DOI:
10.1039/C6RA24476A
(Paper)
RSC Adv., 2016,
6, 108583-108589
Synthesis of Fe3O4/P(St-AA) nanoparticles for enhancement of stability of the immobilized lipases
Received
1st October 2016
, Accepted 9th November 2016
First published on 9th November 2016
Abstract
Core–shell Fe3O4/P(St-AA) (Fe3O4/polystyrene–poly acrylic acid) nanoparticles modified with carboxylic acid groups were synthesized and employed as magnetic carriers for Candida rugosa lipase (CRL) and porcine pancreas lipase (PPL) immobilization. The loading amount of CRL on the Fe3O4/P(St-AA) nanoparticles was calculated to be 87.7 mg protein per g particles. Effects of solution pH and reaction temperature on the relative activity of immobilized CRL were measured and compared with the free CRL. The hydrolytic kinetics of the free and immobilized CRL was also investigated. Hydrolytic activity tests indicated that the stability of the immobilized CRL was strengthened. It should be mentioned that the immobilized CRL retained more than 56.1% of its initial activity after reuse 10 times. Most importantly, the immobilized PPL can tolerate organic media and maintain high esterification activity in n-hexane solution. This work provided a facile and general approach to synthesize magnetic functional nanocomposites for applications in biological and biocatalytic fields.
Introduction
Biocatalysts exhibit high effectiveness, high specificity and only require mild reaction conditions and have attracted tremendous attention with the rapid development of green and sustainable chemistry.1 As one of the most extensively used biocatalysts, lipases have very broad substrate specificity and are therefore used in a wide range of applications, both in research laboratories and in industry.2–4 However, lipases do not fulfill all the requirements of an industrial biocatalyst, limiting their practical industrial applications.5 An industrial biocatalyst should be heterogeneous, reasonably stable under conditions that may be quite far from their physiological environment and retain high catalytic activity when acting with non-physiological substrates.6 Immobilization of enzyme molecules on solid supports is considered to be an effective strategy to improve the handling, stability and recyclability of lipases.7–11 So, various different kinds of carriers, including magnetic microspheres, chitosan beads and porous silica beads, have been developed to immobilize lipases.12–16 Unfortunately, most of the reported supports have only a small number of active chemical sites exposed on the surface, which is obviously disadvantageous with regard to the lipases immobilization.17 Therefore, the development of a strategy for the fabrication of simple and efficient carriers is of vital importance for the immobilization of lipases.
As a kind of conventional magnetic material, magnetite (Fe3O4) nanoparticles are one of the most promising platforms owing to their unique magnetic properties, ready availability, and environmentally friendly merits.18,19 Correspondingly, considerable attention has been paid to the fabrication of magnetite nanostructures with different morphologies, such as nanoparticles, nanocubes, nanowires, nanotubes, nanorods, nanoplates, octahedra, rhombic dodecahedra and other polyhedral.20–24 However, the applications of such magnetic materials are limited by the agglomeration into bigger objects and the sensitivity to acidic and oxidative conditions,25 which result in losing the specific properties associated with their nanometre dimensions. Coating an outer protective layer is an effective strategy to maintain the stability of Fe3O4 nanoparticles. A remarkable approach to achieve this is enveloping Fe3O4 nanoparticles by a polymer shell to form core–shell structured nanocomposites, which can extend their technical applications as a result of unique characteristics of polymer shells including high stability in extreme conditions and a platform for chelating groups. So, various methods have been proposed for the fabrication of magnetic polymers, including Fe3O4/polystyrene, Fe3O4/poly(acrylic acid), Fe3O4@polystyrene/poly(glycidyl methacrylate), Fe3O4@poly(acrylic acid)/chitosan, polystyrene–poly(acrylic acid)/Fe3O4/poly(acrylic acid), and so on.26–34 However, most of the reported syntheses are complicated and not eco-friendly.35 Therefore, there is still challenge that needs to be overcome for the development of more facile routes, especially green methods.
Herein, we fabricated magnetic Fe3O4/P(St-AA) nanoparticles with a core–shell structure for CRL and PPL immobilization. The immobilization of CRL on the core–shell magnetic polymers was conducted and the catalytic performance of the immobilized CRL on the Fe3O4/P(St-AA) nanoparticles was then investigated by the hydrolysis of p-nitrophenyl palmitate (pNPP) in phosphate buffer. Thermal deactivation indicated that the thermal stability of the immobilized CRL was strengthened. It was found that the immobilized CRL retained more than 56.1% catalytic activity after 10 times reuse. Additionally, the immobilized PPL can tolerate organic media and keep high esterification activity in n-hexane solution.
