DOI:
10.1039/C6RA24455F
(Paper)
RSC Adv., 2016,
6, 114790-114799
Ultrafine Sb nanoparticles embedded in an amorphous carbon matrix for high-performance sodium ion anode materials
Received
1st October 2016
, Accepted 29th November 2016
First published on 30th November 2016
Abstract
Sodium-ion batteries (SIBs) have drawn growing interest as alternative energy-storage devices for lithium ion batteries owing to sodium's natural abundance, low cost and environmental benignity. To date, exploitation of suitable anode materials with high capacities and fast sodium ion uptake/removal for SIBs is still a great challenge. Herein, a sea-island-like SbNPs@C nanocomposite composed of Sb nanoparticles (NPs) uniformly embedded in a carbon matrix was synthesized via a facile, low cost, and high yield hydrothermal method followed by an annealing process. When used as anode materials for SIBs, the as-obtained SbNPs@C nanocomposite electrodes exhibited superior electrochemical performances (a high reversible capacity of 622 mA h g−1, 91% capacity retention over 110 cycles at 100 mA g−1 and a good rate capability of 444 mA h g−1 at 1000 mA g−1) and offered a good choice for advanced SIB anode materials.
Introduction
Commercialized lithium ion batteries (LIBs) are currently in widespread use because of their advantages such as large energy density and excellent cycling stability compared to other available energy storage devices.1,2 However, considering the limited lithium resources, the corresponding high cost is gradually becoming a problem that is exigent to be resolved.3–6 Sodium is the second lightest and smallest alkali metal7 with a great abundance in the Earth's crust (2.8%), low cost and environmental benignity; sodium ion batteries (SIBs) have attracted growing interest as an attractive candidate for LIB.8 Furthermore, the Na+/Na redox potential (−2.70 V vs. SHE) is only about 0.34 V higher than that of Li+/Li,5 and both raise the issue of seeking appropriate Na-storage anode materials with the low redox potentials needed to increase the energy density.8,9
Graphite, an anode material that is now extensively utilized for commercial LIBs,7 exhibits insignificant Na-ion storage capacities of 30–35 mA h g−1.10 Because of the ca. 40% larger radius of the Na ion compared to that of the Li ion, the number of Na ions that can reversibly and rapidly be inserted/extracted from graphite (NaC64) is considerably lower compared to Li ions (LiC6).11,12 Fortunately several other carbonaceous materials have relatively high specific capacities of ca. 300 mA h g−1, such as hard carbon,13,14 carbon nanowires,15 and hollow carbon nanospheres.16 Nevertheless, the potential of sodium inserted in a carbonaceous material is very close to the sodium deposition potential, and a severe polarization process occurs, which may lead to security issues.17,18
Recently, metallic and intermetallic anodic host materials as alternative substitutes for the graphite anode have been used to develop Na-storage anode materials, which are universally accepted as safe and high capacity Na-storage anode materials.19 Among several promising anode materials, metals that can form alloys with Na, such as Sn (Na15Sn4: 847 mA h g−1), Sb (Na3Sb: 660 mA h g−1), Pb (Na15Pb4: 484 mA h g−1) and Ge (Na3Ge: 1108 mA h g−1), have drawn considerable attention.20 These alloy-based anodes have large theoretical storage capacities and operate at more secure potentials.18 Unfortunately, the applications of these alloy electrodes are primarily hampered by their structural instabilities due to the drastic expansion/contraction during the alloying/dealloying reaction with Na, e.g., 525% for Sn and 390% for Sb,21,22 which causes structural pulverization of the anode materials, continuous depletion of the electrolyte because of the regrowth of the solid electrolyte interface (SEI) film, loss of contact with the current collector and low conductivity,6,23 resulting in a sudden capacity loss and rapid deterioration of cycling performance.5,24,25
Among the alloy anode materials mentioned above, Sb is regarded as a promising candidate for SIB anode materials because of its fast reaction kinetics,9,25,26 high theoretical capacity (660 mA h g−1), improved cycling performance and excellent rate capability when integrated with conductive carbons.4,25 Several types of Sb-based anode materials for SIBs have been studied and classified into four categories as follows: (a) pure Sb with special morphologies, such as bulk Sb,10,25 cathodically induced Sb,4 Sb porous hollow microspheres,27 monodisperse Sb nanocrystals22 and hollow Sb nanospheres;6 (b) Sb oxides (e.g., Sb2O3 nanowires,28 Sb2O4 thin film29), and Sb oxide–carbon composites (e.g., Sb2O3/Sb@graphene nanocomposites,11 SbOx/reduced graphene oxide composite30); (c) Sb-alloy compounds, such as FeSb2,31,32 Zn4Sb3,33 SnSb,19,20,34–38 Cu2Sb,9,39 Sb/Cu2Sb,40 Mo3Sb7,41 AlSb42 and Sn–Ge–Sb;43 (d) Sb/carbon-based composites including Sb/C fibers,17,44 mechanically milled Sb/C nanocomposites,19 Sb nanocrystals embedded in carbon microspheres,45 Sb nanoparticle decorated N-rich carbon nanosheets,5 wet milled Sb/MWCNT nanocomposites,46 rod-like Sb–C composite,47 pitaya-like Sb@C microspheres,48 peapod-like Sb@C submicron-structures,24 graphene/nano Sb,12 rGO/nano Sb composite,49 Sb/acetylene black composite,23 SiC–Sb–C nanocomposites18 etc. Some of the above mentioned materials exhibit good Na-storage properties; however, a considerable proportion of them suffer from several drawbacks in various respects, such as low specific capacity, poor cycling stability, high cost, and compatibility.6 Taking this into account, it is evident that carbon materials play a prominent part in improving the electrochemical performance of SIBs.12 Their Sb-based composites fully utilize the potential of the active material because of the enhancement of the electrical conductivity compared to pure Sb. Since carbonaceous materials can not only act as a buffering matrix to alleviated the large volumetric stress and separator to restrain the aggregation or coarsening of collapsed nanograins, but also serve as conductive media for electron transfer and provide more active sites for Na-storage; they bring significant improvements in higher rate capability and better cycling stability.23 Furthermore, reducing the particle size allows shorter electron and Na ionic diffusion paths and promotes good charge transportation; a facile approach is to fabricate Sb/carbon nanocomposites and nanostructured Sb as anode materials for SIBs.
