DOI:
10.1039/C5SC03780H
(Edge Article)
Chem. Sci., 2016,
7, 932-938
Chirality sensing of tertiary alcohols by a novel strong hydrogen-bonding donor – selenourea†
Received
5th October 2015
, Accepted 20th October 2015
First published on 20th October 2015
Abstract
Chemical sensors are powerful for the fast recognition of chiral compounds. However, the established sensing systems are less effective for chiral tertiary alcohols. The chiral tertiary alcohol group is an important structural unit in natural products and drug molecules, and its enantioselective recognition represents a significant and challenging task. In this paper, a novel type of chiral bisselenourea sensor was first synthesized and used as a strong hydrogen-bonding donor for highly efficient chiral recognition of a diverse range of tertiary alcohols. The obtained sharply split NMR signals are well-distinguishable with a large (up to 0.415 ppm) chemical shift nonequivalence. The NMR signal of the hydroxyl hydrogen atom was first employed for enantiomeric excess determination of tertiary alcohols, giving accurate results with <2% absolute errors. The 2D NOESY spectra and computational studies suggest that the geometrical differentiation of the formed diastereomeric complexes between the sensor and tertiary alcohols enables the chiral discrimination of the hydroxyl hydrogen signals of the tertiary alcohol in the 1H NMR spectrum.
Introduction
Chirality plays a key role in modern science and technology. Therefore, the recognition of chirality and enantiomeric excess determination of chiral compounds are intensive areas of research.1 Furthermore, the exponentially growing detection demand in modern asymmetric synthesis and drug discovery drives the development of fast, accurate and convenient systems for chiral recognition. Compared with chiral chromatography and X-ray crystallography, sensing systems using chiral chemical sensors are appropriate for this purpose.2 So far, a lot of chiral sensors have emerged employing CD,1b,3 UV-vis,4 fluorescence2b,5 or NMR spectroscopy6–9 for enantiomeric excess (ee) determination of various types of guests. However, there is one kind of guest, chiral tertiary alcohols, whose enantioselective discrimination has been very challenging until now because of their large steric hindrance, weak coordination ability, and complex stereoelectronic effect.10 The chiral tertiary alcohol group is an important substructure with fully substituted tertiary centers and can be found in many well-known compounds, for example linalool, bedaquiline, camptothecin, escitalopram, efavirenz, etc.11 Chiral recognition of tertiary alcohols is mainly dependent on using time-consuming and expensive chiral chromatography.12 Only very few chiral lanthanide shift reagents13 and chiral solvating agents (CSA)8h–j have been reported as NMR sensors for chiral tertiary alcohols, and the resolved effects were less than satisfactory because of either inherent line broadening6a,8a,14 or a small signal split. Therefore, an accurate and highly efficient chemical sensing system for chiral tertiary alcohols is still highly desirable.
To address this problem, we targeted a chirality sensing system with NMR sensors, employing the proton signal of the hydroxyl group (O–
) attached to the quaternary stereogenic center of a tertiary alcohol. Since the O–H group is involved in intermolecular interactions with CSA via hydrogen bonds, the O–
signal is more easily split. Herein, we report a novel type of sensor, bisselenourea (S,S)-CSA-2 (Fig. 1, right), which could give well-resolved and sharp O–
signals for the enantiodiscrimination of structurally diverse tertiary alcohols using 1H NMR technology.
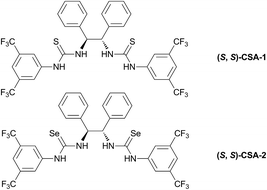 |
| Fig. 1 Structures of bisthiourea and bisselenourea. | |
Results and discussion
Molecular design
A large number of studies has shown that the urea (thiourea) groups of chemical molecules are important recognition sites because they are involved in intermolecular interactions with other molecules via hydrogen bonds.15 In the recognition of chiral compounds, the urea (thiourea) groups primarily act as hydrogen bond donors, and their ability to provide a hydrogen bond is an important factor for the success or failure of the recognition. In general, a urea (thiourea) with a stronger N–H acidity is a better hydrogen bond donor, which is helpful for improving chiral recognition. Accordingly, we developed a CF3-substituted bisthiourea with a strong N–H acidity (Fig. 1, left, (S,S)-CSA-1), which showed excellent enantiodiscrimination for a series of α-carboxylic acids based on split α-H NMR signals.16 However, when used to discriminate several tertiary alcohols, (S,S)-CSA-1 gave much smaller splits of the proton signals due to weak intermolecular interactions. To improve the chiral discrimination of chiral alcohols, we conceived that a stronger hydrogen bond donor than (S,S)-CSA-1 should facilitate the hydrogen-bonding interactions between CSA and tertiary alcohols.
