DOI:
10.1039/C6RA24692C
(Paper)
RSC Adv., 2017,
7, 5610-5620
Uncovering the metastable γ-Ag2WO4 phase: a joint experimental and theoretical study†
Received
3rd October 2016
, Accepted 8th November 2016
First published on 9th November 2016
Abstract
The synthesis of metastable solid phases is a pivotal starting point for innovative materials research. Here, we report the synthesis by means of a precipitation method of the metastable cubic γ-Ag2WO4 phase under ambient conditions. Different experimental techniques such as X-ray diffraction (XRD) with Rietveld refinement, field emission scanning electron microscopy (FE-SEM), micro-Raman/ultraviolet-visible (UV-vis) diffuse reflectance, photoluminescence spectroscopies, and differential scanning calorimetry (DSC) were employed. To complement the experimental data, the geometry, morphology, vibrational and electronic structure of γ-Ag2WO4 were characterized and evaluated using first-principles quantum mechanical calculations at the density functional theory (DFT) level. A theoretical model based on the Wulff construction was introduced to explain the possible crystal morphologies of the faceted crystals, which were obtained by tuning the surface chemistry of the crystals, and are related to their relative stability. Both the experimental and theoretical data revealed the presence of (100), (110), and (111) facets with low surface energies in the γ-Ag2WO4 crystals. When the surface energy value of the (111) surface was lowered, the experimental morphologies of the as-synthesized samples were similar to the theoretically obtained shapes. From the analysis of the experimental and calculated Raman spectra, PL emissions, and the density of states, we can assume that γ-Ag2WO4 is primarily formed by both distorted clusters: [WO4] tetrahedra and, distorted in a lesser extent, [AgO6] octahedra.
1. Introduction
Polymorphism is the ability of a given material to exist in more than one form, that is, to have different crystal structures and properties, while maintaining the same chemical composition. Polymorphism is a key property that enables researchers to investigate different structures depending on external conditions, such as temperature, pressure, type of field (electric, magnetic, etc.), synthetic procedure, and mode and duration of the material processing, i.e., the enhancement of a particular phase modification, out of all possible polymorphic phases, depends on the thermodynamic and kinetic factors. Research on the synthesis of metastable material phases has been fueled by studies where it has been found that these phases exhibit unexplored physical and chemical properties that are distinct from the properties of their thermodynamically stable counterparts.1–5 However, these studies have been incomplete due to the propensity of the metastable phases to transform into more stable phases. In addition, the challenge to develop facile methods for the synthesis of metastable materials remains.6,7 Thus, it would be highly desirable to investigate metastable material phases in a manner similar to that adopted for the thermodynamically stable ones, so as to put both on a comparable footing.
Silver tungstate (Ag2WO4)-based materials constitute a particularly intensively researched family of inorganic compounds because of their particular crystal and electronic structures.8–12 In response to their extensive range of innovative properties and practical applications, a plethora of experimental works have been published on the synthesis and properties of Ag2WO4-based materials,13–26 and on their applications in photocatalysis,17,19,20,25 catalysis,14,18 photoluminescence,9,13,15 sensors,22 and as antibacterial agents.21,23,26 Ag2WO4 exhibits stunningly complex polymorphism,27 and its understanding has evoked recent interest due to both fundamental scientific and technological aspects. Apart from the orthorhombic and thermodynamically stable α-Ag2WO4 phase, β- and γ-phases have also been identified with hexagonal and cubic structures, respectively.27 In addition, it has been well established that these β- and γ-phases are metastable phases that can be transformed into the α-phase when heated.
To date, different synthesis methods have been reported in the literature to obtain Ag2WO4 metastable phases.27–33 In particular, β-Ag2WO4 was successfully prepared by a precipitation method using a surfactant-assisted route in the presence of polymethacrylic acid (PMAA),28 acrylamide (AM),26 or polyvinylpyrrolidone (PVP).29 The transformation from the metastable β-phase to the thermodynamically stable α-phase was inhibited by the surfactant. Without the addition of any surfactants, other conditions (such as temperature and pH) can serve as inhibitors to prevent this phase transformation. For example, a metastable β-Ag2WO4 phase, which coexisted with a weak amount of α-phase, was obtained using a co-precipitation method at pH 4;31 in addition, the synthesis of pure β-Ag2WO4 at low temperatures has also been reported.30 Recently, our research group reported the synthesis of β-Ag2WO4 microcrystals by simple34 and drop-wise precipitation35 methods. Moreover, Gupta et al. presented a new strategy for synthesizing hexagonal metastable β-Ag2WO4 at room temperature through the use of aliovalent Eu3+ dopants (to induced the orthorhombic (α-Ag2WO4) to hexagonal (β-Ag2WO4) phase transition) along with the co-precipitation method.36 Less is known about the γ-Ag2WO4 phase due to its scarcity and complex synthesis, and practically no attempt has been reported regarding the formation of a pure, metastable γ-Ag2WO4.27,32,33 Thus, the transfer of all the preceding experiments to the corresponding cubic phase is most rewarding with respect to elucidating the common properties and differences between the α- and β-Ag2WO4 modifications.
The simultaneous use of theoretical calculations alongside experiments has been a significant aid for the interpretation and assignment of complex spectra. This combination has previously been applied to the investigation of complex materials such as β-Ag2WO4,35 Ag2CrO4,37 and Ag4V2O7 (ref. 38) crystals. To the best of our knowledge, combination of theoretical and experimental studies on the structure of γ-Ag2WO4 crystals is inexistent. This encouraged us to investigate the geometry, cluster coordination, and electronic structure of γ-Ag2WO4 crystals.
Herein, for the first time, the facile synthesis and characterization of the metastable cubic γ-Ag2WO4 phase is reported. Different experimental techniques such as X-ray diffraction (XRD) with Rietveld refinement, field emission scanning electron microscopy (FE-SEM), micro-Raman/ultraviolet-visible (UV-vis) diffuse reflectance, photoluminescence (PL) spectroscopies, and differential scanning calorimetry have been employed. In addition, the geometry, electronic structure, morphology, and optical properties of the γ-Ag2WO4 phase were investigated with first-principles quantum mechanical calculations at the density functional theory (DFT) level, and the theoretical results were compared with the experimental data. The calculations helped us to interpret and understand the physical mechanisms that drive the experimental results. The remainder of this paper is organized as follows: Section 2 describes the experimental procedure and computational details; Section 3 contains the results and discussion; and Section 4 provides a summary of this study and the concluding remarks.