Very recently, W. Luo et al. revealed a novel synthetic route for the fabrication of 1D peapod-like Sb@C structures by thermally reducing carbon-coated Sb2O3 submicron-wires composites.24 In this paper, mixed-valence antimony oxide (Sb6O13) hollow nanospheres are first fabricated via a facile hydrothermal method using sodium stibogluconate powder as a precursor. Subsequently, we imitated Luo's method and successfully obtained a sea-island-like nanocomposites consisting of Sb nanoparticles (NPs) uniformly distributed in a carbon matrix, and the size of the Sb NPs varied from 5 to 20 nm. The SbNPs@C nanocomposites displayed excellent cycle stability (540 mA h g−1 in the 145th cycle) and rate performance (443 mA h g−1 at a current density of 1000 mA g−1) for sodium insertion. Compared with previous Sb–carbons composites for SIBs, the hybrid has a lower cost and comparable electrochemical performance, indicating that it is a promising anode material for high performance SIBs.
Experimental
Preparation of Sb6O13 nanospheres (NSs)
Sb6O13NS samples were synthesized via a hydrothermal method. In a typical synthesis, 810 mg (25 mmol L−1) of sodium stibogluconate (Xiya Reagent, 99%) was dissolved into 35 mL of deionized water under vigorous agitation at room temperature. After stirring to achieve a colorless solution, dilute hydrochloric acid was added drop by drop into the above solution until the final pH of the solution was about 1.0–1.5 with stirring. A homogeneous solution formed immediately, and the total volume was controlled at 35 mL. Afterwards, the resulting colorless precursor solution was transferred into a 50 mL Teflon-lined stainless steel autoclave and maintained at 190 °C for 24 h. After being cooled to ambient temperature naturally, the dark brown Sb6O13NSs in the autoclave were collected by centrifugation, washed with deionized water followed by absolute ethanol several times and dried at 80 °C over night in air.
Pretreatment of Sb6O13NSs
Typically, 400 mg of poly(vinyl pyrrolidone) (PVP, Aladdin, Mw = 10
000 g mol−1) was first dissolved in a mixture of 0.6 mL of ethylenediamine (EDA, Guangzhou Jinhuada Chemical Co., Ltd.) and 74 mL of deionized water. Then, 608 mg of as-prepared Sb6O13NSs powder was added into the PVP/EDA/deionized water mixture under sonication for 30 min and then continuously stirred for 4 h at room temperature. Subsequently, the mixture solution was centrifuged and washed with deionized water followed by absolute ethanol repeatedly. Thus, pretreated Sb6O13NSs was collected after it was dried at 80 °C over night in air.
Synthesis of Sb6O13NSs@C
Pretreated Sb6O13NSs were coated with a layer of glucose-derived carbon via a hydrothermal reaction, which gave an intermediate product (referred to as Sb6O13NSs@C). Pretreated Sb6O13NSs (100 mg) were dispersed into an aqueous solution of glucose (35 mL, 0.01 M) under sonication and then transferred into a 50 mL Teflon-lined stainless steel autoclave and kept in an oven at 200 °C for 2 h. Sb6O13NSs@C was collected by centrifugation and washed with deionized water followed by absolute ethanol several times and dried at 80 °C over night in air.
Synthesis of SbNPs@C
SbNPs@C was prepared through a thermal reduction by converting Sb6O13NSs@C to the sea-island structure SbNPs@C nanocomposite with Sb NPs uniformly distributed in the carbon coating layer. The intermediate product (Sb6O13NSs@C) was then heated in a tube furnace at 500 °C for 2 h in H2 (30 vol%)/Ar (70 vol%) atmosphere with a heating rate of 6 °C min−1 to obtain the final product.
Materials characterization
The morphology of the Sb6O13NSs and SbNPs@C was characterized using a transmission electron microscope (TEM, FEI Tecnai G2F30) under 300 kV. Structure, composition and elemental analyses were based on X-ray powder diffraction (XRD, D/MAX 2200 VPC) with Cu Kα as the radiation source scanning at 5° min−1 from 10° to 90° (2θ), thermogravimetric analysis (TGA, NETZSCH TG209) heating from room temperature to 800 °C with a ramp rate of 10 °C min−1 in static-air conditions, energy dispersive X-ray spectrometry (EDS), scanning transmission electron microscopy (STEM) and EDS mapping.
Electrochemical measurements
The anode material was fabricated by mixing SbNPs@C, a conductive agent (Super-P, Sigma Aldrich) and sodium alginate (SA, Sigma Aldrich) in a weight ratio of 70
:
20
:
10. Then, the mixture was coated on a copper foil (d = 18 μm). The mass loading of the active materials on our electrode was about 0.25 mg cm−2, which was measured by an ultramicro analytical balance (Mettler Toledo XP2U, Switzerland, 0.1 μg resolution). After drying in air at 90 °C for 6 h, the electrodes were assembled into coin-like cells (CR2032) with a pure home-made sodium slice as both the counter electrode and the reference electrode and a glass fiber (GF/D, Whatman) as the separator in an argon (99.999%)-filled glovebox (Mikrouna Co., Ltd. Universal). The electrolyte used was 1.0 M NaClO4 in a 50
:
50 (v/v) mixture of ethylene carbonate (EC) and diethyl carbonate (DEC) solution with 5 wt% fluoroethylene carbonate (FEC) additive. The electrochemical performance of the half-cell, including cyclic voltammetry (0.01–2.50 V, 0.1 mV s−1) and electrochemical impedance spectrometry (5 mV perturbation amplitudes, 105 to 10−1 Hz) were conducted using an electrochemical workstation (Solartron 1280B). The galvanostatic charge/discharge test and the cycle stability at constant current were carried out using a multi-channel battery tester (Shenzhen Newware Technology Limited Co. China). All electrochemical tests were carried out at room temperature.
