DOI:
10.1039/C6RA24111E
(Paper)
RSC Adv., 2016,
6, 100344-100351
Ultrathin 2D VOPO4 nanosheets: a novel reinforcing agent in polymeric composites
Received
28th September 2016
, Accepted 17th October 2016
First published on 17th October 2016
Abstract
In the present work, ultrathin VOPO4 nanosheets were prepared through simple ultrasonication in 2-propanol, starting from bulk VOPO4·2H2O chunks. Subsequently, the ultrathin VOPO4 nanosheets were incorporated into poly(vinyl alcohol) (PVA) matrix as reinforcing agents based on a solution casting method. The ultrathin VOPO4 nanosheets were homogeneously dispersed in PVA matrix and no obvious aggregation was observed, leading to remarkable improvements of thermal stability, flame retardancy, and mechanical properties. With the incorporation of 3.0 wt% VOPO4 nanosheets into PVA, the initial decomposition temperature (Tonset)and half weight loss temperature (T50%) increased by 45 °C and 139 °C, respectively. In addition, the presence of ultrathin VOPO4 nanosheets decreases the decomposition rate of PVA and increases the amount of char residues. Furthermore, the peak heat release rate and the tensile strength of PVA films was decreased by 67% and improved by 45%, respectively, compared to that of neat PVA. These remarkably enhanced properties were mainly attributed the well dispersion, physical barrier effect and catalytic carbonization effect of the ultrathin VOPO4 nanosheets.
1. Introduction
Recently, ultrathin two-dimensional (2D) nanosheets with atomic thickness have attracted a great deal of attention due to their excellent properties and promising application.1 The ultrathin 2D nanosheets can not only enhance the intrinsic properties of their corresponding bulk samples, but also bring about new properties.2 As the most well-known layered material, graphene has attracted tremendous attention in the past ten years due to its fascinating properties.3 Accompanying the booming development of researching on graphene, extensive attentions have been focused on the inorganic graphene-like materials including boron nitrides, carbon nitrides and transition metal dichalcogenides.4 Owing to the high specific area and fascinating properties, 2D materials are promising for many applications.
As is well-known, 2D nanosheets are potential fillers for polymer nanocomposites owing to their outstanding physicochemical property and unique structure. When the 2D layered nanofillers are uniformly dispersed in the polymer matrix, the introduction of small amounts of 2D nanosheets can bring about substantial improvements in mechanical properties, thermal stability, fire resistance, and gas permeability.5 As far as we are concerned, layered nanofillers such as montmorillonite (MMT), layered double hydroxides (LDH), zirconium phosphate (α-ZrP), graphene and molybdenum disulfide (MoS2) have been proven to impart effective reinforcement and flame retardancy with polymers.6–10 Physical barrier effect of nanosheets which slows down the diffusion of gases and degradation products, and the interface interaction between nanosheets and polymer matrix, are the main reasons for these improvements.11
In recent years, much attention has been paid to the catalytic flame retardancy of polymers in the presence of acidic compounds such as MMT,12 zeolite,13 transition metal halides14 and metal phosphates.15,16 These compounds are recognized to be the catalysts for charring of polymers during their thermal degradation and combustion. Among the transition metal ions, vanadium has largely been investigated because of its redox properties which can be used to catalyze various oxidative dehydrogenations.17 Therefore, the introduction of vanadium sites on acidic saponite materials can be relevant to enhance the formation of charring products when opportunely dispersed in polymeric matrices. Sandro et al. has synthesized vanadium-containing saponite samples by one-pot synthetic procedure with the aim of preparing samples for potential application as fillers for polymeric composites. The results indicated that the V-containing solids can catalytic conversion of propene into coke and can be used as potential flame retardant filler for polymer nanocomposite materials.18 Meanwhile, phosphorus-containing compounds as efficient halogen-free flame retardants are often used alone or combined with flame retardants, which are able to increase the conversion of organic matrices to charred layers during burning. The charred layers can decrease the amount of flammable volatile gases reaching the flame zone and reduce the heat transfer from the flame to the polymer. Therefore, the application of one kind of compounds which is consist of transition metal element V and phosphate anion in the flame retardant polymeric materials is an interesting problem.
