DOI:
10.1039/C5SC03732H
(Edge Article)
Chem. Sci., 2016,
7, 2427-2436
Selective capture of hexavalent chromium from an anion-exchange column of metal organic resin–alginic acid composite†
Received
1st October 2015
, Accepted 18th November 2015
First published on 18th November 2015
Abstract
We report an anion exchange composite material based on a protonated amine-functionalized metal–organic framework, denoted Metal Organic Resin-1 (MOR-1), and alginic acid (HA). MOR-1–HA material shows an exceptional capability to rapidly and selectively sorb Cr(VI) under a variety of conditions and in the presence of several competitive ions. The selectivity of MOR-1–HA for Cr(VI) is shown to be the result of strong O3CrVI⋯NH2 interactions. The composite sorbent can be successfully utilized in an ion-exchange column, in contrast to pristine MOR-1 which forms fine suspensions in water passing through the column. Remarkably, an ion exchange column with only 1% wt MOR-1–HA and 99% wt sand (an inert and inexpensive material) is capable of reducing moderate and trace Cr(VI) concentrations to well below the acceptable safety limits for water. The relatively low cost of MOR-1–HA/sand column and its high regeneration capability and reusability make it particularly attractive for application in the remediation of Cr(VI)-bearing industrial waste.
Introduction
The contamination of water resources by toxic species represents a major cause of global concern. Among the most commonly found pollutants are various oxo-hydroxy anions.1 A characteristic example is Cr(VI)-oxo species, found as dichromate (Cr2O72−), hydrogen chromate (HCrO4−) or chromate (CrO42−) ions depending on the acidity/basicity of water. Cr(VI) is a well-known carcinogen, which is released to the environment from leather-tanning, cement, metal plating, dyes industries etc.2 Commonly used and inexpensive methods such as precipitation of the ions from solution are not sufficiently effective to lower the concentration of Cr(VI) below the acceptable limits3a,b and they also generate large amounts of metal-containing sludge.3c Reduction of Cr(VI) to Cr(III) is a promising method, but presents some limitations such as generation of high concentrations of chromium(III) ions in the solution (a secondary treatment of the solution is then needed) or formation of solid waste (e.g. Cr(OH)3).4 Absorption and ion exchange are considered as highly effective and relatively inexpensive methods for the treatment of Cr(VI)-containing waste.5 Several sorbents have been tested for this purpose. Organic resins, containing functional groups suitable for binding of specific cations or anions, are the most widely-used sorbents in various remediation processes and in the purification of drinking water.5 Commercially available resins, with amine-functional groups, have shown promising Cr(VI) sorption properties.6 Such materials, however, are not only of relatively high cost, but also absorb Cr(VI) through reduction–precipitation of Cr(III) species. Thus, such resins are actually oxidized–decomposed by Cr(VI) and they cannot be regenerated and reused. Furthermore, some tests indicated that these Cr(III)-loaded resins are not safe for disposal as non-hazardous solid waste due to significant Cr leaching.6 On the other hand, layered double hydroxides (LDHs), the typical inorganic anionic exchangers, are inexpensive, but show relatively slow sorption kinetics for Cr(VI) and limited selectivity in the presence of competitive ions.7
Metal–organic frameworks (MOFs),8 decorated with organic groups having strong binding affinity for toxic ions, may be ideal sorbents for various remediation processes. Such functionalized materials, which can be prepared with facile methods and on a large scale, can be called metal–organic resins (MORs), since they combine the organic functionalities of the amorphous organic resins (strong binding groups)5 and the ordered porous structure of crystalline MOFs. Thus, MORs with a combination of functional-group based selectivity and a highly porous structure with defined pore size distribution, promise fast sorption kinetics and high efficiencies in practical separation processes. So far, there are limited reports on cationic metal–organic materials showing sorption capability for Cr(VI).9 These examples show efficient Cr(VI) ion exchange capacity. However, all reported materials have been tested with batch methods and no studies on their use in columns have been carried out. Note that industrial wastewater processes require continuous bed flow ion exchange columns. A sorbent material, in order to be appropriate for use in such columns, should display (a) high selectivity and fast sorption kinetics for the targeted ion, (b) particle size suitable to allow continuous flow of wastewater through the column and (c) good mechanical strength to withstand high water pressures.10MORs and other porous materials are usually characterized by very small particle size and insufficient mechanical strength, which hinder their use in columns. Therefore, as-prepared MORs are not suitable for practical environmental remediation applications and thus, novel approaches are required in order to produce an engineered form of the materials to fulfil the requirements of column testing.
Herein we describe the anion exchange composite material based on the [Zr6O4(OH)4(NH3+-BDC)6]Cl6MOR (MOR-1) and alginic acid (HA) polymer. The MOR is the analogue of the UiO-66 material containing NH3+ functional groups (Fig. 1).11 Through detailed batch studies, the highly efficient and selective anion exchange properties of the composite for Cr(VI) are revealed. Importantly, for the first time, we demonstrate the successful use of MORs, in the form of MOR–HA composite, in an ion exchange column. The stationary phase in this column is a mixture of MOR-1–HA and sand (an inert and inexpensive material). Remarkably, a column with MOR-1–HA/sand stationary phase containing only 1% wt MOR-1–HA is capable to reduce moderate and trace levels of Cr(VI) well below the allowed safe levels defined by the USA-EPA (100 ppb)3a and EU (50 ppb),3b despite the presence of a large excess of competitive ions (Cl−, Br−, NO3−, SO42−etc.). Furthermore, the column can be easily regenerated and reused several times with almost no loss of its capacity. The efficiency and simplicity of this ion exchange column make it attractive for use in the decontamination of wide variety of Cr(VI)-containing wastes.