In order to achieve an outstanding performance for the chiral recognition of tertiary alcohols, selenourea attracted our attention. Selenium has similar properties to sulfur with a larger atomic radius. The larger atomic radius makes selenium able to accommodate more negative charge than sulfur, which could induce a stronger N–H acidity of selenoureas.17 Therefore, selenourea should be a stronger hydrogen-bonding donor than thiourea. However, the study of selenourea as a proton donor is limited and data on the N–H acidity of selenourea is not available in literature. Using diphenyl urea, diphenyl thiourea and diphenyl selenourea as model molecules, we found that the N–H pKDMSOa values decrease in the order of diphenyl urea, diphenyl thiourea and diphenyl selenourea, as shown in Table 1 by theoretical calculations. This suggests that selenourea is a stronger hydrogen-bonding donor than thiourea. This result encouraged us to employ selenourea compounds for the chiral recognition of tertiary alcohols. So, we designed and synthesized (S,S)-CSA-2, which is an analog of (S,S)-CSA-1 (Scheme 1). The calculated pKDMSOa value of (S,S)-CSA-2 is significantly less than that of (S,S)-CSA-1 (Table 1). (S,S)-CSA-2 was expected to give a better performance in the chiral recognition of tertiary alcohols than (S,S)-CSA-1.
Table 1 The N–H pKa values of (thio)urea and selenourea
Compound |
pKDMSOa (exptl) |
pKDMSOa (calcd)a |
Calculated pKDMSOa values with the use of the relative determination method. 4-Nitrophenol (10.8) was chosen as the reference acid.
N–H of 3,5-bistrifluormethyl aniline of (S,S)-CSA-1.
N–H of 3,5-bistrifluormethyl aniline of (S,S)-CSA-2.
|
|
18.7 (ref. 18) |
18.5 |
|
13.4 (ref. 18) |
13.8 |
|
— |
11.8 |
(S,S)-CSA-1 |
— |
9.9b |
(S,S)-CSA-2 |
— |
7.5c |
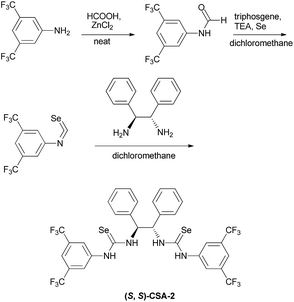 |
| Scheme 1 Synthesis of bisselenourea (S,S)-CSA-2. | |
Test discriminating ability
With (S,S)-CSA-2 in hand, we first compared its enantiomeric discriminating ability with that of (S,S)-CSA-1 by testing several tertiary alcohols under the same experimental conditions (10 mM racemic guest and 10 mM host in CDCl3) (Table 2). As shown in Table 2, (S,S)-CSA-2 does have a better recognition effect than (S,S)-CSA-1, in line with our expectation. This result confirms that replacing S with Se does effectively enhance the acidity of N–H to facilitate the hydrogen-bonding interactions of the alcohols with (S,S)-CSA-2 and improve the chiral recognition. Then, we chose guest A as a model molecule to optimize the discriminating conditions (Table 3). By changing the solvent, a larger (up to 0.052 ppm) ΔΔδ value was obtained with deuterated benzene, implying that a tighter diastereomeric complex could be formed in a more nonpolar solvent, resulting in a better resolution (Table 3, entries 1–4). Increasing the concentrations of guest A and (S,S)-CSA-2 caused the resolution effect to significantly improve because the equilibrium of the diastereomeric complex formation was driven to the right with higher concentrations (Table 3, entries 4–7). The best result was obtained with 30 mM of guest A and 90 mM of (S,S)-CSA-2 (Table 3, entry 9). The corresponding ΔΔδ value is 0.137 ppm, which shows enantiodifferentiation of the enantiomers of the racemic guest A clearly. Therefore, the optimized conditions for our further investigations were chosen as follows: C6D6 as the solvent with 30 mM of the tertiary alcohol guests and 90 mM of bisselenourea (S,S)-CSA-2.
Table 2 Comparison of the discriminating ability of (S,S)-CSA-2 and (S,S)-CSA-1a
Entry |
Guest |
Spectrumb |
ΔΔδc (ppm) |
All samples were prepared by mixing 1 : 1 of CSAs and guests in NMR tubes (10 mM in CDCl3).
Red spectra were obtained with (S,S)-CSA-1, and blue spectra were obtained with (S,S)-CSA-2 by 1H NMR (400 MHz) at 25 °C.
Chemical shift non equivalences (ΔΔδ) of the O– s of guests, red values were obtained with (S,S)-CSA-1, and blue values were obtained with (S,S)-CSA-2.
|
1 |
|
|
0.027 (blue), 0.020 (red) |
2 |
|
|
0.043 (blue), 0.028 (red) |
3 |
|
|
0.022 (blue), 0.012 (red) |
Table 3 Optimization of the discriminating conditions for 2-phenyl-2-butanol (guest A) by (S,S)-CSA-2a
Entry |
Solvent |
C (mM) |
ΔΔδb (ppm) |
Guest A |
(S,S)-CSA-2 |
Guest A and (S,S)-CSA-2 were mixed in the specified solvent (0.6 mL) and the 1H NMR data were collected on a Bruker Avance 400 MHz spectrometer at 25 °C.