As an emerging 2D graphene-like material, dehydrated vanadyl phosphate (VOPO4) has the characteristic layered structure, in which the sheets of VOPO4 form from vertex-sharing VO6 octahedra linking to phosphate PO4 tetrahedra. Between each VOPO4 layer, one water molecule coordinates with a vanadium atom through an oxygen atom and the other links adjacent layers together through weak hydrogen bonds. The presence of weak hydrogen bonds in VOPO4 provides the feasible clue to the exfoliation of layered VOPO4 into ultrathin nanosheets while maintaining the integrity of the in-plane structure.19 Benefiting from the inherent lamellar structure, VOPO4 shows many attractive characteristics including large surface area and aspect ratio, catalytic activity, and the potential to delaminate within polymers. Similar to graphene and MoS2 nanosheets, ultrathin VOPO4 nanosheets are expected to improve the thermal stability, flame retardancy and mechanical properties of polymers. Moreover, the ultrathin VOPO4 nanosheets may catalyze dehydrogenation of polymers and promote the charring of polymers. However, the study and application of VOPO4 are still restricted in some fields. At present, the VOPO4 is mainly used as a platform for studying intercalation chemistry, the positive electrode materials for lithium batteries and provided the higher potential and active center to generate pseudocapacitance.19–21 Thus, broadening the application of VOPO4 is a promising avenue of research. To the best of our knowledge, the utilization of ultrathin VOPO4 nanosheets to fabricate polymer composite is still rare, let alone study of its thermal, flame retardant and mechanical properties.
In present work, the ultrathin VOPO4 nanosheets are incorporated into PVA matrix by solvent blending and casting method to prepare polymer nanocomposite with high performances. The thermal stability, flame retardancy and mechanical properties of the PVA nanocomposites are improved obviously, compared with those of pure PVA. It demonstrates that the ultrathin VOP nanosheets are promising and useful filler for future different polymer nanocomposite applications.
2. Experimental section
Materials
Poly(vinyl alcohol) (polymerization degree 1750 ± 50), phosphoric acid (H3PO4, 85 wt% solution), vanadium pentoxide (V2O5) and 2-propanol were purchased from Sinopharm Chemical Reagent Co., Ltd. (China). All reagents were of analytical grade and used as received without further purification.
Synthesis of bulk VOPO4·2H2O
Bulk VOPO4·2H2O chunks were prepared according to a simple method reported in previous literature.19 In a typical procedure, 9.6 g V2O5 and 26.6 mL H3PO4 solution were added into 231 g H2O and then refluxed at 110 °C for 16 h. Thereafter, the system was allowed to cool down to room temperature. The yellow precipitate was finally collected by filtration and washed several times with water and acetone, respectively. The obtained products were dried in vacuum at 60 °C for 8 h.
Preparation of PVA/VOP nanocomposite films
0.1 g bulk VOPO4·2H2O chunks were dispersed in a sealed beaker with 50 mL 2-propanol and then the dispersion was ultrasonicated in iced water for 2 h to form the VOPO4 ultrathin nanosheets which were abbreviated as VOP in following research. Neat PVA and PVA/VOP films were prepared by solution casting method, keeping the VOP loading at 1 wt% and 3 wt%. Briefly, PVA was firstly dissolved in deionized water with a concentration of 10 mg mL−1 at 90 °C in a three-neck flask. Then, the desired amount of VOP suspension was dripped into the PVA solution with vigorous stirring and maintained at 90 °C for 24 h. At last, the aqueous mixture was sonicated for 2 h and poured into Teflon Petri dishes and heated to 40 °C in oven for 24 h to form membranes. The obtained membranes were further heated at 60 °C for 6 h to remove residual water and cut into pieces for tests. Neat PVA films were prepared according to the above method except for incorporating VOP suspension.