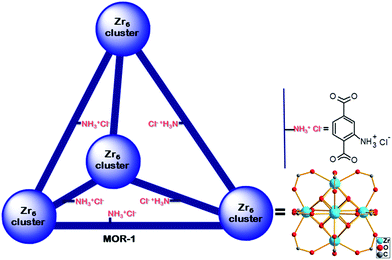 |
| Fig. 1 Representation of the structure of (protonated) MOR-1 material shown as a tetrahedral cage (based on the structure of UiO-66 material11). | |
Results and discussion
Synthesis of MOR-1–HA composite
Zr4+MORs of the UiO-66 family are very promising materials for sorption applications due to their high surface areas, easy incorporation of functional groups and hydrolytic-thermal stability.11 However, as mentioned above, as prepared MORs as fine powders are not suitable for practical ion exchange applications. This is particularly true for UiO-66 type MORs usually isolated as nanoparticles (100–200 nm).11g The latter is a major drawback for the application of such materials as stationary phases in columns. To this end, we applied the alginate encapsulation method to prepare UiO-66 type-composite solids.12 This encapsulation method (method A) involves (a) addition of the sorbent to be encapsulated (i.e.MOR) into an aqueous solution of sodium alginate (SA) so that one or more monolayers of alginate-saturated water cover each particle of the sorbent and (b) addition of CaCl2 to the SA-sorbent suspension so that the monolayer is immediately converted to calcium alginate (CA), forming a water-insoluble polymer shell around the sorbent particulates (Fig. 2). Then, the MOR-1–CA material was treated with hydrochloric acid to produce [Zr6O4(OH)4(NH3+-BDC)6]Cl6–HA (MOR-1–HA) (HA = alginic acid) composite with protonated amine groups and extra-framework easily exchangeable Cl− anions (see below), Fig. 2. Note that only 4% wt of alginate (i.e. alginate
:
MOR-1 mass ratio used was ∼0.04) is sufficient for the composite to be formed and thus, MOR is not encapsulated by thick HA particles that would hinder the diffusion of ions into the MOR pores. Alternatively, MOR-1–HA composite may be prepared directly by adding HCl into a suspension of MOR-1 in SA aqueous solution (method B). The material synthesized with this method shows almost identical structural characteristics and ion-exchange properties with those of composite prepared with method A, as revealed by their very similar PXRD, IR and Cr(VI) ion-exchange isotherm data (see ESI†). However, for all studies described below, composite samples isolated with method A have been used.
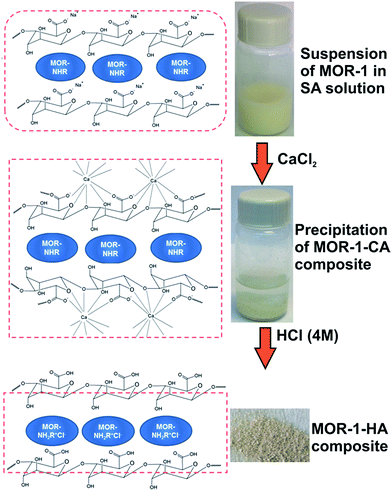 |
| Fig. 2 Schematics for the preparation of the MOR-1–HA composite. | |
Due to the presence of the HA shell covering the MOR particles, the composite MOR-1–HA material is not easily dispersed in water, in contrast to the pure MOR-1 which forms a fine suspension upon mixing it with water (Fig. 3). This feature of the composite material is proved to be key for its successful utilization in ion-exchange columns (see below).
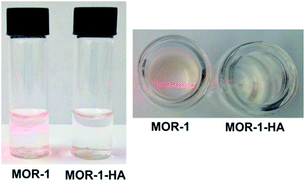 |
| Fig. 3 Upon mixing MOR-1 with water a fine suspension is immediately formed (left vial), whereas MOR-1–HA is clearly separated from water (right vial). This is confirmed by the illumination of the mixtures with a laser pointer. Thus, the laser beam can be easily observed as it travels through the liquid in the left vial (Tyndall effect), whereas it is invisible when it travels through the clear solution in the right vial. | |
Characterization of MOR-1–HA composite
Scanning electron microscopy (SEM) images of MOR-1–HA and MOR-1 particles indicate similar morphological characteristics for these materials (Fig. S1, ESI†). EDS analysis for the MOR-1–HA sample indicates a Zr
:
Cl ratio of ∼1, which is in agreement with the protonation of the six amino-groups of the Zr6 cluster and the presence of six Cl− counter ions. Thermogravimetric analysis (TGA) was used for the determination of the lattice water molecules (21 H2O molecules, Fig. S2†). Powder X-ray diffraction (PXRD) data indicate that the MOR retains its structure in the composite form (Fig. 4A). The Brunauer–Emmett–Teller (BET) surface area of the MOR-1–HA was determined as 1004 m2 g−1 (Fig. 4B), a value within the range of surface areas found for amino-functionalized UiO-66 materials.11b,c Analysis of CO2 adsorption data with density function theory (DFT) gives a pore size of about 7 Å, (Fig. S3†).
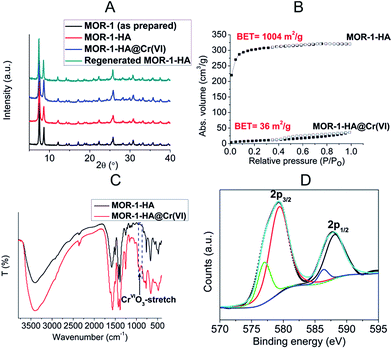 |
| Fig. 4 (A) PXRD patterns of MOR-1 (as prepared), MOR-1–HA, MOR-1–HA@Cr(VI) and regenerated MOR-1–HA. (B) Nitrogen adsorption–desorption isotherms at 77 K for MOR-1–HA and MOR-1–HA@Cr(VI). (C) IR spectra of MOR-1–HA and MOR-1–HA@Cr(VI). (D) High-resolution Cr 2p1/2 and Cr 2p3/2 core-level photoelectron spectra of MOR-1–HA with their deconvolution into two components. The minor signals with binding energy at 586.3 and 577.0 eV are attributed to Cr(III) traces resulted from the known reduction effects under X-ray irradiation.14 | |
Isolation and characterization of Cr(VI)-loaded material
Detailed Cr(VI) sorption studies for MOR-1–HA were performed at low pH (pH ∼ 3), in order to model the usual acidic conditions of Cr(VI) industrial waste (for example tannery and metal plating wastewater).13 Under such conditions, the predominant Cr(VI) species is Cr2O72− (with some contribution from HCrO4− at dilute solutions).2b
By immersing the MOR-1–HA material in a Cr2O72− solution, the removal of Cr2O72− is accomplished at a very fast rate (within a few minutes), an event that can be visually observed by the decolouration of the solution and colour change of the sorbent (Fig. S4†). This ion exchange process is described by the following equation:
| [Zr6O4(OH)4(NH3+-BDC)6]Cl6–HA + 3Cr2O72− → [Zr6O4(OH)4(NH3+-BDC)6](Cr2O7)3–HA + 6Cl− | (1) |
EDS data revealed no Cl− anions in the Cr(VI)-loaded material MOR-1–HA@Cr(VI). ICP-MS, EDS and UV-Vis data (see below) indicate a Zr
:
Cr ratio of 0.9–1.2, closed to the expected one (theoretical Zr
:
Cr = 1, considering the insertion of 3Cr2O72− per Zr6 cluster). PXRD data revealed that the MOR structure is retained after the incorporation of the dichromate anions (Fig. 3A).
