager, R. Hoogenboom and U. S. Schubert, Chem.–Asian J., 2009, 4, 154 CrossRef CAS PubMed;
(f) K. J. Kilpin, E. L. Gavey, C. J. McAdam, C. B. Anderson, S. J. Lind, C. C. Keep, K. C. Gordon and J. D. Crowley, Inorg. Chem., 2011, 50, 6334 CrossRef CAS PubMed;
(g) T. Y. Kim, A. B. S. Elliott, K. J. Shaffer, J. C. McAdam, K. C. Gordon and J. D. Crowley, Polyhedron, 2013, 52, 1391 CrossRef CAS;
(h) Y. Li, L. Zhao, A. Y.-Y. Tam, K. M.-C. Wong, L. Wu and V. W.-W. Yam, Chem.–Eur. J., 2013, 19, 14496 CrossRef CAS PubMed;
(i) J. P. Byrne, J. A. Kitchen, O. Kotova, V. Leigh, A. P. Bell, J. J. Boland, M. Albrecht and T. Gunnlaugsson, Dalton Trans., 2014, 43, 196 RSC;
(j) J. P. Byrne, J. A. Kitchen, J. E. O'Brien, R. D. Peacock and T. Gunnlaugsson, Inorg. Chem., 2015, 54, 1426 CrossRef CAS PubMed;
(k) N. K. Allampally, C.-G. Daniliuc, C. A. Strassert and L. De Cola, Inorg. Chem., 2015, 54, 1588 CrossRef CAS PubMed.
- Shuttle 9a was isolated in 73% yield in 95% purity. An analytical sample for use in shuttling experiments was obtained by subjecting a portion of this material to a second round of column chromatography.
- The use of CuI and ZnII allows the co-conformational behaviour of 9a and 9e to be monitored readily and in detail by NMR. However, as Sauvage previously reported,3c although the ZnII ion can be removed readily, more forcing conditions (e.g. KCN) are required in the case of CuI. Thus although these systems behave as molecular switches, the reversible switching process itself is somewhat cumbersome. We are currently investigating the in situ electrochemical switching (CuI → CuII) behaviour of such shuttles and these results will be reported in due course.
- C. L. Perrin and T. J. Dwyer, Chem. Rev., 1990, 90, 935–967 CrossRef CAS.
- M. Schmittel, V. Kalsani, R. S. K. Kishore, H. Cölfen and J. W. Bats, J. Am. Chem. Soc., 2005, 127, 11544–11545 CrossRef CAS PubMed.
- C. J. Campbell, D. A. Leigh, I. J. Vitorica-Yrezabal and S. L. Woltering, Angew. Chem., Int. Ed., 2014, 53, 13771–13774 CrossRef CAS PubMed.
Footnote |
† Electronic supplementary information (ESI) available: Full synthetic procedures and characterisation of all novel compounds. CCDC 1442682–1442684. For ESI and crystallographic data in CIF or other electronic format see DOI: 10.1039/c6sc00011h |
|
This journal is © The Royal Society of Chemistry 2016 |
Click here to see how this site uses Cookies. View our privacy policy here.