Characterizations
X-ray diffraction was performed using a Japan Rigaku D/Max-Ra rotating-anode X-ray diffractometer equipped with a Cu Kα tube and a Ni filter (λ = 0.1542 nm) at 40 kV. FTIR spectra were obtained with a Nicolet 6700 spectrometer (Nicolet Instrument Corporation, Madison, WI, USA). SEM (JSM-6800F, Japan Electron Optics Laboratory) was used to observe the morphology of the bulk VOPO4·2H2O chunks and the fracture surface structure of PVA nanocomposites. TEM (Hitachi model H-800, JEM-2011, Japan Electron Optics Laboratory) was carried out to observe the morphology of the bulk VOPO4·2H2O chunks and ultrathin VOP nanosheets. AFM observation of ultrathin VOP nanosheets was obtained using a DI Multimode V scanning probe microscope (Veeco, Bruker Company, USA). Thermogravimetric analysis was carried out using a Q5000 thermoanalyzer instrument (TA Instruments Inc., New Castle, DE, USA) under air flow of 25 mL min−1. The temperature was increased from room temperature to 800 °C at a linear heating rate of 20 °C min−1. The thermogravimetric analysis/infrared spectrometry (TG-IR) of the samples was performed using a TGA Q5000 IR thermogravimetric analyzer that was interfaced to the Nicolet 6700 FTIR spectrophotometer. Microscale combustion colorimeter (MCC) was used to investigate the flammability characteristics of PVA and PVA nanocomposites according to ASTM D7309-07. The tensile strength and elongation at break of PVA nanocomposites were measured according to the Chinese standard of GB 13022-91 with a MTS CMT6104 universal testing machine (MTS Systems Co. Ltd, Changchun, China) at a crosshead speed of 50 mm min−1. Nanocomposite membranes were cut into special pieces, and five parallel runs were performed for each sample to obtain the averages.
3. Results and discussion
Characterization of the ultrathin VOP nanosheets
The XRD pattern of bulk VOPO4·2H2O chunks is shown in Fig. 1a. It can be seen that the bulk VOPO4·2H2O exhibits obvious diffraction peaks at 2θ = 11.9° and 24.0°, corresponding to the (001) and (002) diffraction planes with d values of 0.74 and 0.37 nm, respectively, which is a characteristic of an ordered layered structure for VOPO4·2H2O. All the diffraction peaks can be readily indexed into the tetragonal VOPO4·2H2O with the standard JCPDS card no. 84-0111 which is in good consistence with previously reported structure.22 Additionally, no peaks of impurities are detected, implying that the obtained samples are of high purity. The FTIR spectra of synthesized VOPO4·2H2O chunks is presented in Fig. 1b. The absorption peaks at 3570 and broad absorption at 3400 cm−1 are assigned to P–OH stretching vibration and OH stretching of water molecule, respectively, and the peak at 1620 cm−1 is attributed to the bending vibration of interlayer water molecules in VOPO4·2H2O chunks.23 In the low wave number region below 800 cm−1, the lattice vibration peaks are caused by V–O group.24 The presence of characteristic peaks at 1080 and 950 cm−1 are ascribe to P–O bond, suggesting the existence of PO43− group.25 These characteristic peaks are in good agreement with the chemical composition of VOPO4·2H2O. The XRD and FTIR results demonstrate that the bulk VOPO4·2H2O chunks have been successfully synthesized.
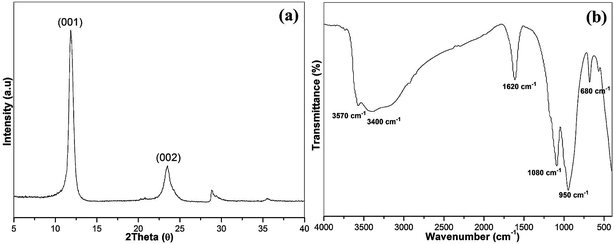 |
| Fig. 1 XRD patterns and FTIR spectra of the synthesized bulk VOPO4·2H2O chunks. | |
To illustrate the outlook and structural information of the bulk VOPO4·2H2O chunks, microscopic characterization is carried out by SEM and TEM. The SEM images of bulk VOPO4·2H2O chunks are shown in Fig. 2(a–c). It can be clearly seen that bulk VOPO4·2H2O samples show typical layered structure morphology, which is consisted of thin platelets and tightly stacked layer by layer. The TEM image in Fig. 2d also presents the platelet structure of the bulk VOPO4·2H2O samples, which is consistent with the aforementioned SEM observation. The ultrathin VOP nanosheets can be obtained by exfoliation of bulk VOPO4·2H2O chunks through a simple ultrasonication method in 2-propanol. During the process of exfoliation, strong ultrasonication stimulates the VOP layers swelled and split into thin nanosheets. TEM image of the exfoliated VOP nanosheets in Fig. 3a reveals a free-standing, sheet-like morphology, which also confirms the ultrathin nanosheet morphology of the VOP. The thickness of the ultrathin VOP nanosheets is further evaluated by tapping-mode AFM. The measured height is about 3.7 nm (Fig. 3b), denoting that the nanosheets are comprised of 5 single layers. These characterization results indicate that VOPO4·2H2O chunks are successfully exfoliated into ultrathin VOP nanosheets, which exhibit good potentials in reinforcing polymer composites.