There is a drastic decrease, however, in the BET surface area for MOR-1–HA@Cr(VI) indicating the pores of the structure are filled by Cr(VI) species. Specifically, after the insertion of Cr2O72− anions, the surface area for MOR-1–HA drops from ∼1000 to 36 m2 g−1 (Fig. 4B). Further evidence for the presence of Cr(VI) species in MOR-1–HA@Cr(VI) was provided by infrared (IR) and X-ray photoelectron spectroscopy (XPS). The IR spectrum (Fig. 4C and S5†) of the exchanged sample showed the existence of an absorption peak at ∼924 cm−1 (not present in the spectra of pristine MOR-1–HA material) assigned to the antisymmetric CrVIO3-stretch (for more detailed interpretation of IR data see also below). XPS data revealed the presence of Cr
2p1/2 and Cr
2p3/2 core-level signals, with their main components corresponding to binding energies of 588.1 and 579.3 eV (Fig. 4D). These binding energies are consistent with Cr(VI).14
Batch ion exchange studies
Ion-exchange equilibrium data.
To gain further insight into the Cr2O72− sorption properties of the MOR-1–HA material, we first performed batch ion exchange studies. The Cr2O72− ion exchange equilibrium data for MOR-1–HA composite are shown graphically in Fig. 5. The description of the data can be provided by the Langmuir model (fitting also can be done with the Freundlich model, see ESI Fig. S6 and Table S1†).15 The sorption capacity is 242 ± 17 mg Cr2O72− per g of MOR-1–HA (or 242/0.96–252 mg g−1 of MOR-1, considering that MOR-1–HA contains 96% wt of MOR-1), which is well-comparable with the capacities of the best Cr(VI) sorbents.7,9 This sorption capacity is consistent with the absorption of ∼2.7 ± 0.2 moles of Cr2O72− per formula unit of the MOR-1, which is close to the expected maximum sorption capacity of the material (3.0 mol per formula unit). The affinity of the MOR-1–HA for dichromate can be expressed in terms of the distribution coefficient Kd which is given by the equation | 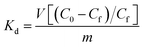 | (2) |
where C0 and Cf are the initial and equilibrium concentration of Cr2O72− (ppm), respectively, V is the volume (mL) of the testing solution and m is the amount of the ion exchanger (g) used in the experiment.15 Values for Kd equal to or above 104 L g−1 are considered excellent. The maximum
values for the MOR-1–HA, obtained from the batch equilibrium studies, are in the range of (1.2–5.5) × 104 L g−1, which reveal the exceptional affinity of this material for dichromate ions. We should also note that MOR-1–HA samples loaded with Cr2O72− can be easily regenerated by treating them with concentrated HCl solutions (1.2–4 M). The PXRD pattern of the regenerated MOF-1–HA is almost identical with that of as prepared MOR-1–HA material (Fig. 4A). The regenerated MOR-1–HA showed similar dichromate exchange capacity (230–240 mg g−1) as that of pristine material (more detailed regeneration studies were performed for the ion exchange columns, see below).
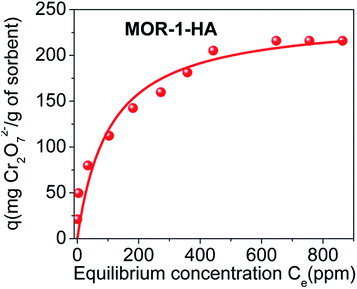 |
| Fig. 5 Equilibrium Cr2O72−sorption data for MOR-1–HA material (pH ∼ 3). The solid line represents the fitting of the data with the Langmuir model. | |
Kinetic studies.
The kinetics of the Cr2O72− exchange of the MOR-1–HA composite was also studied. The results indicate that the capture of Cr2O72− by the composite was remarkably fast, as indicated by UV-Vis and ICP-MS data (Fig. 6). Within only 1 min of solution/composite contact, ∼94.2% of the initial Cr2O72− amount (C0 = 21.6 ppm, pH ∼ 3) was removed by the solution. After 3 min of solution/composite contact, the Cr(VI) ion exchange almost reached its equilibrium with ∼97.5% removal capacity. These kinetic data can be fitted (Fig. 6B, inset) with the Lagergren's first-order equation | qt = qe[1 − exp(−KLt)], | (3) |
where qe = the amount (mg g−1) of metal ion absorbed in equilibrium, KL = the Lagergren or first-order rate constant (fitting parameters: qe = 21.1 ± 0.4 mg g−1, KL = 3.3 ± 0.1 min−1, R2 = 0.90).16 From these data, it is clear that the ordered highly porous structure of MOR-1–HA facilitating the diffusion of ions in and out of pores and the presence of protonated amine-functional groups strongly interacting with Cr(VI) (see theoretical studies below) result in a sorbent with exceptionally rapid sorption kinetics.
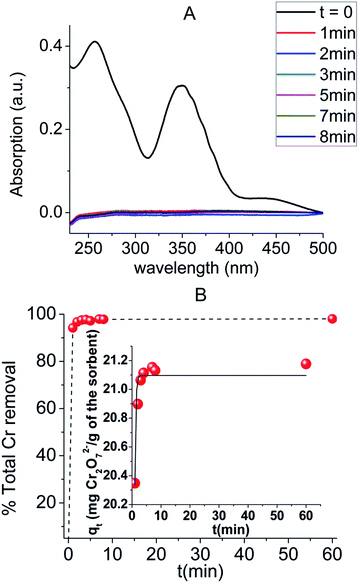 |
| Fig. 6 (A) Selected UV-Vis data from the kinetic experiments (initial dichromate concentration = 21.6 ppm, pH ∼ 3). The Cr2O72− anions are not detectable after the sorption process with UV-Vis and thus, the dichromate concentrations of the solutions were determined by ICP-MS. (B) % Total Cr removal (determined by ICP-MS data) by MOR-1–HAvs. time (min). Inset graph: fitting of the kinetics data with the Lagergren's first-order equation. | |
Variable pH studies.
Although the Cr(VI) ion exchange studies for MOR-1–HA were mainly performed at pH ∼ 3 in order to evaluate the capability of the sorbent to operate under acidic conditions usually present in industrial waste, the composite material is capable of absorbing Cr(VI) from solutions of a relatively wide pH range (1–8), Fig. 7. Specifically, it shows 91–98% total Cr removal capacities in pH ∼ 3–8 (initial dichromate concentration = 21.6 ppm), whereas it retains high Cr removal capability even under highly acidic conditions (∼81 and 90% removal capacities at pH ∼ 1 and 2, respectively).