2. Experimental
2.1 Material synthesis
High-purity silver nitrate (AgNO3; 99.8% purity, Sigma-Aldrich) and sodium tungstate (Na2WO4·2H2O; 99.0% purity, Sigma-Aldrich) were obtained from commercial sources and used as received. The γ-Ag2WO4 phase was obtained by simultaneous addition of 1.13 g (6.66 mmol) of AgNO3 and 1.10 g (3.33 mmol) of Na2WO4·2H2O in 20 mL of deionized water. The reaction was performed under low temperature conditions (0–5 °C) and under continuous stirring. In order to have a better understanding of the process of formation of the γ-Ag2WO4 particles, the reaction progress was checked in two moments: one portion was extracted of the reaction after 10 min and then the precipitate was collected; and the second portion was allowed to react for another 10 min, for a total 20 min reaction time. Subsequently, the two portions were washed several times with acetone and dried at 50 °C.
2.2 Characterization
In order to determine the crystal phase, X-ray diffraction (XRD) measurements were carried out using a D/max-2000/PC X-ray powder diffractometer (Rigaku) with Cu Kα radiation at a scanning speed of 2° min−1. The XRD accelerating voltage and emission current were 40 kV and 30 mA, respectively. The microstructure and surface characteristics were investigated using an FE-SEM Inspect F50 (FEI Company, Hillsboro, OR) apparatus operated at 10 kV. High-resolution Raman spectra were recorded with a He–Ne laser at 632.81 nm (model CCD DU420AOE325) operating at 25–1000 cm−1, keeping its maximum output power at 6 mW. The transformation of the material during its heat treatment was studied by differential scanning calorimetry (DSC) using an STA 409/429 Netzsch instrument at a rate of 10 °C min−1 from 25 to 700 °C. UV-vis diffuse reflectance spectra measurements were carried out using a Varian spectrophotometer (model Cary 5G) at the 100–800 nm wavelength analysis range. Photoluminescence emission spectra were recorded on a Monospec 27 monochromator (Thermal Jarrel Ash) coupled to an R446 (Hamamatsu Photonics) photomultiplier, and a krypton-ion laser (Coherent Innova 90K; λ = 350 nm), over a wavelength range of 350–850 nm.
2.3 Computational details
Calculations on the periodic γ-Ag2WO4 structure were performed with the CRYSTAL14 software package.39 For the calculations, tungsten was described by a large-core ECP, derived by Hay and Wadt, and modified by Cora et al.,40 while the silver and oxygen centers were described using HAYWSC-311d31G and O (6-31d1G) basis sets, respectively, which were taken from the Crystal website.41 The screened-Coulomb HSE06 (ref. 42 and 43) range-separated hybrid functional was used to obtain accurate band gaps of the computed structures. The diagonalization of the Fock matrix was performed at adequate k-point grids in the reciprocal space. The thresholds controlling the accuracy of the Coulomb and exchange integrals calculations were set to 10−8 and 10−14, and the mixing percent of the Fock/Kohn–Sham matrices was set to 40% (IPMIX keyword).39 The band structure and the density of states (DOS) projected on the atoms and orbitals of bulk γ-Ag2WO4 were constructed along the appropriate high-symmetry directions of the corresponding irreducible Brillouin zone. To confirm the character of local minima on the potential energy of the surfaces, vibrational frequency calculations were carried out to ensure that only positive frequencies corresponded to minima for the studied ordered and disordered structural models.
The disordered models were generated by creating Ag or W atom dislocations from their initial coordinates in the ordered and most stable structure. The following displacements were explored: (i) 0.05 to 0.20 Å in the z-coordinate of the Ag atom; (ii) 0.05 to 0.20 Å in the z-coordinate of the W atom; and (iii) 0.05 to 0.20 Å in the z-coordinate of the Ag and W atoms. These displacements were considered for 25% and 50% of all Ag or W atoms.
Stoichiometric slabs for the (100), (110) and (111) surfaces of the cubic γ-Ag2WO4 structure were modeled, and the surface energy value, Esurf, which is defined as the energy per unit area required to form the surface relative to the bulk, was calculated as follows:
|
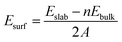 | (1) |
where
Eslab is the total energy of the 2D slab,
nEbulk is the energy of the corresponding amount of bulk Ag
2WO
4 units, and
A represents the surface area, which is created on each side of the 2D slab and is repeated periodically. After the corresponding thickness optimization process and convergence tests, slab models consisting of four molecular units were obtained for the (100) and (111) surfaces, while six molecular units were used for the (110) surface. In addition, different slab terminations have been taken into account in order to find the
Esurf values.
The equilibrium shape of a crystal can be calculated using the classic Wulff construction, which minimizes the total surface free energy at a fixed volume, and provides a simple relationship between the Esurf of the (hkl) plane and its distance in the normal direction from the center of the crystallite. This strategy can be used to correlate the theoretical and experimental morphologies for α and β phases of Ag2WO4 system.44,45
3. Results and discussion
3.1 XRD
The X-ray diffraction pattern of the Ag2WO4 sample is shown in Fig. 1. From the analysis of the peak positions and their relative intensities, we confirmed that the as-synthetized samples, with a lattice parameter of a = 9.335 Å, were consistent with the standard powder diffraction pattern of γ-Ag2WO4 (JCPDS file no. 861157). This lattice parameter value is in agreement with those of other isomorphous spinel compounds such as Na2WO4 with a = 9.13 Å,32 Ag2MoO4 with a = 9.26 Å (ref. 46) and a = 9.25 Å,47 Na2MoO4 with a = 9.108 Å,48 and Ag2WO4 with a = 9.35 Å.27 To confirm the γ-Ag2WO4 structure, the dhkl and the respective relative intensity for the associate peak were compared with other reported data from similar structures as shown in Table S1 of the (ESI†).