ΔΔδ of the O– group of guest A.
|
1 |
CDCl3 |
10 |
10 |
0.027 |
2 |
DMSO |
10 |
10 |
0 |
3 |
CD3COCD3 |
10 |
10 |
0 |
4 |
Benzene |
10 |
10 |
0.052 |
5 |
Benzene |
20 |
20 |
0.074 |
6 |
Benzene |
30 |
30 |
0.097 |
7 |
Benzene |
40 |
40 |
0.108 |
8 |
Benzene |
30 |
60 |
0.125 |
9
|
Benzene
|
30
|
90
|
0.137
|
The applicability of these conditions for racemic alcohols with various structures is demonstrated by the data displayed in Table 4. The ΔΔδ values of the O–
groups are large enough to give a baseline resolution for all the tested tertiary alcohols ranging from simple to multifunctional ones on a 400 MHz NMR instrument at 25 °C (Table 4, entries 1–7). Guest C containing a tetrahydronaphthyl group gave well-distinguishable proton signals with a large (up to 0.415 ppm) ΔΔδ value. Racemic axially chiral BINOL (guest H), containing sp2 C–OH and being structurally similar to tertiary alcohols, could also be effectively enantiodiscriminated by (S,S)-CSA-2 (Table 4, entry 8). Compared with A, although secondary alcohol I lacks one electron-donating ethyl group, its smaller steric hindrance facilitates the intermolecular noncovalent interactions with (S,S)-CSA-2 resulting in a similar ΔΔδ value to A (Table 4, entry 9). Compared with the 0.062 ppm ΔΔδ value of aliphatic secondary alcohol J, the much larger ΔΔδ value (0.144 ppm) of I indicates that pi–pi interactions between the aromatic rings of I and (S,S)-CSA-2 contribute to the chiral discrimination (Table 4 entries 9–10). In addition, (S,S)-CSA-2 has no proton signals in the middle and high fields of its 1H NMR spectrum (see the ESI†), and so it does not interfere with the O–
signals of the guests. Besides 1H NMR spectroscopy, (S,S)-CSA-2 could chiral discriminate tertiary alcohols by other heteronuclear NMR spectroscopy methods. For instance, guests K and L could be clearly resolved by (S,S)-CSA-2 using 19F NMR spectroscopy (guest L is a precursor of efavirenz, an important chiral drug used against HIV) (Table 4, entries 11–12).
Table 4 Measurements of NMR ΔΔδ of racemic guests in the presence of (S,S)-CSA-2a
Entry |
Guest19 |
Spectrumb |
ΔΔδ (ppm) |
All samples were prepared by mixing (S,S)-CSA-2 and the guests in NMR tubes (30 mM of the guest and 90 mM of (S,S)-CSA-2 in 0.6 mL of C6D6). 1H NMR and 19F NMR data were collected on a Bruker Avance 400 MHz spectrometer at 25 °C.
The O– signals of guests A–J and the fluorine signals of guests K and L; the configuration was determined by comparing with the spectra of nonracemic samples of known configurations.
ΔΔδ of the O– of guests A–J.
ΔΔδ of the fluorine atoms of guests K and L.
|
1 |
|
|
0.137c |
2 |
|
|
0.328c |
3 |
|
|
0.415c |
4 |
|
|
0.105c |
5 |
|
|
0.320c |
6 |
|
|
0.144c |
7 |
|
|
0.111c |
8 |
|
|
0.315c |
9 |
|
|
0.144c |
10 |
|
|
0.062c |
11 |
|
|
0.029d |
12 |
|
|
0.046d |
We further investigated the influences of time and temperature on the chiral discrimination of alcohols using guest I as an example. The results show that time has no influence on the chiral discrimination. Lowering the temperature increases the ΔΔδ value but has no effect on the ee value (see the ESI†).
To verify the accuracy of the ee determination method for tertiary alcohols using (S,S)-CSA-2, the ee values of several nonracemic samples of guest F were determined by integration of the O–
signals of guest F. Fig. 2 shows that (S,S)-CSA-2 maintains analytical resolution for the tertiary alcohol F samples over a wide range of ee values. The linear relationship between the NMR determined values (based on the O–
signals) and the HPLC determined values is excellent. The absolute errors in the ee measurements by NMR and HPLC integrations are within 2%.
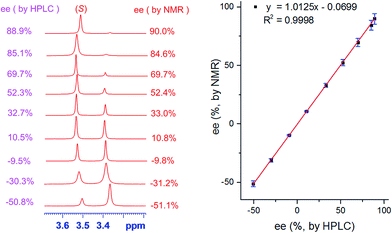 |
| Fig. 2 Selected regions of the 1H NMR spectra of nonracemic guest F (various ee values) with (S,S)-CSA-2 and the linear correlation between the ee values determined by HPLC and NMR (ee values are defined in terms of (S)-F). | |
Molecular recognition modes
To understand the nature of the chiral recognition of (S,S)-CSA-2 for tertiary alcohols, the recognition modes of (S,S)-CSA-2 to guest A were studied. We first investigated the stoichiometry of the host–guest in the complex by Job plots (see the ESI†). The Job plots exhibit a maximum at X = 0.5, indicating 1
:
1 complexation of the host–guest. Then, the binding constants of the (R)-A/(S,S)-CSA-2 complex and (S)-A/(S,S)-CSA-2 complex were determined by the nonlinear least-squares method (see the ESI†). The larger binding constant of (S)-A/(S,S)-CSA-2 suggests that it is a more stable complex than (R)-A/(S,S)-CSA-2 (Table 5).
