DOI:
10.1039/C6RA23595F
(Paper)
RSC Adv., 2016,
6, 111389-111397
Catalytic hydrolysis of HCN on ZSM-5 modified by Fe or Nb for HCN removal: surface species and performance
Received
22nd September 2016
, Accepted 19th October 2016
First published on 7th November 2016
Abstract
The catalytic hydrolysis of HCN was systematically investigated using Fe/ZSM-5, Nb/ZSM-5 and Fe–Nb/ZSM-5 catalysts. Fe–Nb/ZSM-5 exhibited the highest HCN hydrolysis activity and the reaction products were NH3 and CO. However, no NH3 was detected due to the large ammonia storage capacity of the catalysts. The interaction between the Fe and Nb species resulted in increased amounts of isolated Fe3+, Nb5+, oligomeric FexOy and NbxOy clusters, which could contribute to improving HCN hydrolysis. Furthermore, the excellent redox properties, favored pore structure and abundance of surface acid sites were responsible for the superior catalytic hydrolysis of HCN. Furthermore, the reaction pathway was speculated as follows: HCN and H2O reacted to produce methanamide. Methanamide further reacted with H2O to generate ammonium formate, which decomposed to formic acid and NH3. Formic acid was then converted into CO and H2O via pyrolysis.
1 Introduction
Hydrogen cyanide (HCN) emitted from the combustion of fossil fuels is regarded as an unconventional component of air pollution.1 HCN gas is 20 times more toxic than CO, and can influence human health at ppm levels in air.2 Therefore, the development of efficient catalysts to remove HCN from the atmosphere is imperative.
To date, several methods for controlling HCN have been reported, such as catalytic oxidation,3,4 adsorption5–7 and catalytic hydrolysis.1,8 Among these technologies, the adsorption method is widely used because of its flexible design and easy operation. However, this method has major drawbacks, such as HCN failing to convert or degrade, resulting in the presence of secondary pollution in the adsorption process. In order to avoid secondary pollution or toxic HCN emissions, the catalytic hydrolysis or oxidation of HCN have become attractive solutions. Zhao et al.4 reported that oxidation produced NOx (NO, NO2 and N2O), H2O, CO2 and N2 due to the strong oxidizing ability of Pt/Al2O3 catalyst during the catalytic oxidation of HCN. Gao et al.9 found that the carbon-supported catalysts showed superior catalytic activities in HCN oxidation. Furthermore, Miyadera10 declared that HCN, a selective catalytic reducing agent in the reaction with NOx, could be removed, with CuSO4/TiO2 catalyst exhibiting excellent catalytic activity. However, N2O generation impeded further development of this approach.
Regarding the catalytic hydrolysis of HCN, Kröcher et al.1 demonstrated that Fe/ZSM-5 catalyst could hydrolyze HCN to NH3, but the relationship between surface species and hydrolysis activity was not established. Marsh et al.11 also found that Al2O3 exhibited catalytic activity in the hydrolysis of HCN in the presence of H2O at 400 °C. Furthermore, zeolites are considered excellent candidates for the catalytic hydrolysis of HCN due to their ordered frameworks, favored pore diameters and large specific surface areas.1,12–14 Nanba et al.12 found that H-ferrierite could convert about 78% of HCN at 500 °C using water and oxygen. However, low-temperature hydrolysis activity should be improved. The introduction of Fe species into zeolites might improve their production rate and activity in HCN hydrolysis.13,14 Therefore, HCN removal was also studied in the hydrolysis of HCN over Fe–ZSM-5. However, the relationship between surface species and catalytic hydrolysis was not investigated thoroughly. In addition, Buckner et al.15 reported that Nb species could contribute to HCN removal. In our experiment, the HCN hydrolysis rate could be markedly improved by Nb addition. Furthermore, the effect of the interaction between the Nb and Fe species in ZSM-5 on the catalytic hydrolysis of HCN has not been reported. Therefore, ZSM-5 catalysts modified with Nb and Fe species were prepared and investigated in the catalytic hydrolysis of HCN.