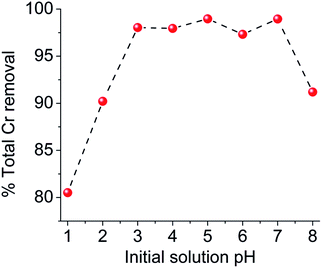 |
| Fig. 7 % Total Cr removal (determined by ICP-MS data) by MOR-1–HAvs. pH (initial dichromate concentration = 21.6 ppm). For pH ≥ 9, dissolution of the composite was observed. | |
Selectivity studies.
Cr(VI) bearing industrial effluent contains a number of competitive anions, such as Cl−, NO3−, Br− and SO42−, in high concentrations. Thus, we have performed competitive Cr2O72−/Cl−, Cr2O72−/Br−, Cr2O72−/NO3− and Cr2O72−/SO42− sorption experiments for MOR-1–HA. We have observed an exceptional ability of MOR-1–HA to absorb Cr2O72− (initial concentration = 54 ppm, pH ∼ 3) almost quantitatively (81.6–97.6% dichromate removal capacity) and very high
(4.4 × 103 to 4 × 104 L g−1) in the presence of a tremendous (up to 1000-fold) excess of Cl−, Br−, or NO3−, which indicates the very high selectivity of MOR-1–HA for Cr2O72− against these anions (Fig. S7 and Table S2†). SO42− as a bivalent anion is expected to be stronger competitor than monovalent anions for dichromate anion exchange. Nevertheless, even with relatively large (20–80-fold) excess of SO42−, MOR-1–HA retained a very good Cr2O72− removal efficiency (40–68%) and relatively high
values (up to 2.1 × 103 mL g−1) (Fig. S7 and Table S2†). By plotting
vs. log(CB) (CB = concentration of Cl−, Br−, NO3− or SO42− ions in mol L−1), large selectivity coefficients15 of MOR-1–HA for Cr2O72− against Cl−, Br−, NO3− and SO42− were observed (selectivity coefficient K(Cr2O72−/Cl−) = 200, K(Cr2O72−/Br−) = 229, K(Cr2O72−/NO3−) = 224, K(Cr2O72−/SO42−) = 159, ESI†).
Comparative batch ion-exchange studies.
For comparison, we have also performed batch Cr2O72− sorption studies (at pH ∼ 3) for (a) protonated MOR-1 [Zr6O4(OH)4(NH3+-BDC)6]Cl6 (MOR-1 treated with HCl 4 M), (b) non-protonated MOR-1 [Zr6O4(OH)4(NH2-BDC)6] (prepared without adding acid in the reaction mixture) and (c) UiO-66 MOF ([Zr6O4(OH)4(BDC)6])–HA composite. The results indicate that the sorption capacities of protonated MOR-1 and non-protonated MOR-1 are similar to each other (247 ± 10 and 267 ± 23 mg g−1 respectively, Fig. S6 and Table S1†) and also close to that of MOR-1–HA composite, whereas the sorption capacity (129 ± 18 mg g−1) of UiO-66–HA is almost half of that for MOR-1–HA. The efficiency, however, of protonated MOR-1 and MOR-1–HA for sorption of dichromate in relatively low initial concentrations, as revealed by the
values, is significantly higher than that of non-protonated MOR-1 and UiO-66–HA. Specifically, UiO-66–HA and non-protonated MOR-1 materials show
values of 2.3 and 6.5 × 103 L g−1, respectively, for initial dichromate concentration of ∼21.6 ppm (Fig. 8), which are one order of magnitude less than those (∼5.5 × 104 L g−1) for MOR-1–HA and protonated MOR-1 samples (Fig. 8).
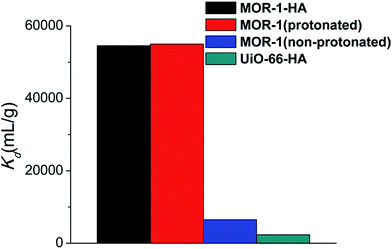 |
| Fig. 8
K
d values for MOR-1–HA, MOR-1 (protonated), MOR-1 (non-protonated) and UiO-66–HA (initial dichromate concentration = 21.6 ppm). | |
As the above results revealed, both protonated and non-protonated MOR-1 materials display similar maximum sorption capacities, since at the acidic environment the NH2-groups will be eventually protonated and the inserted Cl− can be exchanged by dichromate anions. However, at low initial Cr(VI) concentrations the materials pre-treated with acid (i.e. protonated MOR-1 and MOR-1–HA) are much more effective for the sorption of Cr(VI) as revealed by their much higher Kd values compared to that of MOR-1 used without any pre-treatment (non-protonated MOR-1). Presumably, the pre-existence of exchangeable Cl− anions in the protonated materials enhances the kinetics of the Cr(VI) sorption, whereas the Cr(VI) sorption by the non-protonated MOR is a slower two-step process involving first protonation of the amine-sites/insertion of Cl− anions and then exchange of Cl− by Cr(VI) species. The enhancement of sorption kinetics is particularly important in the case of low initial Cr(VI) concentrations, which are not as effective as the high Cr(VI) levels at shifting the ion-exchange equilibrium towards the Cr(VI)-containing material. The above explanation is supported by the kinetic study of the Cr2O72− exchange of the non-protonated MOR-1 (Fig. S8†) using a relatively low initial dichromate concentration (21.6 ppm, pH ∼ 3). The results showed that after 1 min of solution/MOR contact only 24% Cr2O72− removal is achieved, whereas even after 60 min of reaction significant amount of dichromate remains in the solution (∼76% Cr2O72− removal). These data are in contrast with the corresponding kinetic results for MOR-1–HA which indicated almost quantitative sorption of dichromate anions within only 1 min of solution/composite contact (Fig. 6). Furthermore, fitting of the kinetic data for the non-protonated MOR-1 with the Lagergren's first order equation revealed a rate constant of 0.55 ± 0.14 min−1 (Fig. S8†), which is six-times smaller than that for the Cr2O72− sorption by MOR-1–HA. This improvement of kinetics via the protonation of the material is the key for its substantially higher column sorption efficiency compared to that of non-protonated sorbent (see below).