Table 5 Binding constants between (S,S)-CSA-2 and guest A
Host |
Guest |
K
a
(M−1) |
The Ka values were calculated by the nonlinear least-squares method.
|
(S,S)-CSA-2 |
(R)-A |
12.4 |
(S,S)-CSA-2 |
(S)-A |
26.8 |
2D NOESY experiments for the mixture of racemic guest A and (S,S)-CSA-2 show a strong correlation between guest A and (S,S)-CSA-2 (Fig. 3), indicating that the intermolecular noncovalent interactions present in the complexes result in a closeness of multiple hydrogen atoms (Hk, Hl, Hm, HR or HS) of guest A to (S,S)-CSA-2 (Ha and Hd) in space. Among them, Ha of (S,S)-CSA-2 shows strong NOESY correlated signals with guest A. In addition, its chemical shift dramatically moves downfield (see the ESI†). These results suggest that Ha is more likely to be the intermolecular interaction site.
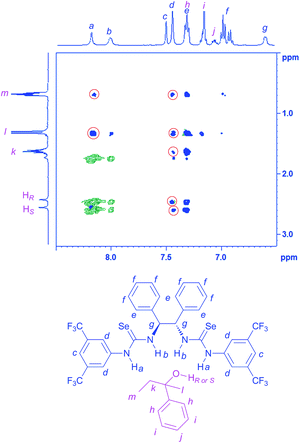 |
| Fig. 3 A portion of the 500 MHz 2D NOESY spectrum of a solution of racemic guest A (100 mM)/(S,S)-CSA-2 (100 mM) in C6D6 at 25 °C (intermolecular correlations of guest A to (S,S)-CSA-2 are circled in red). | |
Computational modeling studies20 show that both (S)-A/(S,S)-CSA-2 and (R)-A/(S,S)-CSA-2 complexes have hydrogen-bonding and pi–pi interactions. As shown in Fig. 4 and Table 6, (S)-A/(S,S)-CSA-2 has a relatively shorter hydrogen bond length, a larger calculated binding energy ΔG and a larger calculated chemical shift of O–
compared to (R)-A/(S,S)-CSA-2, which also indicates that (S)-A/(S,S)-CSA-2 is more stable. We propose that the preference of (S)-A/(S,S)-CSA-2 is due to a weaker steric repulsion between (S)-A and (S,S)-CSA-2. As shown by the optimized structures, in (S)-A/(S,S)-CSA-2, the CH3 group is situated in the bimolecular interacting region, while the larger C2H5 group is far away. By contrast, in (R)-A/(S,S)-CSA-2, the larger C2H5 group is situated in the bimolecular interacting region, which could result in stronger steric repulsion. Therefore, (S)-A/(S,S)-CSA-2 is a sterically preferred complex compared to (R)-A/(S,S)-CSA-2.
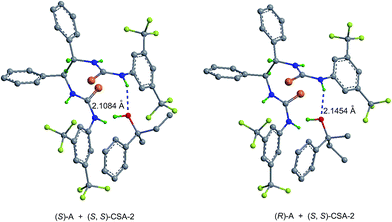 |
| Fig. 4 Space-filling representations of the complexes. | |
Table 6 Calculated δ values of guest A and binding energies between (S,S)-CSA-2 and guest A
Guest |
δ (calcd)a (ppm) |
−ΔG (kcal mol−1) |
The chemical shift of O– of guest A in the guest A/(S,S)-CSA-2 complex.
|
(R)-A |
2.17 |
15.8 |
(S)-A |
2.54 |
18.0 |
On the basis of the above experimental investigations, the mechanism of the chiral discrimination of tertiary alcohol A with (S,S)-CSA-2 was proposed. Each enantiomer of racemic guest A interacts with chiral (S,S)-CSA-2 to form a 1
:
1 diastereomeric complex via noncovalent interactions. The spatial differentiations of the two O–H groups in the formed diastereomeric complexes cause a split of the O–
signals of the two enantiomers of racemic guest A. (S)-A combines with (S,S)-CSA-2 more tightly than (R)-A, which causes the chemical shift of O–
of (S)-A to be moved more downfield with a larger δ value.
Experimental
Materials and methods
Diphenyl urea (TCI Co. Ltd.), diphenyl thiourea (TCI Co. Ltd.), BINOL (TCI Co. Ltd.), 1-phenylethanol (TCI Co. Ltd.), (1R,2R,5R)-(+)-2-hydroxy-3-pinanone (TCI Co. Ltd.), (1S,2S,5S)-(−)-2-hydroxy-3-pinanone (TCI Co. Ltd.), (1S,2R,5S)-(+)-menthol (Adamas-beta®), (1R,2S,5R)-(−)-menthol (Adamas-beta®), 3,5-di(trifluoromethyl)aniline (Adamas-beta®), and (S,S)-1,2-diphenylethane-1,2-diamine (TCI Co. Ltd.) were used as received. Other tertiary alcohols and isoselenocyanate were synthesized by following the literature procedure.21
Theoretical calculations
All electronic structure calculations were performed with the Gaussian09 program.20 The pKDMSOa values were calculated with the use of the relative determination method, and 4-nitrophenol (10.8) was chosen as the reference.18 The gas-phase acidities of all compounds were calculated using the M06-2X/cc-pVTZ method, the solvent effects were evaluated with the IEFPCM model, and the pKa values of all compounds in DMSO were calculated by ΔpKa = ΔG/RT (ΔpKa and ΔG are the pKa and free energy differences between the given compound and the reference molecule). Computational modeling studies were performed with the Gaussian09 program.20 The geometries of the ternary complexes were optimized using the molecular mechanics method initially and then the DFT method at the M06-2X/cc-pVTZ level of theory. All geometry optimizations were carried out in the gas phase. Binding energies and NMR chemical shifts were calculated at the M06-2X/cc-pVTZ level of theory in benzene solvent with the GIAO method (solvent effects were evaluated with the IEFPCM model).