Experimental
Materials
CRL, PPL (Type VI-S), N-hydroxysuccinimide (NHS), 1-(3-dimethylaminopropyl)-3-ethylcarbodiimide hydrochloride (EDC·HCl), pNPP, p-nitrophenol (pNP), Coomassie Brilliant Blue G-250 and bovine serum albumin (BSA) protein were purchased from Sigma-Aldrich. Fe(acac)3 (99.9%), oleic acid (90%), oleylamine (>70%), phenyl ether, dodecanol, 2(N-morpholino)ethane sulfonic acid (MES), potassium persulfate (KPS) were purchased from Aladdin Chemical Co. Ltd. (Shanghai, China). St and AA were purchased from Sinopharm Chemical Reagent Co., Ltd. (Shanghai, China) and were purified by vacuum distillation. All other chemicals and reagents were analytical grade, purchased from Sinopharm Chemical Reagent Co., Ltd. (Shanghai, China), and used without further purification. Deionized water was used throughout.
Preparation of Fe3O4 nanoparticles
Fe(acac)3 (2 mmol) was mixed in phenyl ether (20 mL) with ethylene glycol (1 mL), oleic acid (6 mmol), and oleylamine (6 mmol) under nitrogen atmosphere and was heated to 200 °C for 30 min. Subsequently, the mixture was refluxed for 2 h under nitrogen atmosphere to obtain Fe3O4 nanoparticles. The as-obtained black precipitate was collected and washed with ethanol 2 times for future use.
Preparation of Fe3O4/P(St-AA) nanoparticles
The as-prepared Fe3O4 nanoparticles was mixed in 36 mL water with 0.5 mL dodecanol and 150 mg sodium dodecyl sulfate (SDS), and was treated by ultrasound for 15 min with 650 W protected by ice-water bath to obtain Fe3O4 emulsion. SDS (70 mg) was mixed in water (30 mL) with dodecanol (0.1 mL), St (0.1 mL) and AA (0.4 mL) were mixed by 20 min of ultrasonic bath to obtain the monomer emulsion. Fe3O4 emulsion, monomer emulsion and 45 mg KPS were added to three-neck flask and stirred for 30 min at 400 rpm in nitrogen atmosphere. Then the reactor was placed in 80 °C water bath for 24 h to obtain Fe3O4/P(St-AA) nanoparticles suspension. Subsequently, the as-obtained Fe3O4/P(St-AA) nanoparticles were collected, washed with ethanol (25%, v/v) and distilled water several times, and dried in a vacuum oven at 30 °C for 8 h.
Immobilization of lipases
The as-prepared Fe3O4/P(St-AA) nanoparticles (100 mg) were dispersed in 20 mL of MES buffer (0.1 M, pH 6.0) containing 191.7 mg EDC·HCl and 115.1 mg NHS by sonication for 2 min. Subsequently, the mixture was incubated at 30 °C in a shaker operating at 160 times per min for 2 h. After the incubation, the Fe3O4/P(St-AA) nanoparticles were washed 2 times with phosphate buffer (0.1 M, pH 7.0), then dispersed in 40 mL phosphate buffer (0.1 M, pH 7.0) containing 40 mg CRL for the covalent attachment of the enzyme molecules onto the particle surface. After 1.5 h of the reaction, the final samples were recovered by magnetic separation, washed with 30 mL phosphate buffer (0.1 M, pH 7.0) 3 times to remove excess CRL, and stored at 4 °C until they were used for activity related tests. Using the same procedures, immobilized PPL was synthesized. The loading of CRL molecules on Fe3O4/P(St-AA) nanoparticles was analysed by the Bradford method using BSA as a standard. The amount of immobilized CRL was calculated by subtracting the amount of free CRL from the total amount of the CRL used for the immobilization. The mass ratio percentage of immobilized CRL to Fe3O4/P(St-AA) nanoparticles was reported as the loading capacity percentage (w/w%).
Lipases activity assay
The enzymatic activities of free and immobilized CRL were assayed by the hydrolysis of pNPP in phosphate buffer (0.1 M).12 The concentration of the hydrolysis product (pNP) was measured using a UV 757 CRT spectrophotometer at 410 nm.36 By taking the maximum activity value of the free and immobilized CRL under optimal conditions as 100%, the activities obtained from other conditions were expressed as relative activities. The relative activity was defined as the ratio of the residual activity to the initial activity. Each data was the average of three parallel experiments at least. The standard error for the result was below 5% of the average value.