In this work, the influence of the interaction between the Nb and Fe species on chemical valence, alkalinity, adsorption properties and the existing states of Nb and Fe were investigated, and the relationship between the surface species and HCN catalytic hydrolysis was studied over Fe/ZSM-5, Nb/ZSM-5 and Fe–Nb/ZSM-5 catalysts. To assess these catalysts, they were characterized using various techniques, including X-ray diffraction (XRD), UV-Vis spectroscopy, X-ray photoelectron spectroscopy (XPS), temperature-programmed reduction (TPR), N2 adsorption–desorption and temperature programmed desorption (TPD).
2 Experimental
2.1 Catalyst preparation
ZSM-5 catalysts modified with Fe and Nb species were prepared using an incipient wetness impregnation method. In a typical preparation procedure, aqueous solutions of Fe(NO3)3·9H2O or Nb(HC2O4)5·xH2O were impregnated into ZSM-5 (Si/Al = 25, Nanhua Co.) and heated at 60 °C for 5 h. Next, the catalyst was dried at 110 °C for 12 h and then calcined at 550 °C for 5 h in air. The resultant samples were denoted as Fe/ZSM-5 and Nb/ZSM-5, respectively. The Fe or Nb contents of the final catalysts were 2.5 wt%. In addition, using the same process as for Fe/ZSM-5, Fe(NO3)3·9H2O and Nb(HC2O4)5·xH2O aqueous solutions were simultaneously impregnated into ZSM-5, with the resultant catalyst denoted as Fe–Nb/ZSM-5.
2.2 Catalytic activity measurement
Catalytic hydrolysis measurements were performed in a fixed-bed quartz flow reactor (6 mm i.d.) with 20 mg of catalyst. Reactant gases were measured using mass-flow controllers before entering the reactor. The concentrations of the simulated gases were as follows: 80 ppm HCN, 0.25 vol% water vapor and balance Ar, with a total flow rate of 50 mL min−1. Water vapor was generated by passing Ar flow through a gas-wash device containing deionized water. HCN concentration was analyzed using a mass spectrometer (MAX300-LG). NH3 production was determined quantitatively using an NH3 detecting pipe. NO and NO2 concentrations were measured using a ECOM J2KN flue gas analyzer. N2O and CO were detected by gas chromatography (Fuli, 9790). The reaction system was maintained for 30 min at each reaction temperature before analysis.
To avoid adsorption affecting the catalytic hydrolysis of HCN over the catalysts, each sample was subjected to a flow of 80 ppm HCN/Ar at a rate of 50 mL min−1 for 60 min at 150 °C. After saturation, the catalysts were purged in pure Ar flow for 30 min at 150 °C. Finally, 0.25 vol% water vapor was introduced. After catalysis, carbon balances were employed in the hydrolysis process to offer additional information about non-measured by-products, such as formic acid and methanamide.1 These C-balances were good for evaluating measurement quality in the catalytic hydrolysis of HCN.
N-balances played an important role in the catalytic hydrolysis of HCN, with the N-containing reaction products comprising only NH3, except in the case of strong oxidation catalysts, which completely oxidized HCN to N2O or NOx (NO2 and NO).
2.3 Catalyst characterization
Powder X-ray diffraction (XRD) measurements were collected on a Rigaku D/Max 2500 system with Cu radiation (λ = 0.15418 nm). The current and tube voltage were 100 mA and 40 kV, respectively. The X-ray powder diffractogram was recorded at 0.03° intervals in the 2θ range of 10–70° to determine the crystal structure. Crystalline phases were identified by comparison with the reference data from International Center for Diffraction Data (ICDD) files.
Microstructures of the samples were obtained using scanning electron microscopy (SEM) on a VEGA3 TEWCAN system at a voltage of 30 kV.
N2 adsorption–desorption isotherms were acquired at −196 °C using a Tristar II 3020 automated gas sorption system. Before N2 adsorption, the catalyst was degassed at 300 °C for 4 h. Adsorption–desorption was achieved by passing nitrogen over the catalysts, which were kept under liquid nitrogen. The specific surface areas of the catalysts were calculated from these isotherms using the Brunauer–Emmett–Teller (BET) model. Pore size distributions were measured from the adsorption branch of the isotherms using the Barrett–Joyner–Halenda (BJH) method.