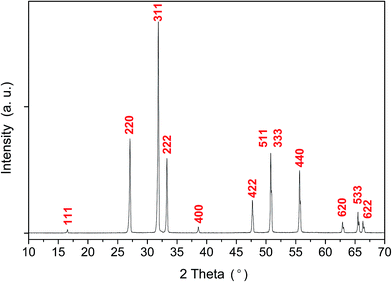 |
| Fig. 1 XRD pattern of γ-Ag2WO4 with its respective Miller index. | |
The theoretical calculations of γ-Ag2WO4 yielded a lattice parameter of 9.3045 Å, which is in agreement with the experimental value obtained, and a simple fully cubic symmetry. The conventional cell for this spinel structure contains 8 formula units where the W atoms occupy the tetrahedral 8a sites, the Ag atoms reside at the octahedral 16d position, and the O atoms stay at the 32e positions in the Fd
m space group, as depicted in Fig. 2. As reported by our group for α- and β-phases, the [AgOy] and [WOz] clusters are the common building blocks in the polyhedral representation of each unit cell.9,15,34,45 However, difference between y and z subscripts are revealed for each structure.
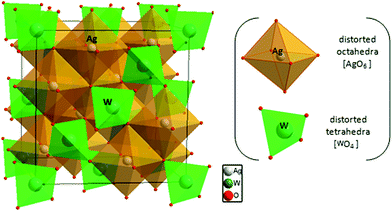 |
| Fig. 2 A polyhedral representation of the γ-Ag2WO4 unit cell. Depicted are the local coordination of the Ag and W atoms corresponding to the octahedral [AgO6] and tetrahedral [WO4] clusters, respectively. | |
3.2 FE-SEM
Different magnification FE-SEM micrographs of samples with synthesis times of 10 and 20 min are shown in Fig. 3, where the morphological evolution of the γ-Ag2WO4 particles can be seen. The images display a broad grain size distribution, at around 2–4 μm, and nearly spherical shapes. For the reaction time of 10 min, some small nanocrystals with a cuboid structure are visible on the large microcrystal surfaces that have not diffused into the interior. The samples corresponding to 20 min reaction time had the most microparticles with irregular or approximately spherical structures, although some faceted particles can be observed. Furthermore, a decrease in the number of nanoparticles on the surface can be perceived. These nanoparticles are observed to be rod-shaped. Although our group had previously reported this rod-like morphology in the α-Ag2WO4 phase,9,12,15 traces of this phase were not detected for the experimental techniques used in this work. For both reaction times, the presence of nanoparticles on the surface of the microparticles can be explained by the incomplete diffusion of these small particles into the larger γ-Ag2WO4 particles. This behavior suggests that an Ostwald-ripening mechanism takes place, where, when the growth kinetics are fast, bigger crystals grow at the expense of smaller nanocrystals.
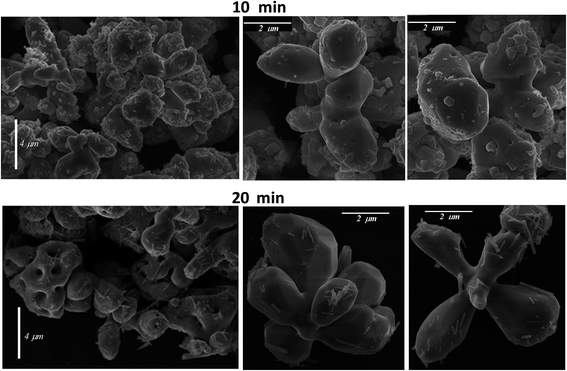 |
| Fig. 3 FE-SEM micrographs at different magnifications for the different times of synthesis. | |
To shed light on the morphology of the γ-Ag2WO4 phase, a theoretical analysis was performed. According to the theoretical calculations, the order of stability of the γ-Ag2WO4 surfaces is (110) < (100) < (111). Slab models with thickness of 9.1 Å, 9.6 Å and 9.3 Å were constructed for the (100), (110) and (111) surfaces, respectively. These surfaces are depicted in Fig. 4, where the most exposed Ag atoms in all surfaces are three-coordinated. The most exposed W atoms form [WO4] clusters as in the bulk. Other non-symmetric slab models constructed for the (100), (110) and (111) surfaces are presented in Fig. S1 of ESI,† which present lower stability.
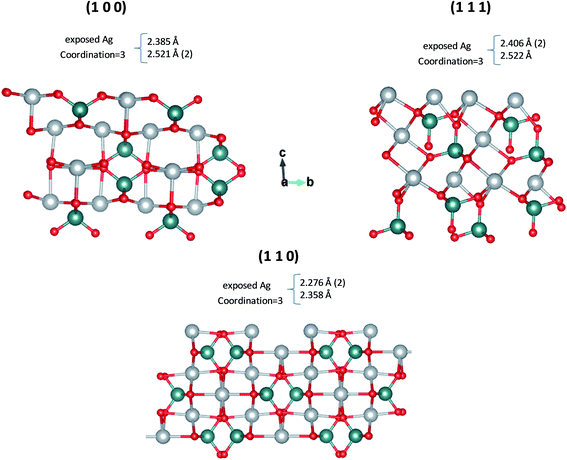 |
| Fig. 4 Slab models constructed for (a) (100), (b) (110) and (c) (111) surfaces. | |
From the Wulff construction, it is derived that the surfaces with the lowest surface energies control the crystal morphology.49 The ideal morphology of γ-Ag2WO4 is a cube dominated by the surface (100). However, it is possible to modify this ideal morphology by tuning the surface energies of the different facets using the Wulff construction.49–51 An analysis of the theoretical results showed that when the relative stability of the facets changes (increases or decreases), more than one type of facet will appear in the resulting morphology. We followed a model recently developed by our research group,44,45 based on the Wulff construction, which can be used to explicitly predict the evolution of morphologies in different environments. The map of the crystal morphologies is displayed in Fig. 5a. The analysis of the theoretical results indicates that the most stable surface is the (110) followed by the (100), which form the ideal morphology (shown in the upper part of Fig. 5a). When the relative stability of the (110) and (100) facets decreased, more than one type of facet appeared in the resulting morphology. A rhombic dodecahedron (picture 1.1 of Fig. 5a) was obtained from the ideal morphology passing through picture 1, as the surface energy of the (110) facet decreased to 0.88 J m−2; while a cube was produced when the surface energy of the (100) facet decreased to 0.82 J m−2 (see morphology 2.1). When the surface energy of the (111) facet decreased to 1.30 J m−2, morphology 3 was obtained, and a subsequent decrease in the surface energy value for the (111) facet rendered the 3.1, 3.2 and 3.3 morphologies (see Fig. 5a). Finally, an octahedron was achieved when the surface energy of the (111) facet decreased to 0.72 J m−2 (morphology 4). The experimental FE-SEM image displayed in Fig. 5b was the best well-faceted sighting to use for comparison. A good agreement between the experimental and theoretical morphologies was obtained when the surface energy values of the (111) facet was 1.20 or 1.15 J m−2. These surface energy values generated 3.1 or 3.2 morphologies, respectively. In the neck region: the particle diverted from the suggested theoretical morphology due to the neck formed with other particles. Thus, variations in the ratio between surface energy values affect the related morphologies, and can be used to obtain correlations with the experimental results. We have reported well-faceted rod-like elongated particles as typical morphology for α- and β-Ag2WO4 microcrystals. For these two polymorphs, a narrow range of values, between 0.2–1.4 J m−2 and 0.08–1.16 J m−2, for α-52 and β-,45 respectively, were calculated for the surface energy values. In comparison, the present work displays: (i) bigger values, 1.17–1.96 J m−2; and (ii) not-faceted shape for γ-Ag2WO4. A possible relationship between value and range of surface energy with the metastability is under review for future works.