The effects of temperature on the activities of free and immobilized CRL were investigated at pH 7.0 under the variety of temperature. The effects of pH on the activities of free and immobilized CRL were investigated at 40 °C under the variety of pH. Thermal stabilities of free and immobilized CRL were studied by measuring the residual activities of the CRL after incubation at 55 °C for 1–10 h.
The esterification activity of immobilized PPL in organic solvents was carried out in 5 mL n-hexane solution containing 2 mmol n-butyl alcohol and 2 mmol carboxylic acid at 45 °C in a shaker operating at 160 times per min for 30 min. After collecting nanoparticles with a magnet, the supernatant was removed to determine the conversions of the starting butyl alcohol by a gas chromatography-mass spectrometry (GC-MS, Shimadzu QP2010 Plus) system using an internal standard.
Characterization
The powder X-ray diffraction (XRD) pattern of the products was recorded on a Shimadzu XRD-6000 X-ray diffractometer. The morphologies of the samples were investigated by scanning electron microscopy (SEM) and transmission electron microscopy (TEM) using a Hitachi S-4800 field emission scanning electron microscope and a JEM-2010 high-resolution transmission electron microscope, respectively. X-Ray photoelectron spectroscopy (XPS) analysis of the surface was carried out using an ESCALAB 250Xi spectrometer (Thermo Scientific) equipped with an Al Kα X-ray radiation source. Water contact angles measurements were conducted by contact angle measurement instrument (JCY-4, Shanghai Fangrui Instrument Co. Ltd.). Before measurements, a small amount of Fe3O4/P(St-AA) nanoparticles was pressed at 5 MPa into a disc. Fourier transform infrared spectroscopy (FT-IR) spectra were obtained on a Vectortm 22. The magnetic properties of the products were collected with a superconducting quantum interference device (SQUID, Quantum Design MPMS3) at 300 K.
Results and discussion
The synthesis of Fe3O4/P(St-AA) nanoparticles has been achieved in the solution containing Fe3O4, dodecanol, sodium dodecyl sulphate, St and AA by a free-radical polymerization process. Fig. 1 shows typical XRD patterns of the as-prepared samples. All diffraction peaks of the Fe3O4 and Fe3O4/P(St-AA) samples could be readily assigned to the face-centered cubic (fcc) Fe3O4 phase by comparison with the Joint Committee on Powder Diffraction Standards (JCPDS) file no. 77-1545. It is clearly seen that the intensities of various peaks of Fe3O4/P(St-AA) samples are much lower than those of Fe3O4 nanoparticles, suggesting that Fe3O4 nanoparticles are encapsulated in a polymer shell. Calculations with the Debye–Scherrer equation (d = 0.89λ/B
cos
θ) for the five strongest peaks (220, 311, 400, 511, and 440) gave mean grain sizes of 17.2 and 16.7 nm for the Fe3O4 and Fe3O4/P(St-AA) nanoparticles, respectively.
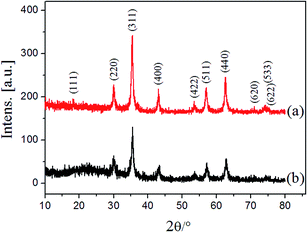 |
| Fig. 1 XRD patterns of (a) Fe3O4 and (b) Fe3O4/P(St-AA) nanoparticles. | |
The typical SEM image of the Fe3O4/P(St-AA) nanoparticles in Fig. 1a reveals that the as-obtained products is in the form of a spherical aggregates with average diameter of 30–60 nm. The dark/light contrast clearly observed in Fig. 2b suggests a different phase composition and indicates the formation of Fe3O4/P(St-AA) core–shell nanostructures. It is clearly seen that the Fe3O4 nanoparticles are completely encapsulated in a polymer shell and that there are no obvious voids between the core and the shell. Fe3O4 cores encapsulated in the polymer shell exhibit a clear spherical shape. Selected-area electron diffraction (SAED) in Fig. 2c of the prepared Fe3O4/P(St-AA) nanocomposites confirmed that they are cubic magnetite. The high-resolution TEM (HRTEM) image shown in Fig. 2d provides detailed structural information about the Fe3O4/P(St-AA) core–shell nanostructures. The lattice fringe calculated from the HRTEM image is 0.30 nm, which fits well with the (220) planes of a cubic Fe3O4 structure. In addition, disordered polymer layers are observed on the outside of the Fe3O4 nanoparticles.