UV-Vis spectra of the samples were obtained using a Varian-Cary 5000 UV-Vis spectrometer with a matched pair of silica cuvettes with a 1 cm optical path length. Spectra were recorded in the range 210–800 nm.
X-ray photoelectron spectroscopy (XPS) was carried out using ULVAC PHI 5000 Versa Probe-II equipment operating at 10−9 Pa with Al Kα radiation (1486.6 eV) to study the chemical states of the samples. Catalyst charging was compensated for by an electron flood gun during measurements. With respect to the sample surface, the electron take-off angle was 45°. The spectra were measured in fixed-analyzer transmission mode. The samples were put into a sample holder and evacuated for 12 h at 25 °C. The observed spectra were corrected using the C 1s binding energy (284.8 eV).
H2 temperature-programmed reduction was performed in a conventional gas chromatograph (Fuli GC-9750) equipped with a thermal conductivity detector. H2-TPR was operated using 5% hydrogen in nitrogen as the reducing gas. Prior to the H2-TPR experiments, a 20 mg sample was pre-treated at 400 °C for 45 min under a N2 flow at a rate of 30 mL min−1 and then cooled to 200 °C. The samples were then subjected to flowing H2 (5% in N2, 30 mL min−1) and heated from 200 to 900 °C at a rate of 10 °C min−1.
NH3/CO2 temperature-programmed desorption (NH3/CO2-TPD) experiments were performed using a conventional gas chromatograph (Fuli GC-9790) equipped with a thermal conductivity detector on 20 mg samples. Prior to experiments, the samples were pretreated with pure He at 400 °C for 40 min and cooled to 100 °C under a He flow at a rate of 30 mL min−1, followed by saturation for 60 min under a stream of NH3 (4%) or CO2 (5%) in He. After saturation, the samples were flushed with pure He flow for 30 min at 100 °C. TPD experiments were operated in pure He from 100 to 800 °C at a heating rate of 10 °C min−1.
3 Results and discussions
3.1 XPS analysis
To investigate the surface species and chemical states of the elements in Fe/ZSM-5, Nb/ZSM-5 and Fe–Nb/ZSM-5, the samples underwent XPS, with the results shown in Fig. 1. Fig. 1a shows the XPS results for Fe 2p in Fe/ZSM-5 and Fe–Nb/ZSM-5. The Fe 2p3/2 peak centered at 711–711.2 eV was assigned to Fe2+, while the Fe 2p3/2 peak at 713.5–713.7 eV was attributed to Fe3+.16,17 This phenomenon indicated that both Fe2+ and Fe3+ species existed in the Fe/ZSM-5 and Fe–Nb/ZSM-5 catalysts. Furthermore, the relative amounts of Fe3+ and Fe2+ were measured using the sum of the divided peak area of Fe2+ and Fe3+ over the Fe 2p3/2 peak, with the results shown in Fig. 1a. The Fe3+ concentration was 72% in the Fe/ZSM-5 catalyst, and the value increased with the simultaneous introduction of Fe and Nb species over Fe–Nb/ZSM-5. Fe–Nb/ZSM-5 contained a higher concentration of Fe3+ (78%). In addition, the binding energies of the Fe2+ and Fe3+ species in Fe–Nb/ZSM-5 were lower than that of Fe/ZSM-5, which demonstrated that interactions caused electron redistribution between the Fe and Nb species in the catalysts.
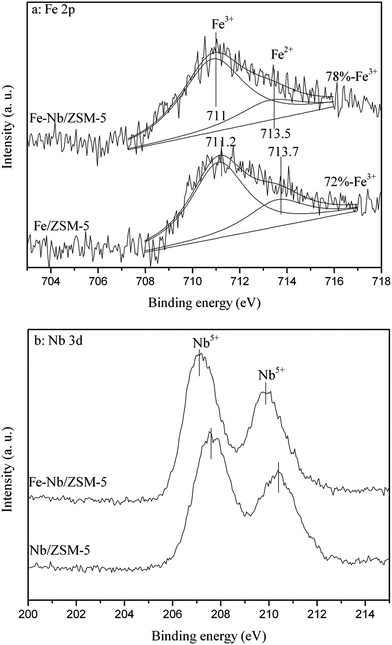 |
| Fig. 1 XPS spectra of the catalysts: (a) Fe 2p and (b) Nb 3d. | |
Fig. 1b shows binding energies in the Nb 3d spectra. Nb 3d peaks centered at 207.1 and 209.7 eV, assigned to the Nb5+ oxidation state, were reported previously.18,19 A visible shift in the Nb/ZSM-5 and Fe–Nb/ZSM-5 catalysts was observed in comparison with the above positions, demonstrating that the oxidation state of the Nb species was +5. Furthermore, the position of Nb in Fe–Nb/ZSM-5 shifted to lower values compared with that of Nb/ZSM-5. This proved that the addition of Fe species into Nb/ZSM-5 could inhibit interaction between Nb and the support (ZSM-5). Additionally, an extra-strong interaction would lead to the presence of agglomerated particles. Therefore, the simultaneous introduction of Fe and Nb species onto ZSM-5 could contribute to excellent dispersity.