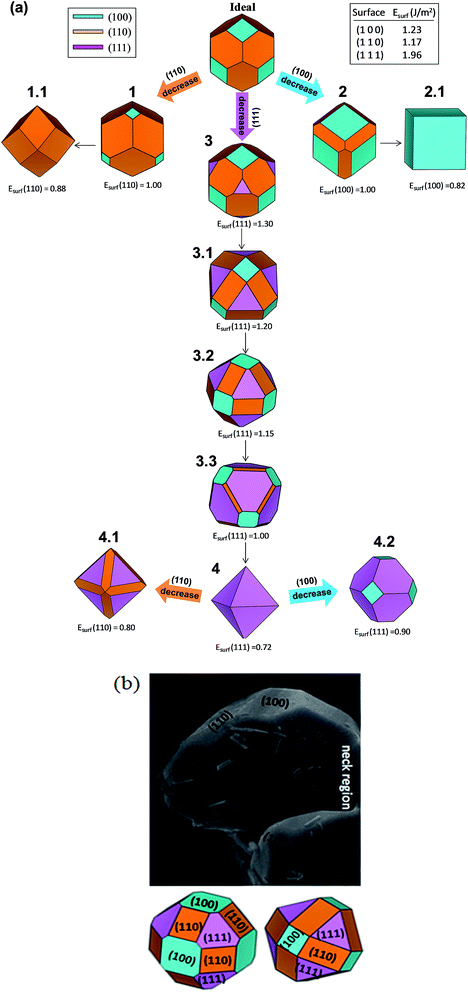 |
| Fig. 5 (a) Theoretical morphology map for γ-Ag2WO4 crystals (the surface energy units are given in J m−2) and (b) experimental FE-SEM images of faceted γ-Ag2WO4 microcrystals. The computationally simulated crystal shapes are included for comparison purposes. | |
3.3 Differential scanning calorimetry
The γ-Ag2WO4 thermograms are shown in Fig. 6. The DSC curve clearly shows three processes. At lower temperatures, the exothermic peak at 184 °C is linked to the γ-α phase transition. Two endothermic peaks at higher temperatures can be observed, abroad peak at 500 °C and a sharp peak at 603 °C, which can be associated to the probable decomposition of the Ag2WO4 and a melting process, respectively. Only the last process is reversible.
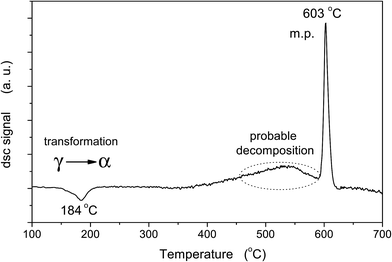 |
| Fig. 6 DSC curves of γ-Ag2WO4. | |
3.4 Raman spectra
Raman spectroscopy is one of the most suitable methods for investigating and characterizing the structure and symmetry of solids, particularly the degree of short-range structural order, and it also provides the degree of structural order–disorder in bonds between atoms in the materials.53 Fig. 7a shows the Raman spectra of γ-Ag2WO4 crystals, where 10 active modes are observed. An analysis of the results rendered the presence of an intense absorption band at 912 cm−1, which did not appear in the other Ag2WO4 phases, as well as active modes at around 880 and 853 cm−1. These bands, as well as others with lower intensities at 658 and 530 cm−1, can be assigned to the W–O stretching bonds, and are usually present in any tungstate sample. Torsional motions of the [WO4] clusters appeared at 361 and 342 cm−1.
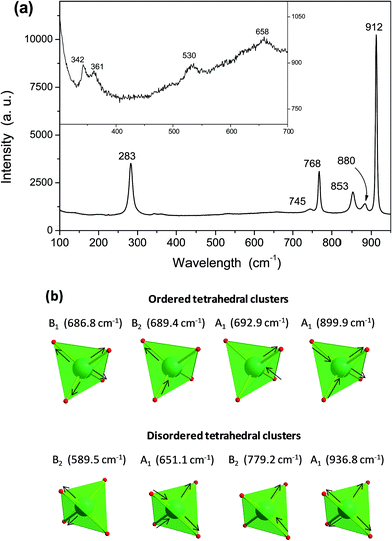 |
| Fig. 7 (a) The micro-Raman spectra of γ-Ag2WO4, the inset shows the wavelength range at a lower intensity band. (b) Theoretical stretching modes in the 580–940 cm−1 range related to the structural rearrangement provoked by the displacement of 50% of the W atoms. | |
In order to better understand the structural arrangement and vibrational behavior of the γ-Ag2WO4 crystals, computational calculations were carried out. The theoretical active Raman modes (Table S2 of the ESI†) can be organized into two groups. For the ordered structure, the first group goes to 380 cm−1 and is composed of the internal vibrations of the symmetric and asymmetric bending of the tetrahedral [WO4] clusters. In the second group, at higher frequencies, there are only two modes, which correspond to the asymmetric and symmetric stretching of the [WO4] clusters at ∼672 cm−1 and ∼905 cm−1, respectively.
Another interesting aspect observed in the Raman spectra is the significant increase of the bandwidth modes, which suggests that some disorder was present due to the weakening of some interatomic interactions. Disorder is used here in its most general sense, namely, any perturbations that disrupt the ideal or ordered crystal. At the time, the emphasis was on the description of the disordered crystal and these disorders were considered to be perturbations. The experimentally observed absorption peaks in the 750–850 cm−1 range, depending on the structural distortion related to a displacement of Ag or W atoms, can be assigned to modes obtained by theoretical calculations. This kind of behavior was also associated with a tighter arrangement of ions in the unit cell. Therefore, it is important to mention that γ-Ag2WO4 is formed by two groups of distorted polyhedral (octahedral [AgO6] and tetrahedral [WO4] clusters). Hence, we assume that tungstate groups are partially disordered, giving rise to the large bandwidth increase observed.