General procedure for the synthesis of (S,S)-CSA-2
3,5-Bis(trifluoromethyl)aniline (10 g, 43.6 mmol) and anhydrous ZnCl2 (1.186 g, 8.7 mmol) were taken in a 50 mL round bottomed flask. To the mixture, formic acid (10 mL, 267 mmol) was added drop-wise with constant stirring for 10 min. This mixture was heated at 70 °C for 5 h and the progress of the reaction was monitored by TLC. When the reaction was completed, the mixture was cooled to room temperature and diluted with ethyl acetate (40 mL). The organic layer was washed with water and brine, and dried over anhydrous Na2SO4. The solvent was removed under reduced pressure and the resulting crude product was purified by recrystallization (in ethanol) to obtain the corresponding N-formyl derivative (10.66 g, 95%, mp 121–122 °C, lit.22 124–125 °C). A solution of triphosgene (692 mg, 2.33 mmol) in dry dichloromethane (5 mL) was added dropwise under Ar over a period of 1 hour to a refluxing mixture of formamide (1 g, 3.89 mmol), TEA (2.25 mL, 15.56 mmol), and 4 A molecular sieves (2 g) in dry dichloromethane (10 mL). After the addition, the resulting mixture was refluxed for 4 hours and then black selenium powder (768 mg, 9.72 mmol) was added and the mixture was refluxed overnight in the dark. Then, the mixture was cooled to room temperature and filtered. The filtrate was collected and concentrated to give a residue, which was purified by column chromatography (eluting with dry hexane) to afford isoselenocyanate (0.49 g, 40%). Caution, this isoselenocyanate is best used directly after separation because it is not very stable and must be stored in the dark.
3,5-Bis(trifluoromethyl)phenyl isoselenocyanate (0.64 g, 2 mmol) was added to a solution of (S,S)-1,2-diphenylethane-1,2-diamine (0.21 g, 1 mmol) in dry dichloromethane (15 mL). The solution was stirred overnight at room temperature in the dark. After completion, dry hexane (100 mL) was added dropwise. The solution was stirred for another 3 hours at room temperature and this produced a lot of white solid, which was filtered off, washed with hexane and dried under vacuum to afford (S,S)-CSA-2 (0.81 g, 95%). Mp 165–166 °C;
= 31.8 g per 100 mL (c = 1.0 in CHCl3). 1H NMR (400 MHz, CDCl3): δ = 8.84 (br s, 2H), 7.95 (br s, 2H), 7.73–7.67 (m, 6H), 7.40–7.20 (m, 10H), 6.17 (s, 2H) ppm; 13C NMR (100 MHz, CDCl3): δ = 179.9, 138.1, 136.4, 133.9, 133.6, 133.2, 132.9, 129.3, 128.9, 127.8, 126.8, 124.8, 124.1, 121.4, 120.7, 118.7, 67.6 ppm; 77Se NMR (95 MHz, CDCl3): δ = 247 ppm; ESI-MS: calcd for C32H22F12N4Se2 + H 851.0062, found 851.0073.
General spectroscopic methods for the chiral discrimination of guests
18 μmol of the guests and 54 μmol of (S,S)-CSA-2 were mixed in 0.6 mL of C6D6, and the 1H NMR data were collected on a Bruker Avance 400 MHz spectrometer at 25 °C.
Conclusions
A chiral bisselenourea was rationally designed and synthesized to serve as a highly efficient NMR sensor for the enantiodiscrimination of various tertiary alcohols. The method employed the sharply split NMR signals of the hydroxyl hydrogen atoms for ee determination, giving accurate results with <2% absolute errors. The study of the chiral resolution mechanism suggests that the geometrical differentiation of the formed diastereomeric complexes enables chiral discrimination of the hydroxyl hydrogen signals of tertiary alcohols in 1H NMR spectra. This method can give well-resolved and sharp signals of the hydroxyl hydrogen atoms of tertiary alcohols with a large chemical shift nonequivalence. Due to the lack of proton signals of this bisselenourea in the middle and high fields of the 1H NMR spectrum, it does not interfere with the hydroxyl hydrogen signals of tertiary alcohols. So, it is especially suitable for chiral discrimination and ee determination of tertiary alcohols.
Acknowledgements
The authors gratefully acknowledge the financial support from The Key Laboratory of Coal to Ethylene Glycol and Its Related Technology, National Natural Science Foundation of China (No. 21173212, 21207131), and Fujian Institute of Research on the Structure of Matter, Chinese Academy of Sciences.