Column ion exchange studies
The next step in our investigations was the study of the column Cr(VI) sorption properties of the MOR-1–HA material. At this point, we should mention that efforts to use as-prepared MOR-1 (even after mixing it with inert materials such as sand) in columns, were unsuccessful since MOR-1 forms fine suspensions in water that pass through the column (Fig. 9A). In contrast, MOR-1–HA composite is kept fixed in the column (Fig. 9B) and thus, it could be successfully employed for column sorption studies. The stationary phase in the columns was a mixture of MOR-1–HA and sand, a common inexpensive and inert material typically used in columns. The use of such mixtures instead of the pure composite has several advantages: (a) the pieces of the composite material are immobilized (not disturbed and moved by the water flow) and separated by particles of sand thus ensuring a continuous water flow through the column, (b) the pressure exerted by water on the composite will be reduced since part of this pressure will be absorbed by the second material (sand) and (c) mixing the composite material with a very low cost material such as sand is economically attractive. We should note that no clogging of MOR-1–HA/sand columns was observed after passing several liters of solutions through them. Remarkably, we found that stationary phases containing only ∼1% wt of MOR-1–HA and 99% wt sand are very effective for the removal of either high or low concentration Cr(VI) from aqueous solutions of various compositions. It can be seen that highly concentrated dichromate solution (C0 = 1075 ppm, pH ∼ 3) is decolorized after passing it through the MOR-1–HA/sand column and also the stationary phase changed color from cream white to orange (red)-brown after the sorption of significant amount of Cr2O72− anions (Fig. 10A and C). The sorbent can be easily regenerated by washing it with HCl solution (1.2–4 M) (Fig. 10B). The regeneration can be visually observed by the restoration of the cream white color of the initial MOR-1–HA/sand stationary phase (Fig. 10D).
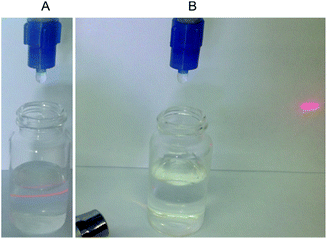 |
| Fig. 9 (A) As-prepared MOR-1/sand column is washed with HCl acid (for protonation or regeneration of the sorbent) and then deionized water (to remove excess acid). As a result, MOR-1 is coming through the column, since it forms a fine suspension as confirmed by the test with the laser beam. (B) The effluent collected after washing the MOR-1–HA/sand column with HCl acid and deionized water is a clear solution (as confirmed by the test with the laser beam). | |
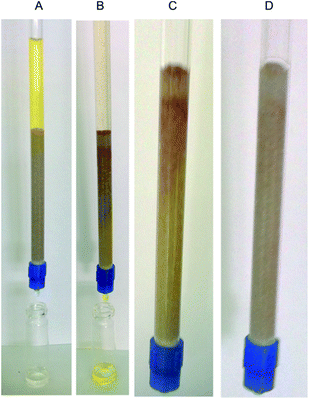 |
| Fig. 10 (A) Decolouration of a Cr2O72− solution (initial concentration = 1075 ppm, pH ∼ 3) with an ion-exchange column of MOR-1–A/sand (0.05 : 5 g). (B) Regeneration of the Cr2O72−-loaded column by washing it with 4 M HCl solution. (C) Column with the stationary phase saturated with Cr2O72−. (D) Column after the regeneration process. | |
Detailed column sorption studies were performed with Cr2O72− solutions of relatively low or trace concentrations, which cannot be treated with common methods such as precipitation. Specifically, column sorption of a solution (pH ∼ 3) of dichromate anions with concentration of 6 ppm results in total Cr concentrations ≤ 47 ppb, which are below than the USA-EPA and EU allowed limits for total Cr in water, for 80 bed volumes (bed volume = [bed height (cm) × cross sectional area (cm2)] mL) of the effluent (Fig. 11A). After regeneration, a breakthrough curve almost identical to that of the first run was obtained, whereas only a small decrease (∼6 bed volumes) of the breakthrough capacity was observed after a fourth run of the column (Fig. 11A).
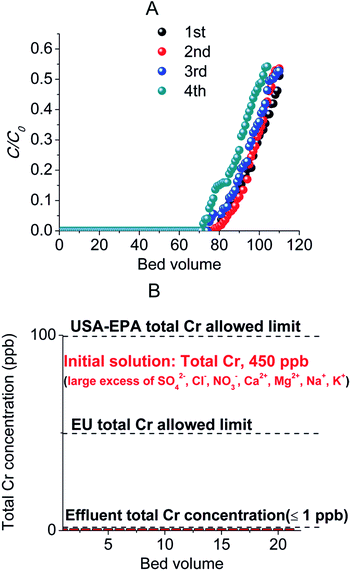 |
| Fig. 11 (A) Breakthrough curves for four column ion exchange runs (C = concentration of the effluent, C0 = initial Cr2O72− concentration = 6 ppm, pH ∼ 3, flow rate 1 mL min−1, one bed volume = 3.5 mL, stationary phase MOR-1–A/sand = 0.05 : 5 g). (B) Total Cr concentration vs. bed volume (each bed volume = 3.5 mL) of natural spring water, to which trace Cr(VI) was added (initial total Cr concentration ∼ 450 ppb, pH adjusted to 3), after passing the solution through an ion-exchange column of MOR-1–A/sand (0.05 : 5 g). | |
Column sorption studies have been also conducted with dichromate solutions (pH ∼ 3, C = 7 ppm) containing 100-fold excess of each of Cl−, Br− and NO3− anions. Still, the MOR-1–HA/sand column shows significant breakthrough capacity (∼43 bed volumes) (Fig. S9†), a value which remains exactly the same after its regeneration.
Because of the excellent Cr2O72−-column sorption properties described above, we decided to examine the applicability of MOR-1–HA/sand column for remediation of real-world water samples intentionally contaminated by trace concentrations of Cr2O72−. Specifically, we tested the performance of this ion exchange column for the decontamination of natural spring water solutions (with the pH of the solution adjusted to ∼3) to which trace levels of Cr2O72− (total Cr concentration analysed with ICP-MS ∼450 ppb) were added. Note that the tested water solutions contain 27, 28 and 305-fold excess of SO42−, NO3− and Cl− anions, respectively, compared to the initial concentration of dichromate anions. The results indicated that at least 21 samples (bed volumes) collected after running the column three times (with regeneration of the column after each run) contain total Cr in a concentration ≤ 1 ppb, i.e. well below the allowed USA-EPA and EU levels for total Cr in water, Fig. 11B. We should also mention that no Zr was detected in the effluent samples (by ICP-MS analysis), thus excluding MOR-1–HA leaching from the column.
Finally, we investigated the performance of MOR-1–CA/sand column for dichromate sorption. The results revealed a breakthrough capacity of only 15 bed volumes (initial dichromate concentration = 6 ppm, pH ∼ 3, MOR-1–CA/sand = 0.05
:
5 g, Fig. S10†) for this column, which is ∼80% smaller than that of the MOR-1–HA/sand column (77 bed volumes, Fig. 11a). These data reflect the significantly enhanced Cr(VI) sorption kinetics for the protonated material (i.e.MOR-1–HA), as discussed above.