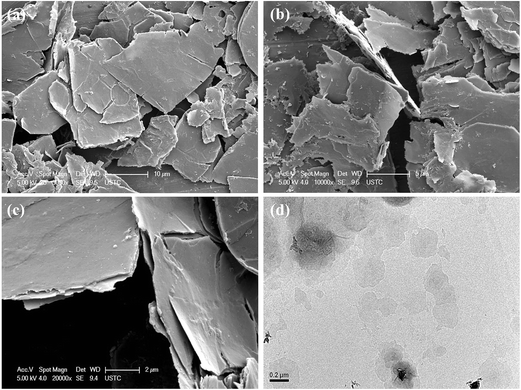 |
| Fig. 2 SEM (a–c) and TEM (d) images of the synthesized bulk VOPO4·2H2O chunks. | |
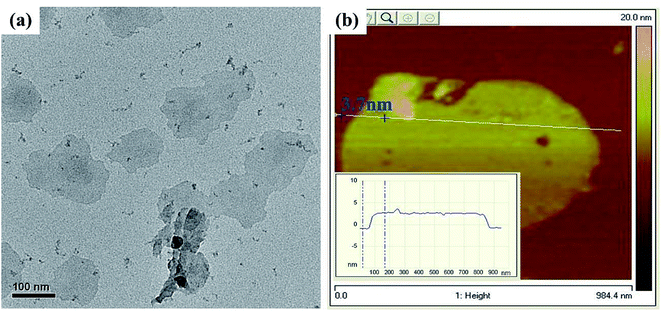 |
| Fig. 3 TEM (a) and AFM (b) images of the exfoliated ultrathin VOP nanosheets. | |
Dispersion state of the ultrathin VOP nanosheets
The dispersion state of the nanofillers and interfacial interaction between polymer matrix and nanofillers are considered to be the key factors that affect the performances of polymer composites.26 Fig. 4 shows the SEM images of the fractured surfaces for PVA composites with 1 wt% and 3 wt% ultrathin VOP nanosheets. Pure PVA represents a smooth fracture surface, which has shown in our previous work.27 In contrast, a rough fracture structure is observed on the fracture surface of PVA composites with VOP nanosheets, especially for the PVA composites with 3 wt% ultrathin VOP nanosheets, suggesting the formation of strong interfacial interaction. Moreover, the VOP nanosheets are randomly dispersed and embedded in the PVA matrix without visible aggregation, suggesting good dispersion of VOP in the matrix. The good dispersion and strong interfacial interaction are conducive to the property improvements listed in the succeeding text.