From all displacements explored, we have considered those that agree well with the experimental results, such as the displacement of 50% of the 0.10 Å W atoms in the z-coordinate, as listed in Table S2.† The displacement of 50% of the W atoms generated the higher mode at 936.8 cm−1 corresponding to the symmetric stretching of only the distorted clusters (half of the [WO4] clusters), while the mode at 899.9 cm−1 refers to the symmetric stretching of the ordered clusters. As previously mentioned, the modes that appeared at 779.2 cm−1, 651.1 cm−1 and 589.5 cm−1 are related to the asymmetric stretching of the distorted [WO4] clusters, while the modes in the range 686–693 cm−1 are associated to the asymmetric stretching of the ordered clusters. Stretching modes in the range 580–940 cm−1 are depicted in Fig. 7b. It is worth noting that the stretching motion of the [WO4] clusters is not affected by the displacement of any percentage of Ag atoms, and therefore it is not appropriate to explain the observed experimental modes in the 750–850 cm−1 range. The relative positions of the theoretical and experimental Raman-active modes are displayed in Fig. 8, where it can be observed that there is good agreement between the experimental and calculated. However, the calculated frequencies are slightly overestimated (except for both bending of disordered structure, A1 mode, and stretching of both ordered, F2g mode, and disordered structures, B1, B2 and A1 modes) compared to experimental data. Hence, we assume that: (i) tungstate groups are partially disordered, giving rise to the large bandwidth increase in the Raman spectra, and (ii) these disordered tungstate groups are present in larger quantities than the disordered silver groups.
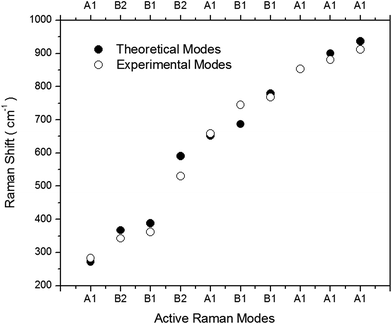 |
| Fig. 8 Comparison between the relative positions of the theoretical and experimental Raman-active modes. | |
3.5 PL spectra
PL emission spectra are dependent on the electronic structure of the material at hand, and they are a powerful probe for structural short and medium range order–disorder effects, such as clusters in the lattice. In Fig. 9, a prominent PL emission band is clearly seen with a maximum emission at 449 nm. This emission peak in the blue region is typically related to distorted [WO6] octahedral in the α- and β-Ag2WO4 crystals.9,15,35,45 However, in our samples, it can be related to distorted [WO4] tetrahedral. For the α-Ag2WO4 phase, another diffused emission in the red region, from 620 to 640 nm, can be attributed to the [AgOy] clusters.9,15 In our samples, any red peak obtained was an indication that the [AgO6] clusters were less prone to be distorted. This result is in agreement with the Raman spectroscopy results.
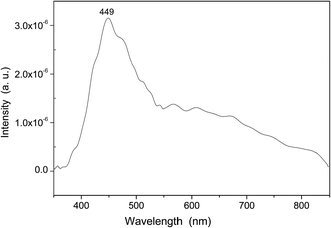 |
| Fig. 9 PL spectra of γ-Ag2WO4 microcrystals. | |
3.6 UV spectra
The determination of the optical band gap was obtained by combining Tauc's equation54 with the method proposed by Kubelka and Munk-Aussig,55 which is based on the transformation of diffuse-reflectance measurements, see ESI.† Tauc's equation is the following,where Egap is the optical energy gap, α is the optical absorption coefficient, hν is the photon energy, A is a constant, and n is a constant associated with different kinds of electronic transitions (n = 0.5 for a direct allowed and n = 2 for an indirect allowed). The band gap energy was estimated from the modified Kubelka–Munk equation, see ESI.† Fig. 10 shows the UV-visible diffuse reflectance spectra for γ-Ag2WO4. According to literature, the optical absorption spectrum in the silver tungstate family exhibits direct electronic transitions between the valence and conduction bands;9,13,56 therefore, in this work a n = 0.5 value was used in eqn (2). The band gap energy, estimated from the intercept of the tangent to the plot, was 3.21 eV.
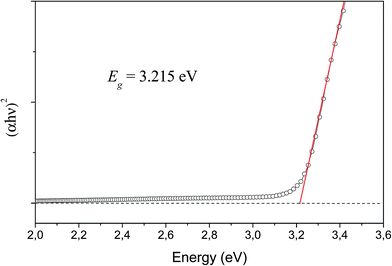 |
| Fig. 10 UV-vis spectra of γ-Ag2WO4 metastable. | |
For the theoretical band gap, we report an indirect band gap value of 4.37 eV from Γ to W (see Fig. 11), which serves as a guide to understand band gap trends, but does not directly correspond to the physically measured quantity. The difference between the experimental and theoretical band gap values can be associated to the short and medium-range distortions on the [AgO6] and [WO4] clusters. An analysis of the DOS projected on the atoms renders that the conduction band (CB) is mainly attributed to W atoms, while the valence band (VB) is mainly formed by O and Ag atoms. An analysis of the DOS projected on the orbitals shows that the top of the VB is formed by the hybridization of Ag 4d and O 2p orbitals, while the bottom of the CB is mainly formed by the hybridization of W 5d and O 2p orbitals.
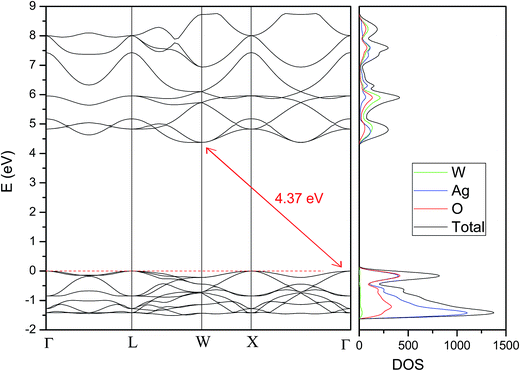 |
| Fig. 11 Band structure and DOS projected on γ-Ag2WO4 atoms. | |
The value of this difference, 1.16 eV, is bigger than the difference between experimental and theoretical values reported by our group for α- and β-, ∼0.40 and 0.40 eV, respectively.15,45,52 This agree with the suggestion that γ-Ag2WO4 is the polymorph more susceptible to be disordered.