Results and discussion
In this communication, we present a facile hydrothermal carbon-coating reduction route for the fabrication of island-like SbNPs@C with Sb nanoparticles uniformly distributed in the carbon matrix. As illustrated in Scheme 1, we first synthesized Sb6O13 nanospheres (NSs) with irregular nanopores via a hydrothermal reaction using sodium stibogluconate powder as a precursor. Later, the as-prepared Sb6O13NSs powder was soaked in a mixture solution of a poly(vinyl pyrrolidone) (PVP)–ethylenediamine (EDA)–deionized water. In our experiments, PVP was present as the surface stabilizer, and EDA possibly acted as a catalyst.50 Subsequently, the pretreated Sb6O13NSs were coated with a layer of glucose-derived carbon via a hydrothermal reaction, which gave an intermediate product (referred to as Sb6O13NSs@C). There was no significant variation in the size and shape of the Sb6O13NSs, but the peripheries of the nanospheres were covered with carbon layers. Finally, Sb6O13NSs@C was reduced to a sea-island structure SbNPs@C through a hydrogen thermal reduction with Sb NPs uniformly distributed in the carbon coating layer.
 |
| Scheme 1 Schematic for the formation of a sea-island-like SbNPs@C nanocomposite by a simple hydrothermal-carbon coating-reduction route. | |
The morphology, nanoparticle size and structure features of the Sb6O13NSs were revealed by transmission electron microscope (TEM) and X-ray diffraction (XRD) tests. The typical TEM images in Fig. 1a and b show that the average size of the Sb6O13 spherical nanoparticles was ca. 20 nm with irregular nanopores. The high-resolution TEM (HRTEM) image of Sb6O13NSs, shown in the inset of Fig. 1a, exhibits a well-defined lattice spacing of 0.595 nm, corresponding to the (111) plane of cubic Sb6O13. The selected area electron diffraction (SAED, inset in Fig. 1b) pattern of Sb6O13 shows four weak diffraction rings corresponding to the (222), (400), (440) and (622) crystalline planes of the cubic Sb6O13, confirming the formation of polycrystalline cubic Sb6O13 nanocrystals. The phase and crystal structure of the Sb6O13NSs were confirmed by the XRD test. The XRD pattern shown in Fig. 1c indicates that high-purity and highly-crystalline products were obtained that could be indexed to cubic Sb6O13 (PDF#00-071-1091). As can be seen in Fig. 1c, the major diffraction peaks for Sb6O13NSs at 14.88°, 28.71°, 30.02°, 34.80°, 50.04° and 59.73° can be indexed to (111), (311), (222), (400), (440) and (622) of cubic Sb6O13.
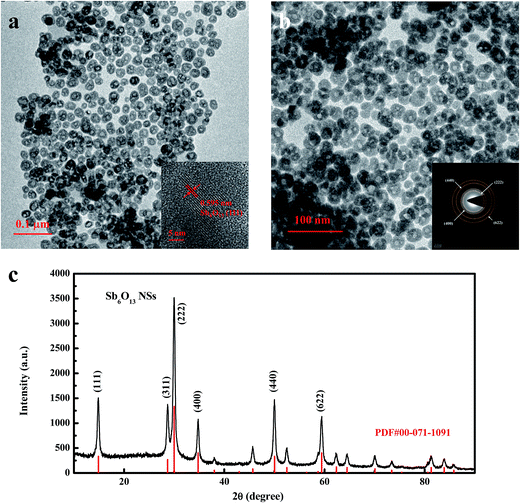 |
| Fig. 1 TEM images (a, b), HRTEM image (inset in (a)), the corresponding SAED pattern (inset in (b)) and XRD patterns (c) of the Sb6O13NSs. | |
Fig. 2a and b show the microstructures of SbNPs@C. A low-magnification TEM image in Fig. 2a reveals that Sb6O13 spherical nanoparticles with an average diameter of 20 nm have been reduced to Sb nanoparticles that homogeneously disperse in the irregular amorphous carbon matrix to obtain a “sea-island”-like structure. An HRTEM image (Fig. 2b) of the SbNPs@C composite shows the crystalline, clear lattice fringes of the Sb nanoparticles and the amorphous structure of the carbon matrix. The HRTEM image (Fig. 2c) attached to the SAED pattern (Fig. 2d) revealed the polycrystallinity of SbNPs@C. Five clear rings made up of discrete spots in the SAED pattern further confirm the presence of nanocrystalline Sb. The XRD patterns of the as-synthesized Sb6O13NSs, Sb6O13NSs@C and SbNPs@C are depicted in Fig. 2e. All of the diffraction peaks can be indexed to the standard diffraction data of Sb6O13 (PDF#00-071-1091) and Sb (PDF#00-071-1173). The as-prepared SbNPs@C with polycrystalline texture was identified and was in good agreement with the HRTEM and SAED results (Fig. 2c and d).