3.2 UV-Vis
UV-Vis spectra results are displayed in Fig. 2. For Fe/ZSM-5, the spectrum shows a broad band at 210–800 nm. Bands below 300 nm were previously attributed to isolated Fe3+ or Nb5+ species, while bands above 400 nm corresponded to large Fe2O3 or Nb2O5 particles on the external catalyst surface, and bands between 300 and 400 nm were assigned to oligomeric FexOy or NbxOy clusters.20–22 Therefore, isolated Fe3+, oligomeric FexOy clusters and large Fe2O3 particles were formed in Fe/ZSM-5. As for Nb/ZSM-5, the band below 300 nm, corresponding to isolated Nb5+ species, appeared in the framework zeolite, while a UV-Vis band at 300–375 nm, due to extra-framework oligomeric NbxOy clusters, was also observed. Moreover, the absence of a peak at 600–800 nm demonstrated that reduced Nb4+ species were not present. For Fe–Nb/ZSM-5, the peak strengths in the range 210–376 nm were higher than those of Fe/ZSM-5 and Nb/ZSM-5, and were assigned to isolated Fe3+, Nb5+, oligomeric FexOy and NbxOy clusters. Hence, the UV-Vis results implied that two kinds of Fe and Nb species were present in Fe/ZSM-5 and Nb/ZSM-5, namely, Fe3+ or Nb5+ ions in the framework, and agglomerated extra-framework Fe or Nb species resulting from large particle sizes. Furthermore, the simultaneous introduction of Fe and Nb species could change the form of active sites (Fe and Nb), resulting in an increase in the amount of metal (Fe3+ and Nb5+) ions and oligomeric clusters (FexOy or NbxOy) because of the higher intensity at 210–400 cm−1 for Fe–Nb/ZSM-5. These XPS results implied that the interaction between the Fe and Nb species was responsible for the formation of Fe and Nb species.
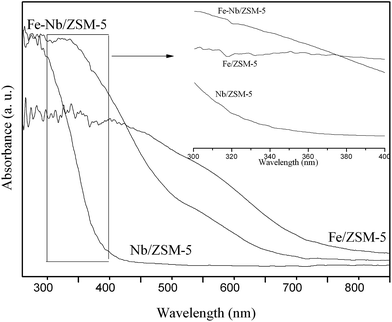 |
| Fig. 2 UV-Vis spectra of Fe/ZSM-5, Nb/ZSM-5 and Fe–Nb/ZSM-5. | |
3.3 XRD analysis
The XRD patterns of the samples are shown in Fig. 3. All samples had characteristic peaks and showed reasonably high crystallinities, which were assigned to typical ZSM-5 structural patterns. These observations implied that the introduction of Fe and Nb species into the ZSM-5 catalysts had not destroyed the original zeolite structure. Furthermore, no diffraction peaks were observed, proving that all Fe and Nb species were highly dispersed or existed as isolated ions in the catalysts. Although the crystal structure of the ZSM-5 catalyst was not significantly affected by the addition of Fe and Nb species, the positions of the diffraction peaks might be influenced by the interactions among Fe, Nb and ZSM-5. The peak positions of Fe/ZSM-5 and Nb/ZSM-5 showed no obvious change. However, the Fe–Nb/ZSM-5 peak shifted to a lower value, implying that lattice expansion had occurred. This phenomenon confirmed the incorporation of Fe and Nb ions inside the pores of ZSM-5,23 which was in good agreement with the UV-Vis results. Based on these peaks (about 2θ = 25.8°), the catalyst crystallites was measured using the Sherrer's equation, giving average sizes of 5.2, 4.9 and 5.1 nm for Fe/ZSM-5, Nb/ZSM-5 and Fe–Nb/ZSM-5. Furthermore, when Fe and Nb species were simultaneously doped into ZSM-5, the Nb/ZSM-5 particle sizes did not increase compared with those of Fe/ZSM-5 and Nb/ZSM-5, implying that the Fe and Nb species were highly dispersed on ZSM-5.