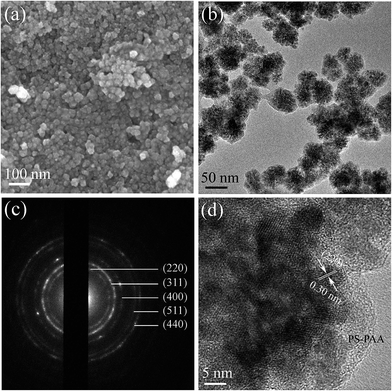 |
| Fig. 2 (a) SEM and (b) TEM, (c) SAED and (d) HRTEM images of Fe3O4/P(St-AA) nanoparticles. | |
The typical C 1s, O 1s, and Fe 2p X-ray photoelectron spectroscopy (XPS) survey spectra of the Fe3O4/P(St-AA) nanocomposites are shown in Fig. 3a–c. The asymmetric C 1s peak was decomposed into three peaks at 284.6, 285.8, and 288.4 eV. The peaks centred at 284.6 and 285.8 eV can be ascribed to carbons in aromatic and aliphatic part of the PS.37,38 The peaks located at 288.4 eV is ascribed to carbons in C
O bond.39 The O 1s peak can be fitted to three peaks at 529.9 (O in Fe3O4), 531.5 (O in C
O) and 533.0 eV (O in O–H),40,41 which indicates the presence of –COOH groups. At the same time, Fe 2p1/2 and Fe 2p3/2 peaks are found to be located at 724.1 and 710.8 eV. No Fe 2p signals of γ-Fe2O3 can be clearly identified on the magnetic samples, indicating that the product is Fe3O4 rather than γ-Fe2O3.41 Fig. 3d shows the wettability of a water droplet on the surface of a Fe3O4/P(St-AA) disc that was prepared by pressing Fe3O4/P(St-AA) particles. The static contact angle of water is 127.4 ± 2.0°, indicating that Fe3O4/P(St-AA) nanocomposites are hydrophobic in nature. The contact angle of the nanocomposites is smaller than that of Fe3O4/PS nanoparticles,42 suggesting the as-prepared Fe3O4/P(St-AA) nanoparticles is more hydrophilic.
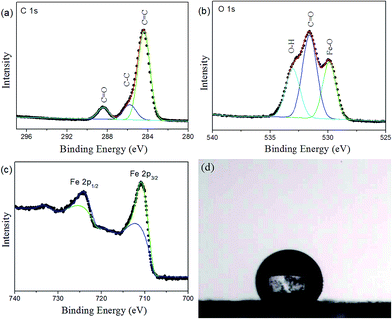 |
| Fig. 3 (a) C 1s, (b) O 1s and (c) Fe 2p XPS spectra of Fe3O4/P(St-AA) nanoparticles, and (d) image of a water droplet sitting on Fe3O4/P(St-AA) nanoparticles. | |
The surface information of the magnetic nanoparticles was further determined by FT-IR analysis. The strong peak at 584 cm−1 corresponds to the characteristic band of Fe–O vibration.43 In Fig. 4a, apart from the peaks (2924, 2853, 1596, 1452, 1421, 1056 and 653 cm−1) corresponding to the benzene ring and methylene which result from the polymer shell, the broad band around 3407 cm−1 can be assigned to the O–H stretching vibrations of carboxyl groups.37 More importantly, a relatively weak band around 1715 cm−1 and the small bands around 1205 cm−1, which can be separately assigned to the C
O and C–O stretching vibration of carboxyl groups,37,43 consolidate the carboxyl-functionalized polymer shells. The weakness in intensity of the bands corresponding to carboxyl groups might be due to the disturbance of benzene rings to carboxyl groups. In order to confirm the presentation of carboxyl groups on the surface of the nanoparticles, CRL molecules can react with the activated Fe3O4/P(St-AA) nanoparticles that was prepared by incubating Fe3O4/P(St-AA) nanoparticles with EDC·HCl and NHS solution. It is observed that the signals at 1629 and 1397 cm−1 for –NH2 bending vibration and C–N stretching vibration appeared (Fig. 4b), indicating that CRL molecules was covalently bonded to the carboxyl-functionalized magnetic nanocomposites.