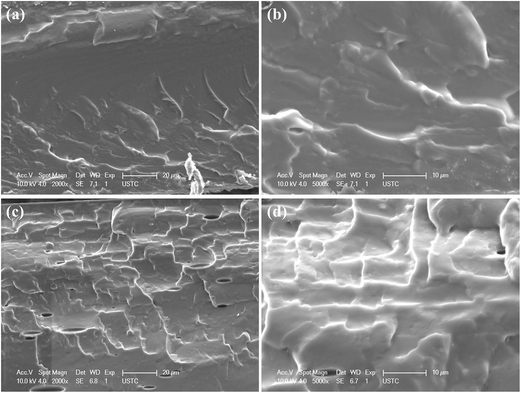 |
| Fig. 4 SEM images of the fractured sections of (a and b) PVA/1.0 wt% VOP and (c and d) PVA/3.0 wt% VOP composites. | |
Thermal degradation behavior
As an emerging 2D layered nanomaterial, the layered structure of the ultrathin VOP nanosheets can be maintained to above 400 °C,22 which makes it a promising candidate for improving the thermal property of polymers. The thermal degradation behavior of PVA and its composites is investigated by TG and TG-IR. TG-DTG profiles of neat PVA and its composites under air atmosphere are displayed in Fig. 5. As can be observed, the pure PVA follows a three-stage degradation process based on the DTG profiles corresponding to the release of absorbed water, degradation of PVA chains and the heating rearrangement of the polyalkene structure to a polyaromatic form, and dehydration of the PVA chains.28 The thermal stability of the PVA composites is improved significantly upon introducing ultrathin VOP nanosheets. When 1.0 wt% VOP nanosheets is introduced, the Tonset (the decomposition temperature on the onset stage) that is defined as the temperature with a 10 wt% mass loss is increased from 231 °C to 242 °C, and the T50% corresponding to 50 wt% weight loss is increased to 304 °C, which is 22 °C higher than that of pure PVA. As the content of VOP nanosheets further increased to 3.0 wt%, Tonset and T50% are increased by 45 °C and 139 °C, respectively, compared with those of pure PVA. Moreover, it can be obtained from DTG profiles that the maximum mass loss rate (the peak of DTG curve) of the PVA composites with ultrathin VOP nanosheets is lower than that of neat PVA, suggesting that VOP nanosheets function as an effective barrier to inhibit the mass loss during the thermal degradation process.29,32 In addition, PVA composites with VOP nanosheets have higher amount of residues than neat PVA, which could provide a protective shield of mass and heat transfer and then slow down the heat release rate during combustion. It can be clearly concluded from these results that the presence of ultrathin VOP nanosheets results in better thermal stability than neat PVA.
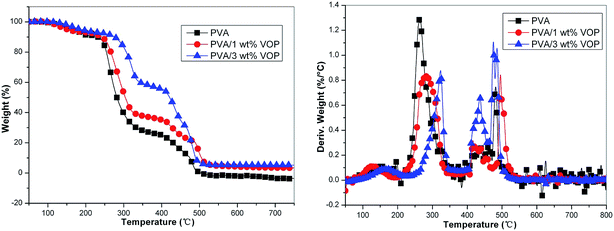 |
| Fig. 5 TG and DTG curves of PVA and its composites in air atmosphere. | |
The TG-IR technique is a useful tool in dynamical analysis as it monitors continuously both the time dependent evolution of the gases and the weight of the residue.31 To further investigate the influence of ultrathin VOP nanosheets on the evolved gaseous volatiles during pyrolysis, the volatile components of PVA and PVA/3 wt% VOP are investigated by TG-IR technique. FTIR spectra obtained at the maximum evolution rate during the thermal decomposition of PVA and its composite are presented in Fig. 6a. Some small molecular gaseous decomposition products evolved from PVA and its composites are identified unambiguously by characteristic strong FTIR signals, such as –C–H groups for allyl alcohol, ethers and hydrocarbons (3100–2800 cm−1), CO2 (2360 cm−1), CO (2180 cm−1) carbonyl compounds (1720 cm−1), and aromatic compounds (1605 and 1510 cm−1).33 The thermal degradation process of the composites is similar to pure PVA. However, the intensity of gas emission of PVA/3 wt% VOP composites is much lower than that of the neat PVA. In order to provide a clear comparison, the Gram–Schmidt (GS) curves vs. time is shown in Fig. 6b. It can be clearly observed that the absorbance intensity of total release of the decomposition products from PVA/3.0 wt% VOP is much lower than that from neat PVA. Consequently, the incorporation of VOP nanosheets into PVA can retard the thermal degradation of PVA molecular chains and reduce the release of combustible gas, resulting in a decrease in the heat release rate.34
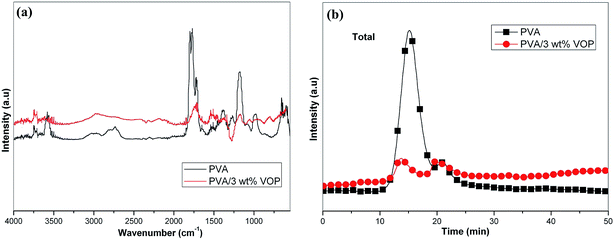 |
| Fig. 6 IR spectra of gasified pyrolysis products at the maximum evolution rate (a) and absorbance intensity of total gaseous products (b) obtained from pure PVA and PVA/3.0 wt% VOP composites. | |
Flammability