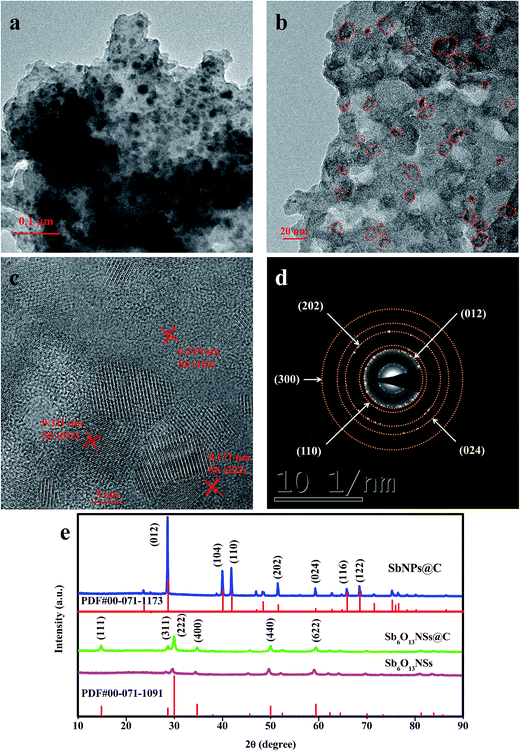 |
| Fig. 2 (a) Low-resolution TEM image of SbNPs@C; (b) high-resolution TEM image of SbNPs@C: Sb nanocrystals are marked in red regions; (c) the (110) and (012) lattice fringes; (d) the SAED pattern of the SbNPs@C: major diffraction circles are labelled with their respective hkl notation; (e) XRD patterns of the pretreated Sb6O13NSs, Sb6O13NSs@C and SbNPs@C, including the reference pattern for Sb6O13 and Sb (purple line – pretreated Sb6O13NSs, green line – Sb6O13NSs@C, blue line – SbNPs@C). | |
Furthermore, TEM, STEM and EDX mapping characterizations were conducted to investigate the microstructure of SbNPs@C. Since the reduction temperature was set to 500 °C, which is lower than the melting point of Sb (630 °C), we speculated that it will effectively prevent the aggregation of SbNPs during the annealing process. As shown in Fig. 3a and b, it can be clearly seen that the SbNPs, with particle size ranging from 5 to 20 nm, are uniformly embedded in the carbon matrix with no evident agglomeration of the Sb nanoparticles. The magnified TEM observation of the selected area marked in Fig. 3a further reveals the amorphous carbon structure of SbNPs@C. The STEM image of SbNPs@C (Fig. 3c) and corresponding EDS mapping images (Fig. 3d–f) confirm that the SbNPs are homogeneously dispersed on the carbon matrix. Moreover, an EDS measurement was performed to analyze the chemical composition of SbNPs@C (Fig. 3g). Strong Sb and C peaks confirm that the elemental contents of Sb and C were 27.3 wt% and 27.0 wt%, respectively. The signals of the Cu and O peaks originate from the carbon-coated copper grid, which is in good agreement with the mapping results mentioned above.
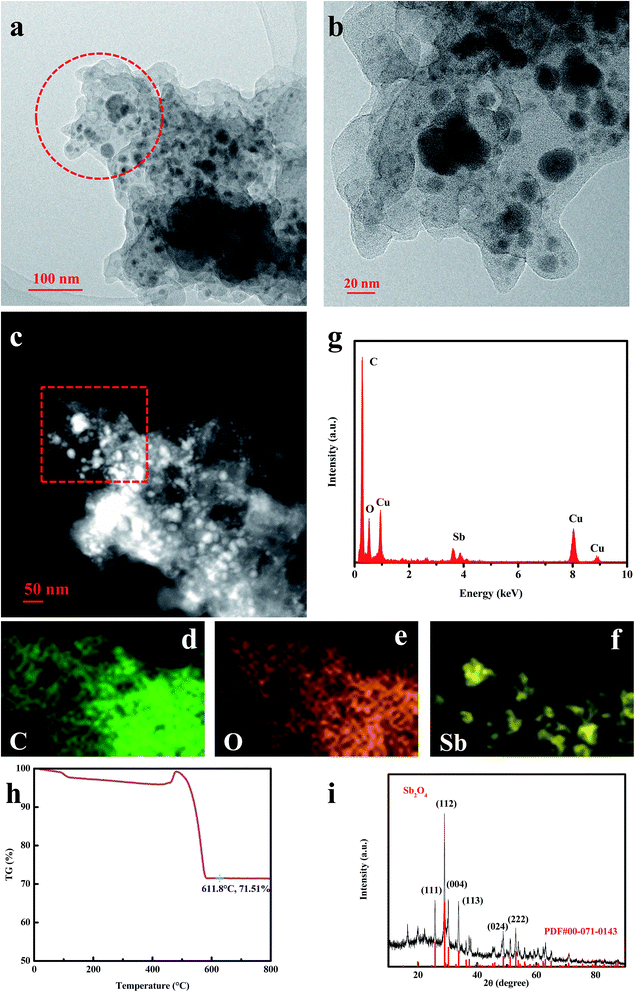 |
| Fig. 3 (a) Bright field image of SbNPs@C; (b) HRTEM image of the area marked by the red circle in (a); (c) dark field image of SbNPs@C used for EDS mapping; (d–f) the EDS elemental mapping of the area marked by the red rectangle in (c), for C (d), O (e) and Sb (f); (g) result of EDS analysis of SbNPs@C; (h) TGA curve of the SbNPs@C composite in flowing air; (i) XRD pattern of the combustion product of SbNPs@C composite. | |
The weight percentage of Sb and carbon in the SbNPs@C was measured by thermogravimetric analysis (TGA) in flowing air. The TGA curve is presented in Fig. 3h, showing a 3.4% weight loss around 100 °C, which is ascribed to adsorbed-water evaporation. Almost no weight loss was observed from 100 °C to 420 °C, demonstrating that the composite is stable up to 420 °C in air, i.e., no oxidation reaction occurs at a temperature lower than 420 °C. The weight of the SbNPs@C composite increased at 420 °C, which is attributed to the oxidation of Sb to Sb2O4, and the following weight loss from 480 °C to 580 °C was mainly due to carbon combustion. After heating to a temperature higher than 580 °C, the weight was stable with increasing temperature because all the Sb nanoparticles were oxidized and the amorphous carbon was burned off. Fig. 3i is the XRD pattern of the combustion product, and all the diffraction peaks can be indexed to the standard diffraction data of Sb2O4 (PDF#00-071-0143). Based on the TGA and XRD results, the SbNPs@C composite was calculated to be approximately 56.6 wt% Sb and 43.4 wt% carbon.