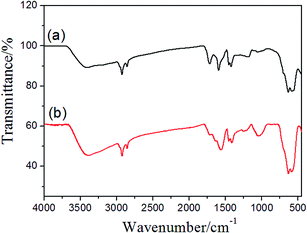 |
| Fig. 4 FT-IR spectra of (a) Fe3O4/P(St-AA) nanoparticles and (b) the immobilized CRL on the Fe3O4/P(St-AA) nanoparticles. | |
The room-temperature magnetic properties of Fe3O4/P(St-AA) nanoparticles were investigated by a SQUID magnetometer with an applied magnetic field ranging from −70 to 70 kOe. The typical ferromagnetic curves (Fig. 5) gave coercivity values of 34.7 Oe for the Fe3O4/P(St-AA) nanoparticles. The magnetic hysteresis plots show that the magnetizations of Fe3O4/P(St-AA) nanoparticles do not saturate in the range of field measured, suggesting the anisotropic effect of the magnetic Fe3O4/P(St-AA) samples. The saturation of the magnetization along the easy axis was observed at 1.5 kOe, whereas the magnetization along the hard axis was still unsaturated at ca. 70 kOe. The observed magnetic anisotropy of the Fe3O4/P(St-AA) samples may be attributed to their structural anisotropy.44 The magnetic separability of the Fe3O4/P(St-AA) nanoparticles was examined in aqueous solution by placing a magnet near the glass bottle. The black particles were attracted toward the magnet and the suspended aqueous solution turned transparent within 20 s, revealing that the Fe3O4/P(St-AA) nanoparticles possess magnetic properties. This will provide an easy and efficient way to separate the Fe3O4/P(St-AA) nanoparticles from a suspension system.
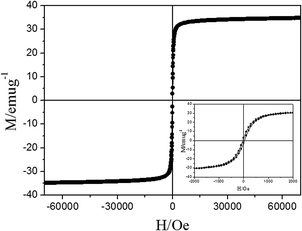 |
| Fig. 5 Magnetic hysteresis loops and enlarged view of Fe3O4/P(St-AA) nanoparticles. | |
To investigate the bioapplication of the Fe3O4/P(St-AA) nanoparticles, lipases were connected onto the surfaces of magnetic nanocomposites via an immobilization process. According the Bradford method, the loading amount of CRL on the Fe3O4/P(St-AA) nanoparticles was calculated to be 87.7 mg protein per g particles. Fig. 6a shows the effects of pH values of the reaction systems on the hydrolytic activities of free and immobilized CRL. The maximum activity was at pH 7.0 for immobilized CRL, which was the same as free CRL. It was found that the immobilized CRL retained higher activities than free CRL over most of the pH values, which indicated that immobilized CRL showed preferable pH adaptability. Fig. 6b displays the effects of reaction temperature on the catalytic activities of free and immobilized CRL. According to Fig. 6b, the optimum temperature of the free and immobilized CRL was 40 °C. Importantly, at higher temperature the immobilized CRL showed higher activity, indicating that the immobilized CRL had better heat resistance than the free one. Kinetic constants of free and immobilized CRL were estimated from the Michaelis–Menten equation45 and listed in Table 1. Compared with the Km value of the free CRL (0.057 mM), the Km value of immobilized CRL increased to 0.145 mM; while the Vmax value of free CRL (0.353 U mg−1 protein) was found to be higher than that of immobilized CRL (0.175 U mg−1 protein). The increase in Km and decrease in Vmax of the immobilized CRL indicated that the immobilized CRL has a lower affinity to its substrate than that of free CRL, which may be due to the steric hindrance of the active site caused by supports, the loss of enzyme flexibility necessary for substrate binding, or diffusional resistance to solute transport around the particles.45
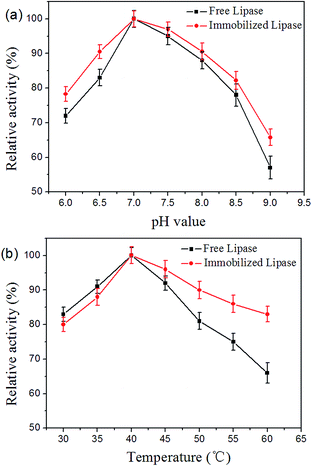 |
| Fig. 6 Effects of (a) pH value and (b) temperature on the activities of free and immobilized CRL. | |
Table 1 Kinetic properties of the free and immobilized CRL
Form of CRL |
Km (mM) |
Vmax (U mg−1 protein) |
R2 |
Free CRL |
0.057 |
0.353 |
0.9934 |
Immobilized CRL |
0.145 |
0.175 |
0.9915 |