As well known, 2D layered nanofillers such as MMT, LDH, graphene and MoS2 are usually used to improve the flame retardancy of polymers. Consequently, ultrathin VOP nanosheets are expected to impart the PVA with fire resistance. In present work, the flammability of PVA and its composites is evaluated by MCC as shown in Fig. 7. The peak of heat release rate (PHRR) is one of the most important parameters to evaluate flammability, and a low value of PHRR is an indication of low flammability. Compared with neat PVA, the PVA composites achieved significant improvements in flame retardancy. The PHRR of all the PVA composites are lower than pure PVA and gradually decrease as the concentration of the VOP nanosheets increases. Meanwhile, the temperatures for PHRR are increased obviously (from 275 to 344 °C). When the concentration of VOP nanosheets reaches 3.0 wt%, the PHRR decreases apparently (from 427 W g−1 to 140 W g−1), falling by 67%, compared with that of pure PVA. The abovementioned results confirm that the incorporation of ultrathin VOP nanosheets can improve the flame retardancy of PVA significantly. According to previous literatures on polymer/2D layered nanomaterial composites, physical barrier effects of nanolayers, including mass barrier and thermal barrier effects, are hypothesized to be the main reasons for the enhanced flame retardancy.35–38 The thermal barrier effect slows down the diffusion of heat, and the mass barrier effect inhibits the emission of degradation products, resulting in the improvement of the flame retardancy. Moreover, char formation of the nanocomposites during heating, evinced by TG results, which can prevent the underlying materials from further combustion, is also a possible factor for the flame retardancy enhancement.
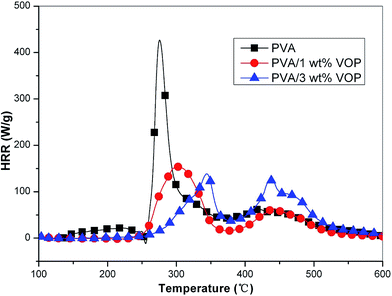 |
| Fig. 7 HRR curves of pure PVA and its composites. | |
Mechanical properties
According to previous research work, the mechanical properties of the PVA nanocomposites are improved obviously by incorporation of 2D layered nanofillers such as LDH and graphene.28,30 Based on the well dispersion of ultrathin VOP nanosheets in PVA matrix, it is speculated that the addition of ultrathin VOP nanosheets is conducive to improve the mechanical properties of the PVA composites. The typical stress–strain curves for PVA and its hybrid composites are presented in Fig. 8. The tensile strength of all the PVA hybrid films is larger than that of pure PVA and gradually increases as the content of the ultrathin VOP nanosheets increases. With loading of 3.0 wt% ultrathin VOP, the tensile strength of PVA hybrid films increases by 45%, compared with that of the pure PVA. The obvious increment of the tensile strength is mainly attributed to the well dispersion of ultrathin VOP nanosheets and strong interfacial interactions between the ultrathin VOP nanosheets and PVA matrix. The elongation at break of the hybrid films gradually decreases with the addition of ultrathin VOP nanosheets, which is mainly due to the lamellar barrier effect of the nanoflakes restricting the segmental motion of the polymer chains in the nanocomposites.31
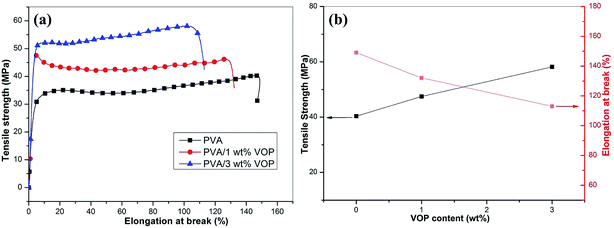 |
| Fig. 8 Typical stress–strain curves of neat PVA and its composites (a) and Tensile strength and elongation at break of neat PVA and its composites as a function of VOP loadings (b). | |
4. Conclusion
In this work, ultrathin VOP nanosheets with thickness of about 3.7 nm, as a new graphene-like material, were successfully synthesized by simple ultrasonication method, starting from bulk VOPO4·2H2O chunks. Then the VOP nanosheets were incorporated into PVA matrix as reinforcing agents based on a solution casting method. The ultrathin VOP nanosheets were homogeneously dispersed in PVA matrix and induced a significant reinforced effect on the thermal, flame retardance and mechanical properties of the PVA composites with a relatively low loading. With the VOP nanosheets volume content of 3.0 wt%, T10% and T50% was increased from 231 to 276 °C and 282 to 421 °C, respectively, compared to pure PVA. In addition, the presence of VOP nanosheets decreased the decomposition rate of PVA and increased the amount of char residues. Moreover, the TG-IR results indicated that the total pyrolysis products of PVA composites were significantly reduced. MCC results presented that the PHRR value of the PVA/3.0 wt% VOP composites was decreased by 67%, compared with pure PVA. The tensile strength of PVA hybrid films was improved by 45% upon addition of 3 wt% ultrathin VOP nanosheets. These remarkably enhanced properties were benefiting from the good dispersion, physical barrier effect and catalytic carbonization effect of ultrathin VOP nanosheets. The abovementioned results indicated that the ultrathin VOP nanosheets were effective reinforcing agents in polymer materials.