Fig. 4a shows the cyclic voltammetry (CV) curves of the initial five cycles of the SbNPs@C electrode at a scan rate of 0.1 mV s−1 over the potential window of 0.01–2.50 V (vs. Na+/Na). The pair of redox peaks observed at 0.01 and 0.14 V is due to sodium insertion/extraction into the carbonaceous materials, which was also observed in other carbon-based materials.44 In addition, a strong broad peak centered at 0.42 V was observed in the first cathodic scan (sodiation process), which is attributed to the formation of a solid electrolyte interface (SEI) layer and amorphous NaxSb alloy phase that is subsequently transformed into cubic and hexagonal Na3Sb upon further sodiation.25,26,51 The cathodic peak splits into three peaks at 0.76, 0.60 and 0.48 V in the following discharge process that corresponds to the multi-step reactions of Sb with sodium ions, resulting in a hexagonal Na3Sb alloy phase. During the anodic process (desodiation process), a long plateau at 0.77 V and a much shorter one at 0.9 V are clearly observed during the charge scans, and they are ascribed to the extraction of Na-ion from Na3Sb to form Sb. In addition, the CV curves of the following cycles are found to almost overlap, implying the excellent cycling stability of the SbNPs@C electrodes.
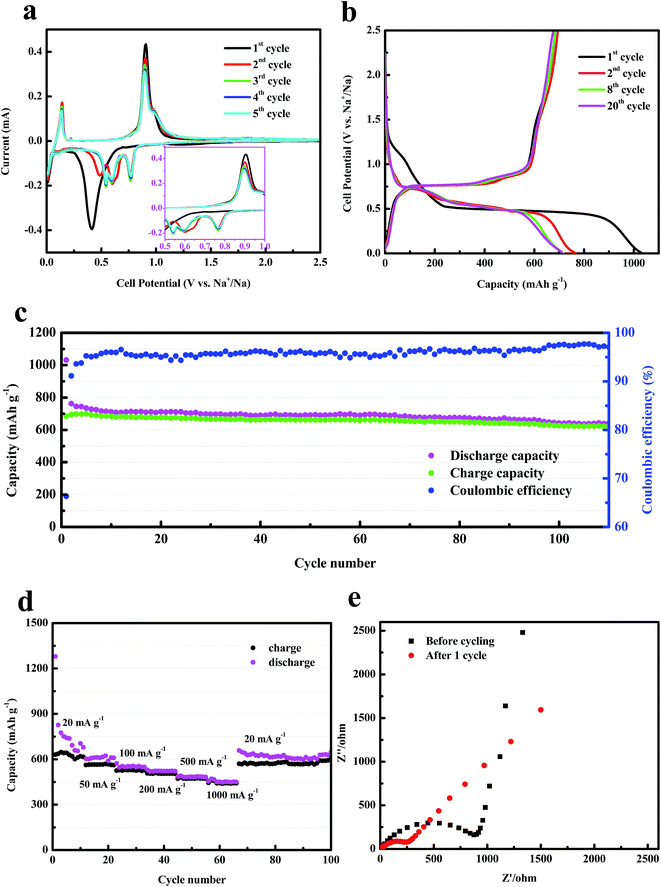 |
| Fig. 4 Electrochemical properties of the SbNPs@C electrode: (a) the initial 5 CV curves from 2.50 to 0.01 V vs. Na+/Na at a scan rate of 0.1 mV s−1; (b) galvanostatic charge/discharge voltage profiles of the 1st, 2nd, 8th, and 20th cycles between 0.01 to 2.50 V vs. Na+/Na at a current density of 100 A g−1; (c) galvanostatic cycling performance and coulombic efficiency of the SbNPs@C electrode at a current density of 0.1 A g−1; (d) rate capability of the SbNPs@C electrode at various current rates from 20 mA g−1 to 1000 mA g−1; (e) Nyquist plots of the electrochemical impedance spectra before cycling and after one cycle of SbNPs@C electrode. | |
The charge/discharge profiles of the SbNPs@C electrode obtained at a current density of 100 mA g−1 are shown in Fig. 4b. Remarkably, the plateau regions observed in the charge/discharge curves are consistent with the peaks seen in the CV curves. The initial discharge curve presents long sloping plateaus at around 0.48 V, which can be assigned to the formation of an SEI film and the conversion of hexagonal Sb to Na3Sb. The subsequent discharge curve has two sloping plateaus between 0.48 and 0.75 V, corresponding to the multi-step transformation of Sb into hexagonal Na3Sb. During the first and second charge process, sloping plateaus from 0.75 to 1.00 V were observed, which can be related to the phase transition from the Na3Sb alloy to Sb.51
The cycling performances of the SbNPs@C electrode were investigated using a current density of 100 mA g−1 with a potential range of 0.01–2.50 V vs. Na+/Na, as shown in Fig. 4c. The initial charge and discharge capacities of the SbNPs@C electrode were 1031 and 684 mA h g−1, respectively, with a coulombic efficiency (CE) of 66%. The large capacity loss of the SbNPs@C electrode is mainly attributed to the formation of the SEI layer and electrolyte decomposition during the alloying/dealloying reactions.25,26 After the initial cycle, the CE reached 95% at the fifth cycle and maintained above 96% after the tenth cycle. Consequently, a high capacity retention (ca. 91%) after 110 cycles was measured. It is worth noting that the specific capacity values were calculated based on the total mass of the SbNPs@C nanocomposite, but the content of Sb in the hybrid is merely 56.6%.
Fig. 4d exhibits the rate performance of the SbNPs@C electrode. As these current densities increase from 20 to 50, 100, 200, 500, and 1000 mA g−1, the electrodes show good capacity retention and slightly decrease from 631 to 562, 525, 507, 471, and 444 mA h g−1, respectively. Although the coulombic efficiencies of the first few cycles were below 90%, the efficiency steadily increased to 93% by the 9th cycle and continued to grow at subsequent increasing current densities. These low efficiencies were mainly attributed to consistent electrolyte decomposition, which led to the irreversible formation of the SEI film on the surface of the SbNPs electrode and carbon conductive additive during the first sodiation processes. In this study, FEC was introduced as an electrolyte additive to inhibit electrolyte decomposition and to form a stable and thin SEI layer, which can promote a longer cycle life by modifying the surface passivation layer. As reported, the coulombic inefficiencies observed in the early cycles are possibly due to: (1) the large surface area of the Sb nanoparticles, which leads to ineffectively suppressed decomposition of the electrolyte and formation of a thick SEI layer on its surface;49,52 (2) the irreversible insertion of alkali metal ions into the carbon black conductive additive;25 (3) the SbNPs@C electrode contains a small amount of oxygen (according to EDS elemental mapping in Fig. 3d and EDS analysis in Fig. 3g), and an irreversible reaction of Na+ with oxygen (e.g., Na+ + O2− → Na2O) may occur.40 Na2O is a much poorer conductor of Na ions and, therefore, may exclude some portion of the Sb material from the reversible charge/discharge;22 (4) the electronic conductivity of the glucose-derived carbon as a coating layer is low due to the low carbonization temperature used.24 Importantly, as the current density returns to 20 mA g−1, the charge capacity can recover to 570 mA h g−1. After 100 cycles, the SbNPs@C electrode delivered a capacity as high as 602 mA h g−1, indicating an excellent rate capability of SbNPs@C hybrids.