For practical applications, it is usually required that immobilized lipases should tolerate relatively tough environmental conditions and keep catalytic activity at a high temperature. Therefore, the thermal deactivation of the free and immobilized CRL were studied by measuring the residual activities of CRL after incubation in phosphate buffer (pH 7.0) at 55 °C for 10 h with continuous shaking. Fig. 7 shows the comparison of thermal stabilities of free and immobilized CRL. The residual activity of immobilized CRL decreased less and more slowly than the free one. It is observed that free CRL lost its activity within 7 h. However, the immobilized CRL retained their initial activity of 61.8% after 10 h of incubation. These results demonstrated that the thermal stability of immobilized CRL was much better than the free one, which could be explained by the magnetic carriers enhancing the CRL rigidity, protected it from unfolding and prevented the conformation transition of the CRL at high temperature.46,47 The reusability of the immobilized CRL determined by hydrolysis of pNPP by the recovered immobilized CRL with magnetic separation and compared with the first running (activity defined as 100%). As shown in Fig. 8, the residual activity of the immobilized CRL decreased about 26.4% catalytic activity in the first 2 cycles. The decrease of enzyme's activity is mainly ascribed to the leakage of CRL from supports upon use. It should be mentioned that the immobilized CRL shows good stability in the next 5 consecutive reuses. The immobilized CRL retains more than 56.1% of its activity even in the case of 10 consecutive reuses, which is similar to that of the reported immobilized CRL.36,48,49
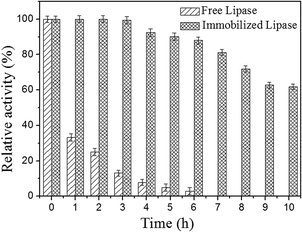 |
| Fig. 7 Thermal stabilities of free and immobilized CRL at 55 °C. | |
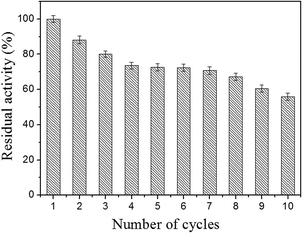 |
| Fig. 8 Reusability of the immobilized CRL. | |
The majority of applications of lipases in organic media are in the synthesis of flavour and fragrance esters for food applications because the products can be labelled natural, which is not the case when a chemical catalyst is used.50 In order to investigate the application of the Fe3O4/P(St-AA) carriers in organic media, PPL was connected onto the surfaces of magnetic carriers using a similar immobilization process. Ester synthesis of immobilized PPL in organic solvents was carried out in 5 mL n-hexane solution containing 0.4 M n-butyl alcohol and 0.4 M carboxylic acid. It is observed that the yield of butyl acetate was about 78% when acetic acid was employed in the esterification reaction. If acetic acid was substituted by propanoic acid, the yield of butyl propionate was decrease to 71%. These results reveal that the immobilized PPL could tolerate organic media and keep catalytic activity in the synthetic system.
Conclusions
This work described an easy and practical approach to synthesis Fe3O4/P(St-AA) nanoparticles for CRL and PPL immobilization. Morphologies, structures and properties of the Fe3O4/P(St-AA) nanoparticles were investigated by a series of modern analytical tools. Combining the merits of the easy separation of Fe3O4 core and carboxyl groups of polymer shell, the Fe3O4/P(St-AA) nanoparticles has been demonstrated to be a valid platform for lipase immobilization. The CRL loading amount on the Fe3O4/P(St-AA) nanoparticles was calculated to be 87.7 mg protein per g particles. The factors related to the catalytic activity of the immobilized CRL were measured and compared with the free CRL. The kinetics of hydrolytic activity of the free and immobilized CRL was also investigated. Hydrolytic activity tests indicate that the stability of the immobilized CRL was strengthened. The immobilized CRL retained more than 56.1% of its initial activities after 10 times reuse. Most importantly, the immobilized PPL could tolerate organic media and keep high esterification activity in n-hexane solution. Though further development is still needed, the as-prepared Fe3O4/P(St-AA) nanoparticles can now be considered as a novel magnetic carries for enzymes immobilization.
Acknowledgements
This work is supported by the National Natural Science Foundation of China (21471002 and 51572004), the opening research foundations of State Key Laboratory of Coordination Chemistry of Nanjing University, and the Outstanding Youth Science Foundation of Anhui Polytechnic University (2015JQ01).
Notes and references
- M. Held, A. Schmid, J. B. van Beilen and B. Witholt, Pure Appl. Chem., 2000, 72, 1337–1343 CrossRef CAS.
- R. D. Schmid and R. Verger, Angew. Chem., Int. Ed., 1998, 37, 1608–1633 CrossRef.