Acknowledgements
This work was supported by the Fundamental Research Funds for the Central Universities, China University of Geosciences (Wuhan) (CUG160607).
References
- J. N. Coleman, M. Lotya, A. O'Neill, S. D. Bergin, P. J. King and U. Khan, et al., Science, 2011, 331, 568–571 CrossRef CAS PubMed.
- Y. Hernandez, V. Nicolosi, M. Lotya, F. M. Blighe and Z. Sun, et al., Nat. Nanotechnol., 2008, 3, 563–568 CrossRef CAS PubMed.
- M. Liu, X. B. Yin, E. Ulin-Avila, B. S. Geng, T. Zentgraf and L. Ju, et al., Nature, 2011, 474, 64–67 CrossRef CAS PubMed.
- M. S. Xu, T. Liang, M. M. Shi and H. Z. Chen, Chem. Rev., 2013, 113, 3766–3798 CrossRef CAS PubMed.
- S. Pavlidou and C. D. Papaspyrides, Prog. Polym. Sci., 2008, 33, 1119–1198 CrossRef CAS.
- N. Bitinis, M. Hernandez, R. Verdejo, J. M. Kenny and M. A. Lopez-Manchado, Adv. Mater., 2011, 23, 5229–5236 CrossRef CAS PubMed.
- Q. Wang and O. O'Hare, Chem. Rev., 2012, 112, 4124–4155 CrossRef CAS PubMed.
- J. Alongi and A. Frache, Polym. Degrad. Stab., 2010, 95, 1928–1933 CrossRef CAS.
- K. W. Putz, O. C. Compton, M. J. Palmeri, S. T. Nguyen and L. C. Brinson, Adv. Funct. Mater., 2010, 20, 3322–3329 CrossRef CAS.
- K. Q. Zhou, S. H. Jiang, C. L. Bao, L. Song, B. B. Wang, G. Tang, Y. Hu and Z. Gui, RSC Adv., 2012, 2, 11695–11703 RSC.
- S. H. Jiang, K. Q. Zhou, Y. Q. Shi, N. N. Hong, S. M. Lo, Y. Hu and Z. Gui, Ind. Eng. Chem. Res., 2013, 52, 16766–16773 CrossRef CAS.
- P. Kiliaris and C. D. Papaspyrides, Prog. Polym. Sci., 2010, 35, 902–958 CrossRef CAS.
- M. Z. Dong, X. Y. Gu, S. Zhang, H. F. Li and P. Jiang, Ind. Eng. Chem. Res., 2013, 52, 9145–9154 CrossRef CAS.
- Y. B. Cai, Y. Hu, L. Song, S. Y. Xuan, Y. Zhang and Z. Y. Chen, et al., Polym. Degrad. Stab., 2007, 92, 490–496 CrossRef CAS.
- X. Q. Liu, D. Y. Wang, X. L. Wang, L. Chen and Y. Z. Wang, Polym. Degrad. Stab., 2013, 98, 1731–1737 CrossRef CAS.
- C. Chen, Z. H. Guo, S. Y. Ran and Z. P. Fang, Polym. Compos., 2014, 35, 539–547 CrossRef CAS.