4. Conclusions
The behavior of metastable phases is of substantial scientific and technological interest. The polymorphism of Ag2WO4 is a puzzling and important problem. In this work, metastable γ-Ag2WO4 crystals were prepared by the precipitation method, and an in-depth physicochemical characterization of these structures was successfully performed. The theoretical models and results, along with our experimental data, allowed us to analyze the structural, morphological, vibrational, and optical properties of the γ-Ag2WO4 phase.
Ordered and disordered models to describe the crystal structure are considered. Disordered [WO4] tetrahedral clusters were key to reproduce the linewidth of the bands appearing in the Raman spectra, while a prominent PL emission band with maximum emission in the blue region was related to these distorted [WO4] tetrahedra. In addition, it was found that γ-Ag2WO4 has an indirect band gap, and the calculated positions of the valence and conduction bands showed the presence of [WO4] tetrahedral and [AgO6] octahedral clusters. These distortions can facilitate the separation and migration of electron and hole pairs, they can improve the photocatalytic performance of γ-Ag2WO4, and they could be the source of the metastable character of this phase. The experimental morphologies of the as-synthesized samples are similar to the theoretically obtained shapes when the surface energy values for the (111) surface was decreased.
Finally, we point out how the combination of experimental techniques with theory is the first step to better understand the chemistry of the less-common γ-Ag2WO4 polymorph. Therefore, this study illustrates the potential of this joint strategy, which helps to open a promising path toward fundamental information regarding the geometry, cluster coordination, and electronic structure of γ-Ag2WO4 crystals. The availability of a facile method to prepare γ-Ag2WO4 is expected to catalyze further empirical studies on the elusive and little-studied γ-phase because of its varied potential applications.
Acknowledgements
This work was financially supported by the following Brazilian research funding institutions: Fundação de Amparo à Pesquisa do Estado de São Paulo (FAPESP; 2012/14004-5 and 2013/07296-2), Conselho Nacional de Desenvolvimento Científico e Tecnológico (CNPq; 479644/2012-8, 350711/2012-7, 304531/2013-8 and 151136/2013-0). Coordenação de Aperfeiçoamento de Pessoal de Nível Superior (CAPES). J. A. acknowledges also the financial support of the Spanish research funding projects: PrometeoII/2014/022 and ACOMP/2014/270 and ACOMP72015/1202 projects (Generalitat-Valenciana), Ministerio de Economia y Competitividad (CTQ2015-652017-P) and Programa de Cooperación Cientifíca con Iberoamerica (Brasil) of Ministerio de Educación (PHBP14-00020). J. A. acknowledges to Ministerio de Economia y Competitividad, “Salvador Madariaga” program, PRX15/00261. L. G. acknowledges Banco Santander (Becas Iberoamérica: Jóvenes profesores e investigadores). We also acknowledge the Servei Informática, Universitat Jaume I for a generous allotment of computer time.
References
- C. C. Chen, A. B. Herhold, C. S. Johnson and A. P. Alivisatos, Size dependence of structural metastability in semiconductor nanocrystals, Science, 1997, 276, 398–401 CrossRef CAS PubMed.
- M. Jansen, I. V. Pentin and J. C. Schoen, A Universal Representation of the States of Chemical Matter Including Metastable Configurations in Phase Diagrams, Angew. Chem., Int. Ed., 2012, 51, 132–135 CrossRef CAS PubMed.
- S. Shukla, S. Seal, R. Vij, S. Bandyopadhyay and Z. Rahman, Effect of nanocrystallite morphology on the metastable tetragonal phase stabilization in zirconia, Nano Lett., 2002, 2, 989–993 CrossRef CAS.
- U. Schubert and N. Hüsing, Synthesis of Inorganic Materials, Wiley-VCH Weinheim, 2012 Search PubMed.
- C. G. Levi, Metastability and microstructure evolution in the synthesis of inorganics from precursors, Acta Mater., 1998, 46, 787–800 CrossRef CAS.
- J. F. Liu, Q. H. Li, T. H. Wang, D. P. Yu and Y. D. Li, Metastable vanadium dioxide nanobelts: Hydrothermal synthesis, electrical transport, and magnetic properties, Angew. Chem., Int. Ed., 2004, 43, 5048–5052 CrossRef CAS PubMed.
- J. Lu, P. F. Qi, Y. Y. Peng, Z. Y. Meng, Z. P. Yang, W. C. Yu and Y. T. Qian, Metastable MnS crystallites through solvothermal synthesis, Chem. Mater., 2001, 13, 2169–2172 CrossRef CAS.
- J. Andrés, L. Gracia, P. Gonzalez-Navarrete, V. M. Longo, W. Avansi Jr, D. P. Volanti, M. M. Ferrer, P. S. Lemos, F. A. L. Porta, A. C. Hernandes and E. Longo, Structural and electronic analysis of the atomic scale nucleation of Ag on α-Ag2WO4 induced by electron irradiation, Sci. Rep., 2014, 5, 5391 Search PubMed.
- L. S. Cavalcante, M. A. P. Almeida, W. Avansi, R. L. Tranquilin, E. Longo, N. C. Batista, V. R. Mastelaro and M. S. Li, Cluster Coordination and Photoluminescence Properties of α-Ag2WO4 Microcrystals, Inorg. Chem., 2012, 51, 10675–10687 CrossRef CAS PubMed.
- X. J. Cui, S. H. Yu, L. L. Li, L. Biao, H. B. Li, M. S. Mo and X. M. Liu, Selective synthesis and characterization of single-crystal silver molybdate/tungstate nanowires by a hydrothermal process, Chem.–Eur. J., 2004, 10, 218–223 CrossRef CAS PubMed.
- Z. Lin, J. Li, Z. Zheng, J. Yan, P. Liu, C. Wang and G. Yang, Electronic Reconstruction of α-Ag2WO4 Nanorods for Visible-Light Photocatalysis, ACS Nano, 2015, 9, 7256–7265 CrossRef CAS PubMed.
- E. Longo, L. S. Cavalcante, D. P. Volanti, A. F. Gouveia, V. M. Longo, J. A. Varela, M. O. Orlandi and J. Andres, Direct in situ observation of the electron-driven synthesis of Ag filaments on α-Ag2WO4 crystals, Sci. Rep., 2013, 3, 1676 CAS.