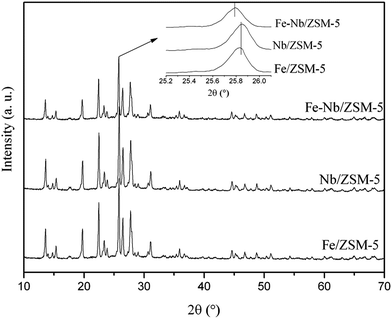 |
| Fig. 3 XRD patterns of Fe/ZSM-5, Nb/ZSM-5 and Fe–Nb/ZSM-5. | |
3.4 SEM analysis
The catalysts were investigated by SEM, with the results shown in Fig. 4. Fe/ZSM-5 presented approximate spheres and uneven large particles, while Nb/ZSM-5 exhibited a slight aggregation of uneven rod or bulk particles, and Fe–Nb/ZSM-5 showed spherical particles with snowflake morphologies. The morphologies of the Fe–Nb/ZSM-5 catalysts were dramatically affected by the simultaneously introduction of the Fe and Nb species, which might be related to the interaction between Fe and Nb species in Fe–Nb/ZSM-5.
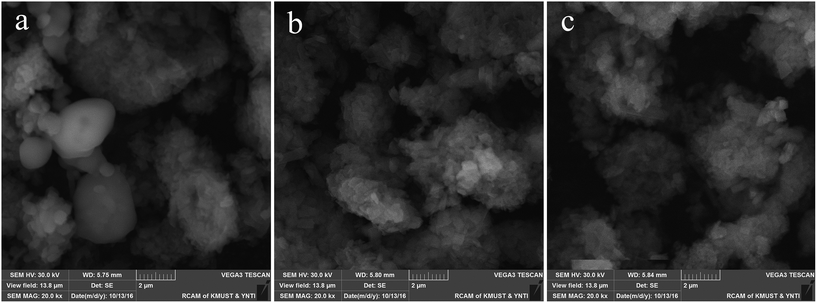 |
| Fig. 4 SEM of Fe/ZSM-5 (a), Nb/ZSM-5 (b) and Fe–Nb/ZSM-5 (c). | |
3.5 N2 adsorption–desorption
The N2 adsorption–desorption isotherms and pore size distributions of Fe/ZSM-5, Nb/ZSM-5 and Fe–Nb/ZSM-5 are shown in Fig. 5. The isotherms of the catalysts at low P/P0 corresponded to type IV isotherms, according to the BDDT classification, which is typically related to capillary condensation in mesopores (2–50 nm).24 The isotherms of Fe/ZSM-5, Nb/ZSM-5 and Fe–Nb/ZSM-5 showed no obvious changes, indicating that the ZSM-5 pore structure was not significantly affected by the introduction of Fe and Nb species. This result was in good agreement with the XRD results. Fig. 5b shows the BJH pore size distributions of the catalysts. The main pore sizes were in the range 2–28 nm, which were ascribed to mesopores. The distribution peaks of the samples were ∼4.1 nm, whereas the peak intensities of Fe/ZSM-5 and Fe–Nb/ZSM-5 were weaker than that of Nb/ZSM-5. This phenomenon showed that Nb/ZSM-5 possessed more abundant mesopores, which contributed to the pore structure.