Mechanism of Cr(VI)-sorption
To provide an explanation for the remarkable selectivity of the protonated amino functionalized material for dichromate anions, we calculated the interaction energies of Cr2O72−, HOCrO3−, Cl−, Br−, NO3−, HOSO3− and SO42− anions with the [Zr6O4(OH)4(NH3+-BDC)6]Cl6–HA (MOR-1–HA), represented by the simple anilinium Ar-NH3+ cation, employing DFT methods (ESI†). The calculated interaction energies along with selected structural parameters of the respective associations are compiled in Table 1, while the optimized geometries and a brief description of them are given in the ESI (Fig. S11†).
Table 1 Interaction energies, IE (in kcal mol−1) and selected structural parameters (bond lengths R in Å, bond angles ∠ in degrees) for the Ar-NH3+⋯A (A = Cl−, Br−, NO3−, HOSO3−, HOCrO3−, Cr2O72−) associations in aqueous solutions calculated by the wB97XD/Def2-TZVPPD/PCM computational protocol
Anion |
IE |
R(O⋯H–N) |
R(N–H) |
∠O⋯H–N |
Cl− |
11.7 |
1.942 |
1.070 |
176.1 |
Br− |
9.9 |
2.139 |
1.061 |
176.0 |
NO3− |
13.3 |
1.597 |
1.071 |
175.7 |
HOSO3− |
12.0 |
1.660 |
1.055 |
166.6 |
2.478 |
1.020 |
110.3 |
HOCrO3− |
12.6 |
1.672 |
1.052 |
159.4 |
2.142 |
1.024 |
127.9 |
Cr2O72− |
15.5 |
1.637 |
1.055 |
163.1 |
Interestingly, our calculations indicate that SO42− abstracts a NH3+ proton from the Ar-NH3+ cation yielding HOSO3− anions via an exothermic process (exothermicity of −26.7 kcal mol−1). Thus, in Cr2O72−/SO42− competition ion-exchange reactions with MOR-1–HA, the actual competitor for dichromate exchange was HOSO3−. The latter as a monovalent anion is expected to be less competitive than SO42− for Cr(VI) sorption. This can be one of the reasons for the relatively high selectivity of MOR-1–HA for Cr(VI) vs. SO42−, which was experimentally observed.
Among the anions studied, the Cr2O72− anion shows the strongest interactions (15.6 kcal mol−1). However, the estimated values of the interaction energies for the Ar-NH3+⋯A (A = Cl−, Br−, NO3−, HOSO3−, HOCrO3−, Cr2O72−) associations could not fully explain the high selectivity of the material under study towards Cr2O72− anions and the limited selectivity for the rest of the competitive anions in the series. Therefore, this selectivity could be due to much stronger interactions between the Cr2O72− anions and the Ar-NH3+ cation. Experimental IR-data indicate that the amine-deformation band is significantly red-shifted for MOR-1–HA@Cr(VI) (1565 cm−1) compared to that for pristine MOR-1–HA (1590 cm−1) and as prepared MOR-1 (1580 cm−1) samples (Fig. 4C and S5†).17a,b Furthermore, the IR peak at 1620 cm−1 (assigned to ring stretching vibration) in the spectrum of MOR-1–HA@Cr(VI), which is also present in the IR spectrum of non-protonated MOR but it is not shown or is of very weak intensity in the spectrum of MOR-1–HA, is indicative of an NH2- rather than NH3+-containing phenyl ring (Fig. S5†).17c In addition, the solid-state UV-Vis spectrum (Fig. S12†) for MOR-1–HA@Cr(VI) reveals a broad feature (around 500 nm) in the visible region (not shown in the spectrum of as-prepared MOR-1 and MOR-1–HA) which may be due to charge transfer from the electron rich NH2-BDC2− ligand to Cr(VI) species (LMCT). The above support strong NH2–Cr(VI) interactions in MOR-1–HA@Cr(VI).
We thus suggest the transformation of dichromate to CrVIO3 species which in turn forms the tetrahedral [(Ar-NH2)CrO3] complex.18 To test this hypothesis, the equilibrium geometry of the [(Ar-NH2)CrO3] complex in aqueous solution was optimized at the wB97XD/Def2-TZVPPD level of theory, Fig. 12. The formation of such complex may be promoted by the significant acidity of anilinium ion (the pKa of the anilinium ion is lower than 4.6). Anilinium may interact with the bridging O atom (nucleophilic center) of Cr2O72− enforcing the rupture of a O–Cr bridging bond with concomitant coordination of aniline to the CrO3 fragment and formation of HOCrO3− anion. The latter subsequently re-equilibrates to produce Cr2O72−:
| Ar-NH3+ + Cr2O72− → (Ar-NH2)CrO3 + HOCrO3− | (4) |
| 2HOCrO3− → Cr2O72− + H2O | (5) |
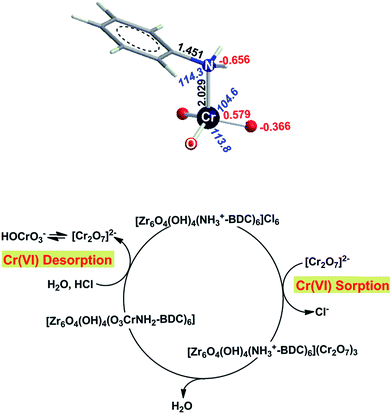 |
| Fig. 12 Top: Equilibrium geometry of the (Ar-NH2)CrO3 complex in aqueous solution optimized at the wB97XD/Def2-TZVPPD level of theory along with selected structural parameters (bond lengths in Å and bond angles marked in blue in degrees) and the natural atomic charges (marked in red) on the donor atoms constituting the coordination sphere and the central Cr atom. Bottom: Suggested mechanism for the Cr(VI) sorption and desorption. | |
The condensation of the HOCrO3− anion to form Cr2O72− is particularly an enthalpy driven reaction with a dimerization constant K = 159 at standard conditions.19
The formation of the (Ar-NH2)CrO3 complex is an almost thermoneutral process, the endothermicity found to be 1.8 kcal mol−1. The presence of six NH3+ functional groups per Zr6 cluster affords six moles of the (Ar-NH2)CrO3 complex, thus accounting well for the experimentally observed sorption of ∼3 moles of Cr2O72− per formula unit of the MOR (Fig. 12).