Notes and references
-
(a) Z. Huang, S. Yu, K. Wen, X. Yu and L. Pu, Chem. Sci., 2014, 5, 3457–3462 RSC;
(b) P. Metola, E. V. Anslyn, T. D. James and S. D. Bull, Chem. Sci., 2012, 3, 156–161 RSC;
(c) T. Miyabe, H. Iida, A. Ohnishi and E. Yashima, Chem. Sci., 2012, 3, 863–867 RSC;
(d) W. Wei, K. Qu, J. Ren and X. Qu, Chem. Sci., 2011, 2, 2050–2056 RSC.
-
(a) X. Zhang, J. Yin and J. Yoon, Chem. Rev., 2014, 114, 4918–4959 CrossRef CAS PubMed;
(b) L. Pu, Acc. Chem. Res., 2012, 45, 150–163 CrossRef CAS PubMed;
(c) G. A. Hembury, V. V. Borovkov and Y. Inoue, Chem. Rev., 2008, 108, 1–73 CrossRef CAS PubMed.
-
(a) H. H. Jo, C.-Y. Lin and E. V. Anslyn, Acc. Chem. Res., 2014, 47, 2212–2221 CrossRef CAS PubMed;
(b) C. Wolf and K. W. Bentley, Chem. Soc. Rev., 2013, 42, 5408–5424 RSC.
-
(a) X. Mei and C. Wolf, J. Am. Chem. Soc., 2006, 128, 13326–13327 CrossRef CAS PubMed;
(b) P. Zhang and C. Wolf, Chem. Commun., 2013, 49, 7010–7012 RSC.
-
(a) K. Wen, S. Yu, Z. Huang, L. Chen, M. Xiao, X. Yu and L. Pu, J. Am. Chem. Soc., 2015, 137, 4517–4524 CrossRef CAS PubMed;
(b) C. Wang, E. Wu, X. Wu, X. Xu, G. Zhang and L. Pu, J. Am. Chem. Soc., 2015, 137, 3747–3750 CrossRef CAS PubMed;
(c) T. A. Feagin, D. P. V. Olsen, Z. C. Headman and J. M. Heemstra, J. Am. Chem. Soc., 2015, 137, 4198–4206 CrossRef CAS PubMed;
(d) K. W. Bentley and C. Wolf, J. Am. Chem. Soc., 2013, 135, 12200–12203 CrossRef CAS PubMed.
- For selected reviews on chiral discrimination using NMR sensors:
(a) D. Parker, Chem. Rev., 1991, 91, 1441–1457 CrossRef CAS;
(b)
T. J. Wenzel, Discrimination of chiral compounds using NMR spectroscopy, Wiley, 2007 Search PubMed;
(c) T. J. Wenzel and C. D. Chisholm, Prog. Nucl. Magn. Reson. Spectrosc., 2011, 59, 1–63 CrossRef CAS PubMed;
(d) G. Uccello-Barretta, F. Balzano and P. Salvadori, Curr. Pharm. Des., 2006, 12, 4023–4045 CrossRef CAS PubMed;
(e) J. Labuta, J. P. Hill, S. Ishihara, L. Hanykova and K. Ariga, Acc. Chem. Res., 2015, 48, 521–529 CrossRef CAS PubMed.
- For selected publications on chiral NMR sensors for carboxylic acids:
(a) S. K. Mishra and N. Suryaprakash, RSC Adv., 2015, 5, 67277–67283 RSC;
(b) H.-T. Feng, X. Zhang and Y.-S. Zheng, J. Org. Chem., 2015, 80, 8096–8101 CrossRef CAS PubMed;
(c) A. E. Sheshenev, E. V. Boltukhina, A. A. Grishina, I. Cisarova, I. M. Lyapkalo and K. K. Hii, Chem.–Eur. J., 2013, 19, 8136–8143 CrossRef CAS PubMed;
(d) M. Perez-Trujillo, E. Monteagudo and T. Parella, Anal. Chem., 2013, 85, 10887–10894 CrossRef CAS PubMed;
(e) D. Kumari, P. Bandyopadhyay and N. Suryaprakash, J. Org. Chem., 2013, 78, 2373–2378 CrossRef CAS PubMed;
(f) S. R. Chaudhari and N. Suryaprakash, New J. Chem., 2013, 37, 4025–4030 RSC;
(g) F. Cuevas, P. Ballester and M. A. Pericas, Org. Lett., 2005, 7, 5485–5487 CrossRef CAS PubMed;
(h) D. Yang, X. Li, Y.-F. Fan and D.-W. Zhang, J. Am. Chem. Soc., 2005, 127, 7996–7997 CrossRef CAS PubMed;
(i) A. Gualandi, S. Grilli, D. Savoia, M. Kwit and J. Gawronski, Org. Biomol. Chem., 2011, 9, 4234–4241 RSC;
(j) M. Hernandez-Rodriguez and E. Juaristi, Tetrahedron, 2007, 63, 7673–7678 CrossRef CAS;
(k) Q. Ma, M. Ma, H. Tian, X. Ye, H. Xiao, L.-h. Chen and X. Lei, Org. Lett., 2012, 14, 5813–5815 CrossRef CAS PubMed;
(l) T. P. Quinn, P. D. Atwood, J. M. Tanski, T. F. Moore and J. F. Folmer-Andersen, J. Org. Chem., 2011, 76, 10020–10030 CrossRef CAS PubMed;
(m) M. Durmaz, M. Yilmaz and A. Sirit, Org. Biomol. Chem., 2011, 9, 571–580 RSC.