- R. Sangeetha, I. Arulpandi and A. Geetha, Res. J. Microbiol., 2011, 6, 1–24 CrossRef CAS.
- A. K. Singh and M. Mukhopadhyay, Appl. Biochem. Biotechnol., 2012, 166, 486–520 CrossRef CAS PubMed.
- R. C. Rodrigues, Á. Berenguer-Murcia and R. Fernández-Lafuente, Adv. Synth. Catal., 2011, 353, 2216–2238 CrossRef CAS.
- K. Hernandez and R. Fernandez-Lafuente, Enzyme Microb. Technol., 2011, 48, 107–122 CrossRef CAS PubMed.
- S. Cantone, V. Ferrario, L. Corici, C. Ebert, D. Fattor, P. Spizzo and L. Gardossi, Chem. Soc. Rev., 2013, 42, 6262–6276 RSC.
- R. C. Rodrigues, C. Ortiz, Á. Berenguer-Murcia, R. Torres and R. Fernández-Lafuente, Chem. Soc. Rev., 2013, 42, 6290–6307 RSC.
- R. A. Sheldon and S. van Pelt, Chem. Soc. Rev., 2013, 42, 6223–6235 RSC.
- J. C. S. dos Santos, O. Barbosa, C. Ortiz, A. Berenguer-Murcia, R. C. Rodrigues and R. Fernandez-Lafuente, ChemCatChem, 2015, 7, 2413–2432 CrossRef CAS.
- C. Garcia-Galan, Á. Berenguer-Murcia, R. Fernandez-Lafuente and R. C. Rodrigues, Adv. Synth. Catal., 2011, 353, 2885–2904 CrossRef CAS.
- M. Kalantari, M. Kazemeini, F. Tabandeh and A. Arpanaei, J. Mater. Chem., 2012, 22, 8385–8393 RSC.
- M. Hartmann and D. Jung, J. Mater. Chem., 2010, 20, 844–857 RSC.
- M. C. R. Franssen, P. Steunenberg, E. L. Scott, H. Zuilhof and J. P. M. Sanders, Chem. Soc. Rev., 2013, 42, 6491–6533 RSC.
- Y. J. Li, G. W. Zhou, W. T. Qiao and Y. Y. Wang, Mater. Sci. Eng., B, 2009, 162, 120–126 CrossRef CAS.
- W. Z. Shen, L. W. Ren, H. Zhou, S. C. Zhang and W. B. Fan, J. Mater. Chem., 2011, 21, 3890–3894 RSC.
- X. H. Li, H. Zhu, J. Feng, J. W. Zhang, X. Deng, B. F. Zhou, H. L. Zhang, D. S. Xue, F. S. Li, N. J. Mellors, Y. F. Li and Y. Peng, Carbon, 2013, 60, 488–497 CrossRef CAS.
- B. J. Li, H. Q. Cao, J. Shao and M. Z. Qu, Chem. Commun., 2011, 47, 10374–10376 RSC.
- Z. J. Zhou, X. L. Zhu, D. J. Wu, Q. L. Chen, D. T. Huang, C. J. Sun, J. Y. Xin, K. Y. Ni and J. H. Gao, Chem. Mater., 2015, 27, 3505–3515 CrossRef CAS.
- Z. H. Qin, X. L. Jiao and D. R. Chen, CrystEngComm, 2011, 13, 4646–4651 RSC.
- W. Cheng, K. B. Tang, Y. X. Qi, J. Sheng and Z. P. Liu, J. Mater. Chem., 2010, 20, 1799–1805 RSC.
- X. Y. Li, D. P. Liu, S. Y. Song, X. Wang, X. Ge and H. J. Zhang, CrystEngComm, 2011, 13, 6017–6020 RSC.
- X. L. Cheng, J. S. Jiang, D. M. Jiang and Z. J. Zhao, J. Phys. Chem. C, 2014, 118, 12588–12598 CAS.
- Z. M. Chen, Y. Sun, W. D. Zhang, T. Yang, L. Chen, R. C. Yang and N. Zhou, CrystEngComm, 2016, 18, 3124–3129 RSC.
- J. H. Zhang, B. Yan and F. Zhang, CrystEngComm, 2012, 14, 3451–3455 RSC.
- S. Xu, Z. H. Weng, J. Tan, J. Guo and C. C. Wang, Polym. Chem., 2015, 6, 2892–2899 RSC.
- L. H. Yu, G. Z. Hao, J. J. Gu, S. Zhou, N. Zhang and W. Jiang, J. Magn. Magn. Mater., 2015, 394, 14–21 CrossRef CAS.