Note that oxochromium(VI)-amine complexes are well-known compounds and many of them have been used as oxidants in organic synthesis.20 The brick-red colour of the oxochromium(VI)-amine complexes20 could account well for the change of colour from cream white to orange (red)-brown of the MOR-1–HA sorbent observed experimentally.
The regeneration of the MOR-1–HA columns by treating them with concentrated HCl solutions (1.2–4 M) can be easily explained by the acidic hydrolysis of the (Ar-NH2)CrO3 complex
| [(Ar-NH2)CrO3] + H2O → Ar-NH3+ + HOCrO3− | (6) |
with concomitant dimerization of HOCrO
3− to Cr
2O
72− (
Fig. 12). The exothermicity of the hydrolysis is predicted to be 30.5 kcal mol
−1 at the wB97XD/Def2-TZVPPD level. The estimated binding energy of the aniline ligand with the CrO
3 moiety is 34.4 kcal mol
−1, while the negative natural atomic charge on the coordinated N donor atom renders the N atom susceptible to electrophilic attack by the H
+ ions, which is transformed to ammonium NH
3+ salt, thus regenerating the
MOR-1–HA column.
Conclusions
Clearly, metal–organic resins (MORs) can be the next generation of resins, since their sorption capability is not only based on the functional-binding group (as occurs with the conventional organic resins) but also on their highly ordered porous structure enhancing the diffusion of ions and the sorption kinetics. Such materials, however, due to their very small particle size form fine suspensions in water, thus being not suitable for water treatment applications. We were able to overcome this limitation of MORs by engineering MOR–alginic acid (HA) composite form, in which the MOR particles are covered by a water-insoluble HA shell. Thus, the successful utilization of MOR–HA composite as stationary phase in ion-exchange columns was achieved. The excellent performance of such ion exchange columns, as demonstrated by their high efficiency for removal of Cr(VI) under a variety of conditions, points towards real-world environmental remediation applications of MOR-based sorbents.
Acknowledgements
The powder X-ray diffraction unit of the Network of Research Supporting Laboratories at the University of Ioannina is acknowledged. The work performed at Northwestern University was supported by National Science Foundation grant DMR-1410169.
References
- E. A. Katayev, Y. A. Ustynyuk and J. L. Sessler, Coord. Chem. Rev., 2006, 250, 3004 CrossRef CAS.
-
(a) M. Costa and C. B. Klein, Crit. Rev. Toxicol., 2006, 36, 155 CrossRef CAS PubMed;
(b) F. Brito, J. Ascanio, S. Mateo, C. Hernandez, L. Araujo, P. Gili, P. MartinZarza, S. Dominguez and A. Mederos, Polyhedron, 1997, 16, 3846 CrossRef.
-
(a) USA-EPA. Chromium in drinking water, http://water.epa.gov/drink/info/chromium/;
(b) European Commission. Council Directive 98/83/EC on the quality of water intended for human consumption, http://eur-lex.europa.eu/legal-content/EN/TXT/?uri=CELEX:31998L0083;
(c) C. S. Peng, H. Meng, S. X. Song, S. Lu and A. Lopez-Valdivieso, Sep. Sci. Technol., 2004, 39, 1501 CrossRef CAS.
-
(a) R. W. Liang, F. F. Jing, L. J. Shen, N. Qin and L. Wu, J. Hazard. Mater., 2015, 287, 364 CrossRef CAS PubMed;
(b) L. B. Hoch, E. J. Mack, B. W. Hydutsky, J. M. Hershman, I. M. Skluzacek and T. E. Mallouk, Environ. Sci. Technol., 2008, 42, 2600 CrossRef CAS PubMed.
-
A. A. Zagorodni, Ion Exchange Materials, Properties and Applications, Elsevier, 2007 Search PubMed.
-
M. J. McGuire, N. Blute, G. Qin, P. Kavounas, D. Froelich, and F. Leighton, Hexavalent Chromium Removal Using Anion Exchange and Reduction with Coagulation and Filtration, AWWA Research Foundation, 2007 Search PubMed.
- S. Kaneko and M. Ogawa, Appl. Clay Sci., 2013, 75–76, 109 CrossRef CAS.
-
(a) M. Eddaoudi, D. B. Moler, H. L. Li, B. L. Chen, T. M. Reineke, M. O'Keeffe and O. M. Yaghi, Acc. Chem. Res., 2001, 34, 319 CrossRef CAS PubMed;
(b) G. Ferey, Chem. Soc. Rev., 2008, 37, 191 RSC;
(c) S. Horike, S. Shimomura and S. Kitagawa, Nat. Chem., 2009, 1, 695 CrossRef CAS PubMed;
(d) D. Bradshaw, J. B. Claridge, E. J. Cussen, T. J. Prior and M. J. Rosseinsky, Acc. Chem. Res., 2005, 38, 273 CrossRef CAS PubMed;
(e) O. K. Farha and J. T. Hupp, Acc. Chem. Res., 2010, 43, 1166 CrossRef CAS PubMed;
(f) S. T. Zheng, T. Wu, J. A. Zhang, M. Chow, R. A. Nieto, P. Y. Feng and X. H. Bu, Angew. Chem., Int. Ed., 2010, 49, 5362 CrossRef CAS PubMed.
-
(a) H. H. Fei, M. R. Bresler and S. R. J. Oliver, J. Am. Chem. Soc., 2011, 133, 11110 CrossRef CAS PubMed;
(b) H. H. Fei, C. S. Han, J. C. Robins and S. R. J. Oliver, Chem. Mater., 2013, 25, 647 CrossRef CAS;
(c) H. R. Fu, Z. X. Xu and J. Zhang, Chem. Mater., 2015, 27, 205 CrossRef CAS;
(d) X. X. Li, H. Y. Xu, F. Z. Kong and R. H. Wang, Angew. Chem., Int. Ed., 2013, 52, 13769 CrossRef CAS PubMed;
(e) P. F. Shi, B. Zhao, G. Xiong, Y. L. Hou and P. Cheng, Chem. Commun., 2012, 48, 8231 RSC;
(f) Q. Zhang, J. Yu, J. Cai, L. Zhang, Y. Cui, Y. Yang, B. Chen and G. Qian, Chem. Commun., 2015, 51, 14732 RSC.
-
G. Tchobanoglous, F. L. Burton and H. D. Stensel, Wastewater engineering: treatment, disposal, and reuse, McGraw-Hill, 1991 Search PubMed.