- G. V. Isaguliants and I. P. Belomestnykh, Catal. Today, 2005, 100, 441–445 CrossRef CAS.
- O. Luca, R. Sandro, B. Chiara, C. Fabio, G. Matteo, M. Leonardo and P. Rinaldo, Chem.–Asian J., 2012, 7, 2394–2402 CrossRef PubMed.
- C. Z. Wu, X. L. Lu, L. L. Peng, K. Xu, X. Peng, J. L. Huang, G. H. Yu and Y. Xie, Nat. Commun., 2013, 4, 2431 Search PubMed.
- T. Nakato, Y. Furumi, N. Terao and T. Okuhara, J. Mater. Chem., 2000, 10, 737–743 RSC.
- B. M. Azmi, T. Ishihara, H. Nishiguchi and Y. Takita, J. Power Sources, 2003, 119, 273–277 CrossRef.
- Y. B. He, X. F. Yang, Y. L. Bai, J. Y. Zhang, L. P. Kang, Z. B. Lei and Z. H. Liu, Electrochim. Acta, 2015, 178, 312–320 CrossRef CAS.
- C. L. Bao, Y. Q. Guo, L. Song, H. D. Lu, B. H. Yuan and Y. Hu, Ind. Eng. Chem. Res., 2011, 50, 11109–11116 CrossRef CAS.
- K. Q. Zhou, Q. J. Zhang, B. Wang, J. J. Liu, P. Y. Wen, Z. Gui and Y. Hu, J. Cleaner Prod., 2014, 81, 281–289 CrossRef CAS.
- X. Y. Li, Z. Z. Wang, L. X. Wu and T. Tsai, RSC Adv., 2016, 6, 74903–74912 RSC.
- K. Q. Zhou, J. J. Liu, P. Y. Wen, Y. Hu and Z. Gui, Appl. Surf. Sci., 2014, 316, 237–244 CrossRef CAS.
- K. Q. Zhou, Z. Gui and Y. Hu, Polym. Adv. Technol., 2016 DOI:10.1002/pat.3900.
- C. L. Bao, Y. Q. Guo, L. Song and Y. Hu, J. Mater. Chem., 2011, 21, 13942–13950 RSC.
- K. Q. Zhou, J. J. Liu, Y. Q. Shi, S. H. Jiang, D. Wang, Y. Hu and Z. Gui, ACS Appl. Mater. Interfaces, 2015, 7, 6070–6081 CAS.
- S. Huang, X. Cen, H. Zhu, Z. Yang, Y. Yang, W. W. Tjiu and T. X. Liu, Mater. Chem. Phys., 2011, 130, 890–896 CrossRef CAS.
- S. D. Jiang, G. Tang and Z. M. Bai, et al., RSC Adv., 2014, 4, 3253–3262 RSC.
- K. Q. Zhou, Z. Gui and Y. Hu, RSC Adv., 2016, 6, 88707–88712 RSC.
- H. M. Zhu, J. H. Yan, X. G. Jiang, Y. E. Lai and K. F. Cen, J. Hazard. Mater., 2008, 153, 670–676 CrossRef CAS PubMed.
- N. N. Hong, Y. Pan, J. Zhan, B. B. Wang, K. Q. Zhou, L. Song and Y. Hu, RSC Adv., 2013, 3, 16440–16448 RSC.
- C. L. Bao, L. Song, C. A. Wilkie, B. H. Yuan, Y. Q. Guo, Y. Hu and X. L. Gong, J. Mater. Chem., 2012, 22, 16399–16406 RSC.
- Z. Matusinovic, R. Shukla, E. Manias, C. G. Hogshead and C. A. Wilkie, Polym. Degrad. Stab., 2012, 97, 2481–2486 CrossRef CAS.
- T. Kashiwagi, R. H. Harris, X. Zhang, R. M. Briber, B. H. Cipriano, S. R. Raghavan, W. H. Awad and J. R. Shields, Polymer, 2004, 45, 881–891 CrossRef CAS.
- C. Nyambo, P. Songtipya, E. Manias, M. M. Jimenez-Gasco and C. A. Wilkie, J. Mater. Chem., 2008, 18, 4827–4838 RSC.
|
This journal is © The Royal Society of Chemistry 2016 |