- L. S. Cavalcante, V. M. Longo, J. C. Sczancoski, M. A. P. Almeida, A. A. Batista, J. A. Varela, M. O. Orlandi, E. Longo and M. S. Li, Electronic structure, growth mechanism and photoluminescence of CaWO4 crystals, CrystEngComm, 2012, 14, 853–868 RSC.
- C.-X. Guo, B. Yu, J.-N. Xie and L.-N. He, Silver tungstate: a single-component bifunctional catalyst for carboxylation of terminal alkynes with CO2 in ambient conditions, Green Chem., 2015, 17, 474–479 RSC.
- E. Longo, D. P. Volanti, V. M. Longo, L. Gracia, I. C. Nogueira, M. A. P. Almeida, A. N. Pinheiro, M. M. Ferrer, L. S. Cavalcante and J. Andres, Toward an Understanding of the Growth of Ag Filaments on α-Ag2WO4 and Their Photoluminescent Properties: A Combined
Experimental and Theoretical Study, J. Phys. Chem. C, 2014, 118, 1229–1239 CAS.
- E. Longo, W. Avansi Jr, J. Bettini, J. Andres and L. Gracia, In situ Transmission Electron Microscopy observation of Ag nanocrystal evolution by surfactant free electron-driven synthesis, Sci. Rep., 2016, 6, 21498 CrossRef CAS PubMed.
- J. W. Tang and J. H. Ye, Correlation of crystal structures and electronic structures and photocatalytic properties of the W-containing oxides, J. Mater. Chem., 2005, 15(39), 4246–4251 RSC.
- M. Vafaeezadeh and M. M. Hashemi, One pot oxidative cleavage of cyclohexene to adipic acid using silver tungstate nano-rods in a Bronsted acidic ionic liquid, RSC Adv., 2015, 5, 31298–31302 RSC.
- J. T.-W. Wang, J. M. Ball, E. M. Barea, A. Abate, J. A. Alexander-Webber, J. Huang, M. Saliba, I. Mora-Sero, J. Bisquert, H. J. Snaith and R. J. Nicholas, Low-Temperature Processed Electron Collection Layers of Graphene/TiO2 Nanocomposites in Thin Film Perovskite Solar Cells, Nano Lett., 2013, 14, 724–730 CrossRef PubMed.
- R. Zhang, H. Cui, X. Yang, H. Tang, H. Liu and Y. Li, Facile hydrothermal synthesis and photocatalytic activity of rod-like nanosized silver tungstate, Micro Nano Lett., 2012, 7, 1285–1288 CAS.
- X. Sang, P. Wang, L. Ai, Y. Li and J. Bu, Preparation of fine Ag2WO4 antibacterial powders and its application in the sanitary ceramics, Adv. Mater. Res., 2011, 284–286, 1321–1325 Search PubMed.
- L. F. da Silva, A. C. Catto, W. Avansi Jr, L. S. Cavalcante, J. Andres, K. Aguir, V. R. Mastelaro and E. Longo, A novel ozone gas sensor based on one-dimensional (1D) α-Ag2WO4 nanostructures, Nanoscale, 2014, 6, 4058–4062 RSC.
- V. M. Longo, C. C. De Foggi, M. M. Ferrer, A. F. Gouveia, R. S. Andre, W. Avansi, C. E. Vergani, A. L. Machado, J. Andres, L. S. Cavalcante, A. C. Hernandes and E. Longo, Potentiated Electron Transference in α-Ag2WO4 Microcrystals with Ag Nanofilaments as Microbial Agent, J. Phys. Chem. A, 2014, 118, 5769–5778 CAS.
- W. d. S. Pereira, J. Andres, L. Gracia, M. A. San-Miguel, E. Z. da Silva, E. Longo and V. M. Longo, Elucidating the real-time Ag nanoparticle growth on α-Ag2WO4 during electron beam irradiation: experimental evidence and theoretical insights, Phys. Chem. Chem. Phys., 2015, 17, 5352–5359 RSC.
- D. Xu, B. Cheng, J. Zhang, W. Wang, J. Yu and W. Ho, Photocatalytic activity of Ag2MO4 (M = Cr, Mo, W) photocatalysts, J. Mater. Chem. A, 2015, 3, 20153–20166 CAS.
- X.-Y. Zhang, J.-D. Wang, J.-K. Liu, X.-H. Yang and Y. Lu, Construction of silver tungstate multilevel sphere clusters by controlling the energy distribution on the crystal surface, CrystEngComm, 2015, 17, 1129–1138 RSC.
- A. J. van den Berg and C. A. H. Juffermans, The polymorphism of silver tungstate Ag2WO4, J. Appl. Crystallogr., 1982, 15, 114–116 CrossRef CAS.
- X. Wang, C. Fu, P. Wang, H. Yu and J. Yu, Hierarchically porous metastable beta-Ag2WO4 hollow nanospheres: controlled synthesis and high photocatalytic activity, Nanotechnology, 2013, 24, 165602–165609 CrossRef PubMed.
- J. Li, C. Yu, C. Zheng, A. Etogo, Y. Xie, Y. Zhong and Y. Hu, Facile formation of Ag2WO4/AgX (X = Cl, Br, I) hybrid nanorods with enhanced visible-light-driven photoelectrochemical properties, Mater. Res. Bull., 2015, 61, 315–320 CrossRef CAS.
- H. Chen and Y. Xu, Photoactivity and
stability of Ag2WO4 for organic degradation in aqueous suspensions, Appl. Surf. Sci., 2014, 319, 319–323 CrossRef CAS.
- Y. V. B. De Santana, J. E. C. Gomes, L. Matos, G. H. Cruvinel, A. Perrin, C. Perrin, J. Andres, J. A. Varela and E. Longo, Silver Molybdate and Silver Tungstate Nanocomposites with Enhanced Photoluminescence, Nanomater. Nanotechnol., 2014, 4, 1–10 CrossRef.
- P. H. Bottelberghs, E. Everts and G. H. J. Broers, Phase-Diagram and High Ionic-Conductivity of System Na2WO4–Ag2WO4, Mater. Res. Bull., 1976, 11, 263–268 CrossRef CAS.