- For selected publications on chiral NMR sensors for alcohols:
(a) L. M. Sweeting, D. C. Crans and G. M. Whitesides, J. Org. Chem., 1987, 52, 2273–2276 CrossRef CAS;
(b) I. Pal, S. R. Chaudhari and N. R. Suryaprakash, Magn. Reson. Chem., 2015, 53, 142–146 CrossRef CAS PubMed;
(c) C. Rosini, G. Uccello-Barretta, D. Pini, C. Abete and P. Salvadori, J. Org. Chem., 1988, 53, 4579–4581 CrossRef CAS;
(d) G. Uccello-Barretta, D. Pini, A. Mastantuono and P. Salvadori, Tetrahedron: Asymmetry, 1995, 6, 1965–1972 CrossRef CAS;
(e) d. B.-H. C. von, A. K. Beck, U. Lengweiler and D. Seebach, Helv. Chim. Acta, 1992, 75, 438–441 CrossRef;
(f) S. H. Wilen, J. Z. Qi and P. G. Williard, J. Org. Chem., 1991, 56, 485–487 CrossRef CAS;
(g) L. S. Moon, M. Pal, Y. Kasetti, P. V. Bharatam and R. S. Jolly, J. Org. Chem., 2010, 75, 5487–5498 CrossRef CAS PubMed;
(h) L. S. Moon, R. S. Jolly, Y. Kasetti and P. V. Bharatam, Chem. Commun., 2009, 1067–1069 RSC;
(i) C. Wolf, A. M. Cook and J. E. Dannatt, Tetrahedron: Asymmetry, 2014, 25, 163–169 CrossRef CAS;
(j) A. Iuliano, G. Uccello-Barretta and P. Salvadori, Tetrahedron: Asymmetry, 2000, 11, 1555–1563 CrossRef CAS.
- For selected publications on chiral NMR sensors for various chiral compounds:
(a) C. F. Dignam, J. J. Zopf, C. J. Richards and T. J. Wenzel, J. Org. Chem., 2005, 70, 8071–8078 CrossRef CAS PubMed;
(b) A. Lakshmipriya, S. R. Chaudhari and N. Suryaprakash, Chem. Commun., 2015, 51, 13492–13495 RSC;
(c) N. Jain, R. B. Patel and A. V. Bedekar, RSC Adv., 2015, 5, 45943–45955 RSC;
(d) I. Pal, S. R. Chaudhari and N. Suryaprakash, New J. Chem., 2014, 38, 4908–4912 RSC;
(e) A. Couffin, O. Thillaye du Boullay, M. Vedrenne, C. Navarro, B. Martin-Vaca and D. Bourissou, Chem. Commun., 2014, 50, 5997–6000 RSC;
(f) T. Ema, D. Tanida and T. Sakai, J. Am. Chem. Soc., 2007, 129, 10591–10596 CrossRef CAS PubMed;
(g) T. Ema, D. Tanida and T. Sakai, Org. Lett., 2006, 8, 3773–3775 CrossRef CAS PubMed;
(h) G. Uccello-Barretta, A. Iuliano, E. Franchi, F. Balzano and P. Salvadori, J. Org. Chem., 1998, 63, 9197–9203 CrossRef CAS;
(i) R. Schwenninger, J. Schlogl, J. Maynollo, K. Gruber, P. Ochsenbein, H.-B. Burgi, R. Konrat and B. Krautler, Chem.–Eur. J., 2001, 7, 2676–2686 CrossRef CAS PubMed;
(j) M. Perez-Trujillo, I. Maestre, C. Jaime, A. Alvarez-Larena, J. F. Piniella and A. Virgili, Tetrahedron: Asymmetry, 2005, 16, 3084–3093 CrossRef CAS;
(k) A. Port, A. Virgili, A. Alvarez-Larena and J. F. Piniella, Tetrahedron: Asymmetry, 2000, 11, 3747–3757 CrossRef CAS;
(l) G. Uccello-Barretta, F. Mirabella, F. Balzano and P. Salvadori, Tetrahedron: Asymmetry, 2003, 14, 1511–1516 CrossRef CAS;
(m) S. Tabassum, M. A. Gilani and R. Wilhelm, Tetrahedron: Asymmetry, 2011, 22, 1632–1639 CrossRef CAS;
(n) J. Omelańczuk and M. Mikolajczyk, Tetrahedron: Asymmetry, 1996, 7, 2687–2694 CrossRef;
(o) Y. Zhao and T. M. Swager, J. Am. Chem. Soc., 2015, 137, 3221–3224 CrossRef CAS PubMed.
- A. Alexakis, S. Mutti and P. Mangeney, J. Org. Chem., 1992, 57, 1224–1237 CrossRef CAS.
-
(a) M. Mueller, ChemBioEng Rev., 2014, 1, 14–26 CrossRef CAS;
(b) P. G. Cozzi, R. Hilgraf and N. Zimmermann, Eur. J. Org. Chem., 2007, 2007, 5969–5994 CrossRef;
(c) C. Garcia and V. S. Martin, Curr. Org. Chem., 2006, 10, 1849–1889 CrossRef CAS;
(d) S. V. Pronin, C. A. Reiher and R. A. Shenvi, Nature, 2013, 501, 195–199 CrossRef CAS PubMed.