Furthermore, electrochemical impedance spectroscopy (EIS) studies were performed before cycling and after one cycle to verify the superior electrochemical performance of the SbNPs@C hybrids, and the results are displayed in Fig. 4e. As can be seen, each Nyquist plot contains a semicircle and one slope. The semicircle across the high-frequency region represents the charge-transfer impedance (Rct) on the electrode/electrolyte interface, and the slope in the low-frequency region corresponds to the Warburg impedance (W) associated with the Na+ diffusivity of the active electrode material.23 According to the diameter of these semicircles, the charge-transfer impedance (Rct) of the SbNPs@C electrode decreases significantly after one cycle, indicating that an activation step might occur during the charge–discharge process, which is in agreement with the excellent rate performance shown in Fig. 4d. Consequently, the amorphous carbon matrix accommodated the volume change of the Sb nanocrystals during the repeated alloying and dealloying processes. The structural stability of the SbNPs@C hybrids during cycling resulted in the superior electrochemical properties of the anode materials for sodium ion storage.
Conclusion
In summary, we synthesized a SbNPs@C nanocomposite using a simple hydrothermal method, followed by an annealing process. The as-prepared SbNPs@C nanocomposite displayed a uniform “sea-island” structure with the Sb nanoparticles embedded homogenously in the carbon matrix, which provided a structurally stable host for Na-ion alloying and dealloying. The SbNPs@C electrode shows an outstanding cycling stability, providing a capacity of 622 mA h g−1 after 110 cycles at a current density of 100 mA g−1. Furthermore, the SbNPs@C nanocomposite also exhibits an excellent rate-capability with a capacity of 444 mA h g−1 at a current density of 1000 mA g−1. These superior electrochemical performances indicate that the SbNPs@C composite is a promising candidate to be used as a high capacity and cycling-stable anode for SIBs.
Acknowledgements
This study was financially supported by the National Natural Science Foundation of China (No. 51125008 and No. 11274392).
References
- L. Fan, J. J. Zhang, J. H. Cui, Y. C. Zhu, J. W. Liang, L. L. Wang and Y. T. Qian, Electrochemical performance of rod-like Sb–C composite as anodes for Li-ion and Na-ion batteries, J. Mater. Chem. A, 2015, 3(7), 3276–3280 CAS.
- H. Pan, Y.-S. Hu and L. Chen, Room-temperature stationary sodium-ion batteries for large-scale electric energy storage, Energy Environ. Sci., 2013, 6(8), 2338 CAS.
- M. D. Slater, D. Kim, E. Lee and C. S. Johnson, Sodium-Ion Batteries, Adv. Funct. Mater., 2013, 23(8), 947–958 CrossRef CAS.
- Y. Yang, X. Yang, Y. Zhang, H. Hou, M. Jing, Y. Zhu, L. Fang, Q. Chen and X. Ji, Cathodically induced antimony for rechargeable Li-ion and Na-ion batteries: the influences of hexagonal and amorphous phase, J. Power Sources, 2015, 282, 358–367 CrossRef CAS.
- H. S. Hou, M. J. Jing, Y. C. Yang, Y. Zhang, Y. R. Zhu, W. X. Song, X. M. Yang and X. B. Ji, Sb porous hollow microspheres as advanced anode materials for sodium-ion batteries, J. Mater. Chem. A, 2015, 3(6), 2971–2977 CAS.
- H. Hou, M. Jing, Y. Yang, Y. Zhu, L. Fang, W. Song, C. Pan, X. Yang and X. Ji, Sodium/lithium storage behavior of antimony hollow nanospheres for rechargeable batteries, ACS Appl. Mater. Interfaces, 2014, 6(18), 16189–16196 CAS.
- M. Dahbi, N. Yabuuchi, K. Kubota, K. Tokiwa and S. Komaba, Negative electrodes for Na-ion batteries, PCCP Phys. Chem. Chem. Phys., 2014, 16(29), 15007–15028 RSC.
- Y. Kim, K. H. Ha, S. M. Oh and K. T. Lee, High-capacity anode materials for sodium-ion batteries, Chem.–Eur. J., 2014, 20(38), 11980–11992 CrossRef CAS PubMed.
- L. Baggetto, E. Allcorn, A. Manthiram and G. M. Veith, Cu2Sb thin films as anode for Na-ion batteries, Electrochem. Commun., 2013, 27, 168–171 CrossRef CAS.
- P. Ge and M. Fouletier, Electrochemical intercalation of sodium in graphite, Solid State Ionics, 1988, 28–30, 1172–1175 CrossRef.
- X. L. Zhou, Y. R. Zhong, M. Yang, M. Hu, J. P. Wei and Z. Zhou, Sb nanoparticles decorated N-rich carbon nanosheets as anode materials for sodium ion batteries with superior rate capability and long cycling stability, Chem. Commun., 2014, 50(85), 12888–12891 RSC.
- Y. Zhang, J. Xie, T. Zhu, G. Cao, X. Zhao and S. Zhang, Activation of electrochemical lithium and sodium storage of nanocrystalline antimony by anchoring on graphene via a facile in situ solvothermal route, J. Power Sources, 2014, 247, 204–212 CrossRef CAS.