- T. Takahashi, S. Ikeda and O. Yamamoto, Solid-State Ionics – New High Ionic Conductivity Solid Electrolyte Ag6I4WO4 and Use of This Compound in a Solid-Electrolyte Cell, J. Electrochem. Soc., 1973, 120, 647–651 CrossRef CAS.
- R. A. Roca, P. S. Lemos, J. Andrés and E. Longo, Formation of Ag nanoparticles on metastable β-Ag2WO4 microcrystals induced by electron irradiation, Chem. Phys. Lett., 2016, 644, 68–72 CrossRef.
- P. S. Lemos, A. Altomare, A. F. Gouveia, I. C. Nogueira, L. Gracia, R. Llusar, J. Andrés, E. Longo and L. S. Cavalcante, Synthesis and characterization of metastable β-Ag2WO4: an experimental and theoretical approach, Dalton Trans., 2016, 45, 1185–1191 RSC.
- S. K. Gupta, K. Sudarshan, P. S. Ghosh, S. Mukherjee and R. M. Kadam, Doping Induced Room Temperature Stabilization of Metastable β-Ag2WO4 and Origin of Visible Emission in α and β-Ag2WO4: Low Temperature Photoluminescence Studies, J. Phys. Chem. C, 2016, 120, 7265–7276 CAS.
- G. S. Silva, M. T. Fabbro, L. Gracia, L. P. S. d. Santos, J. Andrés, E. Condorcillo, H. Beltrán-Mir and E. Longo, Theoretical and Experimental Insight on Ag2CrO4 Microcrystals: Synthesis, Characterization and Photoluminescence Properties, Inorg. Chem., 2016, 55, 8961–8970 CrossRef CAS PubMed.
- R. C. d. Oliveira, L. Gracia, M. Assis, M. S. Li, J. Andrés, E. Longo and L. S. Cavalcante, Disclosing the electronic structure and optical properties of Ag4V2O7 crystals: Experimental and theoretical Insights, CrystEngComm, 2016, 18, 6303–6326 RSC.
- R. Dovesi, V. R. Saunders, C. Roetti, R. Orlando, C. M. Zicovich-Wilson, F. Pascale, B. Civalleri, K. Doll, N. M. Harrison, I. J. Bush, P. D'Arco, M. Llunel, M. Causà and Y. Noël, CRYSTAL14 User's Manual, Theoretical Chemistry Group: University of Turin, Italy, 2014 Search PubMed.
- F. Cora, A. Patel, N. M. Harrison, R. Dovesi and C. R. A. Catlow, An ab initio Hartree–Fock study of the cubic and tetragonal phases of bulk tungsten trioxide, J. Am. Chem. Soc., 1996, 118, 12174–12182 CrossRef CAS.
- Crystal, http://www.crystal.unito.it/basis-sets.php.
- J. Heyd, G. E. Scuseria and M. Ernzerhof, Hybrid functionals based on a screened Coulomb potential, J. Chem. Phys., 2003, 118, 8207–8215 CrossRef CAS.
- A. V. Krukau, O. A. Vydrov, A. F. Izmaylov and G. E. Scuseria, Influence of the exchange screening parameter on the performance of screened hybrid functionals, J. Chem. Phys., 2006, 125, 224106 CrossRef PubMed.
- J. Andrés, L. Gracia, A. F. Gouveia, M. M. Ferrer and E. Longo, Effects of surface stability on the morphological transformation of metals and metal oxides as investigated by first-principles calculations, Nanotechnology, 2015, 26, 405703–405713 CrossRef PubMed.
- R. A. Roca, A. F. Gouveia, P. S. Lemos, L. Gracia, J. Andrés and E. Longo, Formation of Ag Nanoparticles on β-Ag2WO4 through Electron Beam Irradiation: A Synergetic Computational and Experimental Study, Inorg. Chem., 2016, 55, 8661–8671 CrossRef CAS PubMed.
- J. Donohue and W. Shand, The Determination of the Interatomic Distances in Silver Molybdate, Ag2MoO4, J. Am. Chem. Soc., 1947, 69, 222–223 CrossRef CAS.
- L. Cheng, Q. Shao, M. Shao, X. Wei and Z. Wu, Photoswitches of One-Dimensional Ag2MO4 (M = Cr, Mo, and W), J. Phys. Chem. C, 2009, 113, 1764–1768 CAS.
- K. G. Bramnik and H. Ehrenberg, Study of the Na2O–MoO3 system. Na6Mo11O36 – a new oxide with anatase-related structure, and the crystal structures of Na2MoO4, Z. Anorg. Allg. Chem., 2004, 630, 1336–1341 CrossRef CAS.
- G. Wulff, On the question of speed of growth and dissolution of crystal surfaces, Z. Kristallogr., 1901, 34, 449–530 CAS.
- J. W. Gibbs, On the equilibrium of heterogeneous substances, Trans. Conn. Acad. Arts Sci., 1878, 3, 343–524 Search PubMed.
- C. Herring, Some Theorems on the Free Energies of Crystal Surfaces, Phys. Rev., 1951, 82, 87–93 CrossRef CAS.
- R. A. Roca, J. C. Sczancoski, I. C. Nogueira, M. T. Fabbro, H. C. Alves, L. Gracia, L. P. S. Santos, C. P. de Sousa, J. Andrés, G. E. Luz Jr, E. Longo and L. S. Cavalcante, Facet-dependent photocatalytic and antibacterial properties of α-Ag2WO4 crystals: combining experimental data and theoretical insights, Catal. Sci. Technol., 2015, 5, 4091–4105 CAS.
- J. R. Ferraro, K. Nakamoto and C. W. Brown., Introductory Raman Spectroscopy, Academic Press, Amsterdam, 2nd edn, 2003 Search PubMed.
- J. Tauc, Optical Properties and Electronic Structure of Amorphous, Mater. Res. Bull., 1968, 3, 37–46 CrossRef CAS.
- P. Kubelka and F. Munk, Ein Beitrag Zur Optik Der Farbanstriche, Z. Med. Phys., 1931, 12, 593–601 Search PubMed.
- D. Y. Kim, S. H. Im and O. O. Park, Synthesis of Tetrahexahedral Gold Nanocrystals with High-Index Facets, Cryst. Growth Des., 2010, 10, 3321–3323 CAS.
Footnote |
† Electronic supplementary information (ESI) available. See DOI: 10.1039/c6ra24692c |
|
This journal is © The Royal Society of Chemistry 2017 |
Click here to see how this site uses Cookies. View our privacy policy here.