- F. F. Fang, J. H. Kim and H. J. Choi, Polymer, 2009, 50, 2290–2293 CrossRef CAS.
- Y. Y. Xu, M. Zhou, H. J. Geng, J. J. Hao, Q. Q. Ou, S. D. Qi, H. L. Chen and X. G. Chen, Appl. Surf. Sci., 2012, 258, 3897–3902 CrossRef CAS.
- F. Gao, H. B. Gu, H. W. Wang, X. F. Wang, B. Xiang and Z. H. Guo, RSC Adv., 2015, 5, 60208–60219 RSC.
- L. Tian, B. L. Zhang, W. Li, X. J. Li, X. L. Fan, X. K. Jia, H. P. Zhang and Q. Y. Zhang, RSC Adv., 2014, 4, 27152–27158 RSC.
- Y. Y. Li, D. Y. Yuan, M. J. Dong, Z. H. Chai and G. Q. Fu, Langmuir, 2013, 29, 11770–11778 CrossRef CAS PubMed.
- X. H. Zhan, L. Q. Xie, H. L. Chen, Y. Wu and Z. W. Gu, RSC Adv., 2016, 6, 14077–14083 RSC.
- L. Q. Xie, S. H. Ma, Q. Yang, F. Lan, Y. Wu and Z. W. Gu, RSC Adv., 2014, 4, 1055–1061 RSC.
- S. K. Li, F. Z. Huang, Y. Wang, Y. H. Shen, L. G. Qiu, A. J. Xie and S. J. Xu, J. Mater. Chem., 2011, 21, 7459–7466 RSC.
- X. Wang, P. P. Dou, P. Zhao, C. M. Zhao, Y. Ding and P. Xu, ChemSusChem, 2009, 2, 947–950 CrossRef CAS PubMed.
- H. H. Jiang, X. Y. Han, Z. L. Li, X. C. Chen, Y. H. Hou, L. G. Gai, D. C. Li, X. R. Lu and T. L. Fu, Colloids Surf., A, 2012, 401, 74–80 CrossRef CAS.
- D. E. Weibel, F. Kessler and G. V. da S. Mota, Polym. Chem., 2010, 1, 645–649 RSC.
- C. T. Hsieh, H. Teng, W. Y. Chen and Y. S. Cheng, Carbon, 2010, 48, 4219–4229 CrossRef CAS.
- J. S. Shaikh, R. C. Pawar, S. S. Mali, A. V. Moholkar, J. H. Kim and P. S. Patil, J. Solid State Electrochem., 2012, 16, 25–33 CrossRef CAS.
- Y. Tian, B. B. Yu, X. Li and K. Li, J. Mater. Chem., 2011, 21, 2476–2481 RSC.
- J. J. Zhang and Z. L. Lei, Appl. Surf. Sci., 2012, 258, 5080–5085 CrossRef CAS.
- J. Hong, D. M. Xu, P. J. Gong, H. J. Ma, L. Dong and S. D. Yao, J. Chromatogr. B: Anal. Technol. Biomed. Life Sci., 2007, 850, 499–506 CrossRef CAS PubMed.
- Y. Song, S. Ohkoshi, Y. Arimoto, H. Seino, Y. Mizobe and K. Hashimoto, Inorg. Chem., 2003, 42, 1848–1856 CrossRef CAS PubMed.
- M. Y. Chang and R. S. Juang, Enzyme Microb. Technol., 2005, 36, 75–82 CrossRef CAS.
- Y. Yong, Y. X. Bai, Y. F. Li, L. Lin, Y. J. Cui and C. G. Xia, Process Biochem., 2008, 43, 1179–1185 CrossRef CAS.
- H. Zhu, C. Hou, Y. J. Li, G. H. Zhao, X. Liu, K. Hou and Y. F. Li, Chem.–Asian J., 2013, 8, 1447–1454 CrossRef CAS PubMed.
- I. Mahmood, I. Ahmad, G. Chen and L. Huizhou, Biochem. Eng. J., 2013, 73, 72–79 CrossRef CAS.
- X. Liu, X. Chen, Y. F. Li, X. Y. Wang, X. M. Peng and W. W. Zhu, ACS Appl. Mater. Interfaces, 2012, 4, 5169–5178 CAS.
- P. Adlercreutz, Chem. Soc. Rev., 2013, 42, 6406–6436 RSC.
|
This journal is © The Royal Society of Chemistry 2016 |
Click here to see how this site uses Cookies. View our privacy policy here.