- S. Komaba, W. Murata, T. Ishikawa, N. Yabuuchi, T. Ozeki, T. Nakayama, A. Ogata, K. Gotoh and K. Fujiwara, Electrochemical Na Insertion and Solid Electrolyte Interphase for Hard-Carbon Electrodes and Application to Na-Ion Batteries, Adv. Funct. Mater., 2011, 21(20), 3859–3867 CrossRef CAS.
- S. Wenzel, T. Hara, J. Janek and P. Adelhelm, Room-temperature sodium-ion batteries: improving the rate capability of carbon anode materials by templating strategies, Energy Environ. Sci., 2011, 4(9), 3342 CAS.
- Y. Cao, L. Xiao, M. L. Sushko, W. Wang, B. Schwenzer, J. Xiao, Z. Nie, L. V. Saraf, Z. Yang and J. Liu, Sodium ion insertion in hollow carbon nanowires for battery applications, Nano Lett., 2012, 12(7), 3783–3787 CrossRef CAS PubMed.
- K. Tang, L. Fu, R. J. White, L. Yu, M.-M. Titirici, M. Antonietti and J. Maier, Hollow Carbon Nanospheres with Superior Rate Capability for Sodium-Based Batteries, Adv. Energy Mater., 2012, 2(7), 873–877 CrossRef CAS.
- L. Wu, X. Hu, J. Qian, F. Pei, F. Wu, R. Mao, X. Ai, H. Yang and Y. Cao, Sb–C nanofibers with long cycle life as an anode material for high-performance sodium-ion batteries, Energy Environ. Sci., 2014, 7(1), 323–328 CAS.
- L. Wu, F. Pei, R. Mao, F. Wu, Y. Wu, J. Qian, Y. Cao, X. Ai and H. Yang, SiC–Sb–C nanocomposites as high-capacity and cycling-stable anode for sodium-ion batteries, Electrochim. Acta, 2013, 87, 41–45 CrossRef CAS.
- J. Qian, Y. Chen, L. Wu, Y. Cao, X. Ai and H. Yang, High capacity Na-storage and superior cyclability of nanocomposite Sb/C anode for Na-ion batteries, Chem. Commun., 2012, 48(56), 7070–7072 RSC.
- Y. N. Ko and Y. C. Kang, Electrochemical properties of ultrafine Sb nanocrystals embedded in carbon microspheres for use as Na-ion battery anode materials, Chem. Commun., 2014, 50(82), 12322–12324 RSC.
- L. Wu, H. Y. Lu, L. F. Xiao, X. P. Ai, H. X. Yang and Y. L. Cao, Electrochemical properties and morphological evolution of pitaya-like Sb@C microspheres as high-performance anode for sodium ion batteries, J. Mater. Chem. A, 2015, 3(10), 5708–5713 CAS.
- M. He, K. Kravchyk, M. Walter and M. V. Kovalenko, Monodisperse antimony nanocrystals for high-rate Li-ion and Na-ion battery anodes: nano versus bulk, Nano Lett., 2014, 14(3), 1255–1262 CrossRef CAS PubMed.
- H. Hou, Y. Yang, Y. Zhu, M. Jing, C. Pan, L. Fang, W. Song, X. Yang and X. Ji, An Electrochemical Study of Sb/Acetylene Black Composite as Anode for Sodium-Ion Batteries, Electrochim. Acta, 2014, 146, 328–334 CrossRef CAS.
- W. Luo, S. Lorger, B. Wang, C. Bommier and X. Ji, Facile synthesis of one-dimensional peapod-like Sb@C submicron-structures, Chem. Commun., 2014, 50(41), 5435–5437 RSC.
- A. Darwiche, C. Marino, M. T. Sougrati, B. Fraisse, L. Stievano and L. Monconduit, Better cycling performances of bulk Sb in Na-ion batteries compared to Li-ion systems: an unexpected electrochemical mechanism, J. Am. Chem. Soc., 2012, 134(51), 20805–20811 CrossRef CAS PubMed.
- L. Baggetto, P. Ganesh, C.-N. Sun, R. A. Meisner, T. A. Zawodzinski and G. M. Veith, Intrinsic thermodynamic and kinetic properties of Sb electrodes for Li-ion and Na-ion batteries: experiment and theory, J. Mater. Chem. A, 2013, 1(27), 7985 CAS.
- S. P. Ong, V. L. Chevrier, G. Hautier, A. Jain, C. Moore, S. Kim, X. H. Ma and G. Ceder, Voltage, stability and diffusion barrier differences between sodium-ion and lithium-ion intercalation materials, Energy Environ. Sci., 2011, 4(9), 3680–3688 CAS.
- K. Li, H. Liu and G. Wang, Sb2O3 Nanowires as Anode Material for Sodium-Ion Battery, Arabian J. Sci. Eng., 2014, 39(9), 6589–6593 CrossRef CAS.
- Q. Sun, Q.-Q. Ren, H. Li and Z.-W. Fu, High capacity Sb2O4 thin film electrodes for rechargeable sodium battery, Electrochem. Commun., 2011, 13(12), 1462–1464 CrossRef CAS.
- X. Zhou, X. Liu, Y. Xu, Y. Liu, Z. Dai and J. Bao, An SbOx/Reduced Graphene Oxide Composite as a High-Rate Anode Material for Sodium-Ion Batteries, J. Phys. Chem. C, 2014, 118(41), 23527–23534 CAS.
- A. Darwiche, M. Toiron, M. T. Sougrati, B. Fraisse, L. Stievano and L. Monconduit, Performance and mechanism of FeSb2 as negative electrode for Na-ion batteries, J. Power Sources, 2015, 280, 588–592 CrossRef CAS.
- L. Baggetto, H. Y. Hah, C. E. Johnson, C. A. Bridges, J. A. Johnson and G. M. Veith, The reaction mechanism of FeSb(2) as anode for sodium-ion batteries, PCCP Phys. Chem. Chem. Phys., 2014, 16(20), 9538–9545 RSC.