-
(a) J. H. Cavka, S. Jakobsen, U. Olsbye, N. Guillou, C. Lamberti, S. Bordiga and K. P. Lillerud, J. Am. Chem. Soc., 2008, 130, 13850 CrossRef PubMed;
(b) M. J. Katz, Z. J. Brown, Y. J. Colon, P. W. Siu, K. A. Scheidt, R. Q. Snurr, J. T. Hupp and O. K. Farha, Chem. Commun., 2013, 49, 9449 RSC;
(c) M. Kandiah, M. H. Nilsen, S. Usseglio, S. Jakobsen, U. Olsbye, M. Tilset, C. Larabi, E. A. Quadrelli, F. Bonino and K. P. Lillerud, Chem. Mater., 2010, 22, 6632 CrossRef CAS;
(d) A. J. Howarth, M. J. Katz, T. C. Wang, A. E. Platero-Prats, K. W. Chapman, J. T. Hupp and O. K. Farha, J. Am. Chem. Soc., 2015, 137, 7488 CrossRef CAS PubMed;
(e) K. K. Yee, N. Reimer, J. Liu, S. Y. Cheng, S. M. Yiu, J. Weber, N. Stock and Z. T. Xu, J. Am. Chem. Soc., 2013, 135, 7795 CrossRef CAS PubMed;
(f) C. B. He, K. D. Lu and W. B. Lin, J. Am. Chem. Soc., 2014, 136, 12253 CrossRef CAS PubMed;
(g) L. Zhu, D. Zhang, M. Xue, H. Li and S. Qiu, CrystEngComm, 2013, 15, 9356 RSC;
(h) W. Morris, W. E. Briley, E. Auyeung, M. D. Cabezas and C. A. Mirkin, J. Am. Chem. Soc., 2014, 136, 7261 CrossRef CAS PubMed.
-
(a) A. I. Spjelkavik, Aarti, S. Divekar, T. Didriksen and R. Blom, Chem.–Eur. J., 2014, 20, 8973 CAS;
(b) Y. H. Li, F. Q. Liu, B. Xia, Q. J. Du, P. Zhang, D. C. Wang, Z. H. Wang and Y. Z. Xia, J. Hazard. Mater., 2010, 177, 876 CrossRef CAS PubMed;
(c)
M. G. Kanatzidis, D. Sarma and M. J. Manos, Column material for the capture of heavy metal and precious metal ions, US Pat., 20150144568, 2015.
-
(a)
M. Bosnic, J. Buljan, R. P. Daniels and S. Rajamani, Pollutants in tannery effluent, published online, 2003, http://leatherpanel.org/sites/default/files/publications-attachments/polutants.pdf Search PubMed;
(b) F. Akbal and S. Camci, Desalination, 2011, 269, 214 CrossRef CAS.
-
(a) M. C. Biesinger, C. Brown, J. R. Mycroft, R. D. Davidson and N. S. McIntyre, Surf. Interface Anal., 2004, 36, 1550 CrossRef CAS;
(b) E. Desimoni, C. Malitesta, P. G. Zambonin and J. C. Riviere, Surf. Interface Anal., 1988, 13, 173 CrossRef CAS.
-
(a) M. J. Manos, N. Ding and M. G. Kanatzidis, Proc. Natl. Acad. Sci. U. S. A., 2008, 105, 3696 CrossRef CAS PubMed;
(b) M. J. Manos and M. G. Kanatzidis, J. Am. Chem. Soc., 2009, 131, 6599 CrossRef CAS PubMed;
(c) M. J. Manos and M. G. Kanatzidis, Chem.–Eur. J., 2009, 15, 4779 CrossRef CAS PubMed;
(d) M. J. Manos and M. G. Kanatzidis, J. Am. Chem. Soc., 2012, 134, 16441 CrossRef CAS PubMed;
(e) M. J. Manos, V. G. Petkov and M. G. Kanatzidis, Adv. Funct. Mater., 2009, 19, 1087 CrossRef CAS;
(f) J. L. Mertz, Z. H. Fard, C. D. Malliakas, M. J. Manos and M. G. Kanatzidis, Chem. Mater., 2013, 25, 2116 CrossRef CAS.
- A. Benhammou, A. Yaacoubi, L. Nibou and B. Tanouti, J. Colloid Interface Sci., 2005, 282, 320 CrossRef CAS PubMed.
-
(a) M. Karabacak, M. Cinar, Z. Unal and M. Kurt, J. Mol. Struct., 2010, 982, 22 CrossRef CAS;
(b)
D. Lin-Vien, N. Colthup, W. Fateley and J. Grasseli, The Handbook of Infrared and Raman Characteristic Frequencies of Organic Molecules, Academic Press, 1991 Search PubMed;
(c) M. Tanaka and S. Ogasawar, J. Catal., 1972, 25, 111 CrossRef CAS.
- Two crystal structures of tetrahedral [L(CrVIO3] complexes are reported in the following references:
(a) M. R. Sundberg, R. A. M. Uggla, R. Sillanpaa, K. K. Zborowski, A. Sanchez-Gonzalez, J. K. T. Matikainen, S. A. A. Kaltia and T. A. Hase, Cent. Eur. J. Chem., 2010, 8, 486 CrossRef CAS;
(b) W. Mazurek, G. D. Fallon, P. J. Nichols and B. O. West, Polyhedron, 1990, 9, 777 CrossRef CAS.
- F. Brito, J. Ascanio, S. Mateo, C. Hernandez, L. Araujo, P. Gili, P. MartinZarza, S. Dominguez and A. Mederos, Polyhedron, 1997, 16, 3835 CrossRef CAS.
-
(a) H. H. Sisler, J. D. Bush and O. E. Acoountius, J. Am. Chem. Soc., 1948, 70, 3827 CrossRef CAS PubMed;
(b) G. I. Poos, G. E. Arth, R. E. Beyler and L. H. Sarett, J. Am. Chem. Soc., 1953, 75, 422 CrossRef CAS;
(c)
G. Tojo and M. Fernandez, Oxidation of Alcohols to Aldehydes and Ketones, Springer, Berlin, 2006, pp. 1–97 Search PubMed.
Footnotes |
† Electronic supplementary information (ESI) available: Synthesis procedures, experimental details of physical measurements, SEM images, thermal analysis, CO2 sorption data and pore size distribution, adsorption isotherms and details for the estimation of selectivity of MOR-1–HA for dichromate, IR spectrum, breakthrough curve in presence of excess of competitive ions and for MOR-1–CA, additional kinetic and characterization data, computational details and optimized geometries for Ar-NH3+⋯A complexes. See DOI: 10.1039/c5sc03732h |
‡ These authors contributed equally. |
|
This journal is © The Royal Society of Chemistry 2016 |