-
(a) N. M. Maier and G. Uray, J. Chromatogr. A, 1996, 732, 215–230 CrossRef CAS;
(b) A. Kunath, E. Hoeft, H. J. Hamann and J. Wagner, J. Chromatogr. A, 1991, 588, 352–355 CrossRef CAS;
(c) B. Koppenhoefer and H. Allmendinger, Chromatographia, 1986, 21, 503–508 CrossRef CAS.
-
(a) M. D. Johnston Jr, B. L. Shapiro, M. J. Shapiro, T. W. Proulx, A. D. Godwin and H. L. Pearce, J. Am. Chem. Soc., 1975, 97, 542–554 CrossRef;
(b) W. Huang and L. Zhang, Acta Chim. Sin., 1989, 7, 183–189 CrossRef;
(c) M.-C. Blanc, P. Bradesi and J. Casanova, Magn. Reson. Chem., 2005, 43, 176–179 CrossRef CAS PubMed.
-
(a) H. C. Aspinall, Chem. Rev., 2002, 102, 1807–1850 CrossRef CAS PubMed;
(b) R. E. Lenkinski and J. Reuben, J. Magn. Reson., 1976, 21, 47–56 CAS.
-
(a) Z. Zhang and P. R. Schreiner, Chem. Soc. Rev., 2009, 38, 1187–1198 RSC;
(b) A.-F. Li, J.-H. Wang, F. Wang and Y.-B. Jiang, Chem. Soc. Rev., 2010, 39, 3729–3745 RSC;
(c) T. R. Kelly and M. H. Kim, J. Am. Chem. Soc., 1994, 116, 7072–7080 CrossRef CAS.
-
(a) G. Bian, H. Fan, S. Yang, H. Yue, H. Huang, H. Zong and L. Song, J. Org. Chem., 2013, 78, 9137–9142 CrossRef CAS PubMed;
(b) G. Bian, H. Fan, H. Huang, S. Yang, H. Zong, L. Song and G. Yang, Org. Lett., 2015, 17, 1369–1372 CrossRef CAS PubMed.
- F. G. Bordwell, D. J. Algrim and J. A. Harrelson, J. Am. Chem. Soc., 1988, 110, 5903–5904 CrossRef CAS.
- G. Jakab, C. Tancon, Z. Zhang, K. M. Lippert and P. R. Schreiner, Org. Lett., 2012, 14, 1724–1727 CrossRef CAS PubMed.
- Guest (R)-F is commercial (1R,2R,5R)-(+)-2-hydroxy-3-pinanone, (S)-F is commercial (1S,2S,5S)-(−)-2-hydroxy-3-pinanone, D–K is commerical (1S,2R,5S)-(+)-Menthol and L–K is commercial (1R,2S,5R)-(−)-Menthol.
-
M. J. T. G. W. Frisch, H. B. Schlegel, G. E. Scuseria, M. A. Robb, J. R. Cheeseman, G. Scalmani, V. Barone, B. Mennucci, G. A. Petersson, H. Nakatsuji, M. Caricato, X. Li, H. P. Hratchian, A. F. Izmaylov, J. Bloino, G. Zheng, J. L. Sonnenberg, M. Hada, M. Ehara, K. Toyota, R. Fukuda, J. Hasegawa, M. Ishida, T. Nakajima, Y. Honda, O. Kitao, H. Nakai, T. Vreven JrJ. A. Montgomery, J. E. Peralta, F. Ogliaro, M. Bearpark, J. J. Heyd, E. Brothers, K. N. Kudin, V. N. Staroverov, R. Kobayashi, J. Normand, K. Raghavachari, A. Rendell, J. C. Burant, S. S. Iyengar, J. Tomasi, M. Cossi, N. Rega, J. M. Millam, M. Klene, J. E. Knox, J. B. Cross, V. Bakken, C. Adamo, J. Jaramillo, R. Gomperts, R. E. Stratmann, O. Yazyev, A. J. Austin, R. Cammi, C. Pomelli, J. W. Ochterski, R. L. Martin, K. Morokuma, V. G. Zakrzewski, G. A. Voth, P. Salvador, J. J. Dannenberg, S. Dapprich, A. D. Daniels, Ö. Farkas, J. B. Foresman, J. V. Ortiz, J. Cioslowski and D. J. Fox, Gaussian 09, Revision A.1, Gaussian, Inc., Wallingford CT, 2009 Search PubMed.
- Ó. López, S. Maza, V. Ulgar, I. Maya and J. G. Fernández-Bolaños, Tetrahedron, 2009, 65, 2556–2566 CrossRef.
- G. Pettit, M. Kalnins, T. Liu, E. Thomas and K. Parent, J. Org. Chem., 1961, 26, 2563–2566 CrossRef CAS.
Footnote |
† Electronic supplementary information (ESI) available: Original NMR spectra and the results of the computational modeling. See DOI: 10.1039/c5sc03780h |
|
This journal is © The Royal Society of Chemistry 2016 |