- E. D. Jackson, S. Green and A. L. Prieto, Electrochemical performance of electrodeposited Zn4Sb3 films for sodium-ion secondary battery anodes, ACS Appl. Mater. Interfaces, 2015, 7(14), 7447–7450 CAS.
- M. He, M. Walter, K. V. Kravchyk, R. Erni, R. Widmer and M. V. Kovalenko, Monodisperse SnSb nanocrystals for Li-ion and Na-ion battery anodes: synergy and dissonance between Sn and Sb, Nanoscale, 2015, 7(2), 455–459 RSC.
- J.-C. Kim and D.-W. Kim, Synthesis of multiphase SnSb nanoparticles-on-SnO2/Sn/C nanofibers for use in Li and Na ion battery electrodes, Electrochem. Commun., 2014, 46, 124–127 CrossRef CAS.
- L. Baggetto, H.-Y. Hah, J.-C. Jumas, C. E. Johnson, J. A. Johnson, J. K. Keum, C. A. Bridges and G. M. Veith, The reaction mechanism of SnSb and Sb thin film anodes for Na-ion batteries studied by X-ray diffraction, 119Sn and 121Sb Mössbauer spectroscopies, J. Power Sources, 2014, 267, 329–336 CrossRef CAS.
- L. Ji, M. Gu, Y. Shao, X. Li, M. H. Engelhard, B. W. Arey, W. Wang, Z. Nie, J. Xiao, C. Wang, J. G. Zhang and J. Liu, Controlling SEI formation on SnSb-porous carbon nanofibers for improved Na ion storage, Adv. Mater., 2014, 26(18), 2901–2908 CrossRef CAS PubMed.
- A. Darwiche, M. T. Sougrati, B. Fraisse, L. Stievano and L. Monconduit, Facile synthesis and long cycle life of SnSb as negative electrode material for Na-ion batteries, Electrochem. Commun., 2013, 32, 18–21 CrossRef CAS.
- L. Baggetto, K. J. Carroll, H.-Y. Hah, C. E. Johnson, D. R. Mullins, R. R. Unocic, J. A. Johnson, Y. S. Meng and G. M. Veith, Probing the Mechanism of Sodium Ion Insertion into Copper Antimony Cu2Sb Anodes, J. Phys. Chem. C, 2014, 118(15), 7856–7864 CAS.
- D.-H. Nam, K.-S. Hong, S.-J. Lim and H.-S. Kwon, Electrochemical synthesis of a three-dimensional porous Sb/Cu2Sb anode for Na-ion batteries, J. Power Sources, 2014, 247, 423–427 CrossRef CAS.
- L. Baggetto, E. Allcorn, R. R. Unocic, A. Manthiram and G. M. Veith, Mo3Sb7 as a very fast anode material for lithium-ion and sodium-ion batteries, J. Mater. Chem. A, 2013, 1(37), 11163 CAS.
- L. Baggetto, M. Marszewski, J. Górka, M. Jaroniec and G. M. Veith, AlSb thin films as negative electrodes for Li-ion and Na-ion batteries, J. Power Sources, 2013, 243, 699–705 CrossRef CAS.
- B. Farbod, K. Cui, W. P. Kalisvaart, M. Kupsta, B. Zahiri, A. Kohandehghan, E. M. Lotfabad, Z. Li, E. J. Luber and D. Mitlin, Anodes for Sodium Ion Batteries Based on Tin–Germanium–Antimony Alloys, ACS Nano, 2014, 8(5), 4415–4429 CrossRef CAS PubMed.
- Y. J. Zhu, X. G. Han, Y. H. Xu, Y. H. Liu, S. Y. Zheng, K. Xu, L. B. Hu and C. S. Wang, Electrospun Sb/C Fibers for a Stable and Fast Sodium-Ion Battery Anode, ACS Nano, 2013, 7(7), 6378–6386 CrossRef CAS PubMed.
- J. Sychev, N. V. Borisenko, G. Kaptay and K. B. Kushkhov, Intercalation of sodium and lithium into graphite as a first stage in an electrochemical method for producing carbon nanotubes, Russ. J. Electrochem., 2005, 41(9), 956–963 CrossRef CAS.
- X. Zhou, Z. Dai, J. Bao and Y.-G. Guo, Wet milled synthesis of an Sb/MWCNT nanocomposite for improved sodium storage, J. Mater. Chem. A, 2013, 1(44), 13727 CAS.
- R. Van Noorden, A better battery, Nature, 2014, 507(7490), 26–28 CrossRef CAS PubMed.
- K. S. Mohandas, N. Sanil, M. Noel and P. Rodriguez, Electrochemical intercalation of aluminium chloride in graphite in the molten sodium chloroaluminate medium, Carbon, 2003, 41(5), 927–932 CrossRef CAS.
- C. Nithya and S. Gopukumar, rGO/nano Sb composite: a high performance anode material for Na+ ion batteries and evidence for the formation of nanoribbons from the nano rGO sheet during galvanostatic cycling, J. Mater. Chem. A, 2014, 2(27), 10516 CAS.
- Z. T. Deng, F. Q. Tang, D. Chen, X. W. Meng, L. Cao and B. S. Zou, A simple solution route to single-crystalline Sb2O3 nanowires with rectangular cross sections, J. Phys. Chem. B, 2006, 110(37), 18225–18230 CrossRef CAS PubMed.
- H. S. Hou, M. J. Jing, Y. Zhang, J. Chen, Z. D. Huang and X. B. Ji, Cypress leaf-like Sb as anode material for high-performance sodium-ion batteries, J. Mater. Chem. A, 2015, 3(34), 17549–17552 CAS.
- D. H. Nam, K. S. Hong, S. J. Lim, M. J. Kim and H. S. Kwon, High-Performance Sb/Sb2O3 Anode Materials Using a Polypyrrole Nanowire Network for Na-Ion Batteries, Small, 2015, 11(24), 2885–2892 CrossRef CAS PubMed.
|
This journal is © The Royal Society of Chemistry 2016 |