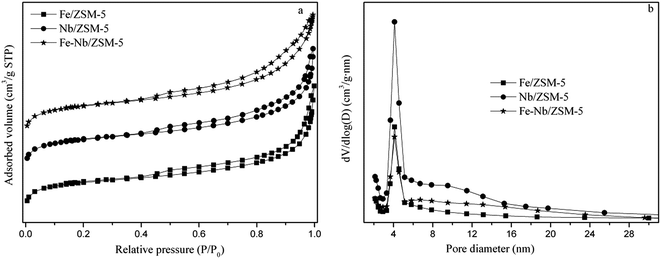 |
| Fig. 5 N2 adsorption–desorption results for the catalysts: (a) N2 adsorption–desorption isotherms; (b) BJH pore size distributions. | |
The textural parameters of the Fe/ZSM-5, Nb/ZSM-5 and Fe–Nb/ZSM-5 catalysts are depicted in Table 1. The BET surface areas of Fe/ZSM-5, Nb/ZSM-5 and Fe–Nb/ZSM-5 were 395, 396 and 381 m2 g−1, with total pore volumes of 0.098, 0.096 and 0.107 cm3 g−1, respectively. The pore structure of Fe–Nb/ZSM-5 was almost unchanged, and even slightly increased compared with those of Fe/ZSM-5 and Nb/ZSM-5, indicating that the interaction existing between the Fe and Nb species was conducive to the excellent dispersity of Fe and Nb species in Fe–Nb/ZSM-5, resulting in the excellent pore structure.
Table 1 BET analysis results of various catalysts
Sample |
BET (m2 g−1) |
Total pore volume (cm3 g−1) |
Average pore diameter (nm) |
Fe/ZSM-5 |
395 |
0.098 |
10.7 |
Nb/ZSM-5 |
396 |
0.096 |
9.7 |
Fe–Nb/ZSM-5 |
381 |
0.107 |
9.4 |
3.6 H2-TPR analysis
Fig. 6 shows the H2-TPR profiles of the Fe/ZSM-5, Nb/ZSM-5 and Fe–Nb/ZSM-5 catalysts. The Nb2O5 reduction peak was reportedly above 850 °C and, thus, should not be detected for Nb/ZSM-5 in the H2-TPR profiles. However, a broad weak reduction peak appeared at 200–700 °C for Nb/ZSM-5, which might be due to the reduction of isolated Nb5+ species or extra-framework oligomeric NbxOy clusters.25 A broad peak centered of Fe/ZSM-5 at 412 °C corresponded with the reduction of Fe3+ ions and oligomeric FexOy clusters species, and the high reduction peak at 594 °C was assigned to the reduction of large Fe2O3 particles.26,27 The reduction peak at 200–550 °C in Fe–Nb/ZSM-5, which was assigned to the mutually overlapping reduction of the Fe and Nb species, appeared at a lower reduction temperature than those of Fe/ZSM-5 and Nb/ZSM-5, resulting from the interaction between the Fe and Nb species. Furthermore, Fe2O3 reduction was absent in Fe–Nb/ZSM-5, which might be attributed to the interaction between the Fe and Nb species, which caused improved Fe and Nb dispersities. This also demonstrated that the redox ability was dramatically improved by the simultaneous introduction of Fe and Nb species. It was reasonable to conclude that the synergetic effect was beneficial to electron transfer, resulting in the catalysts possessing excellent redox abilities. In addition, the higher redox abilities of the catalysts contributed to the reaction because of the synergetic effect between the Fe and Nb species.
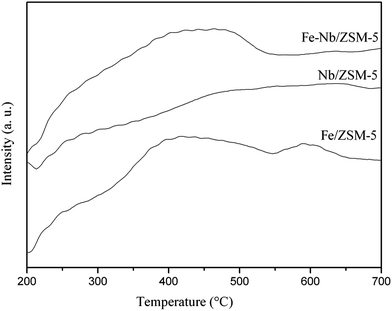 |
| Fig. 6 H2-TPR profiles of Fe/ZSM-5, Nb/ZSM-5 and Fe–Nb/ZSM-5 catalysts. | |
3.7 CO2-TPD analysis
HCN is an acidic gas, and so the surface basicity of the catalyst could affect the adsorption and activation capacities for HCN. Therefore, CO2-TPD analyses of Fe/ZSM-5, Nb/ZSM-5 and Fe–Nb/ZSM-5 were performed. As shown in Fig. 7, a large CO2 desorption peak, centered at 216 °C, was observed for Fe/ZSM-5, while the CO2-desorbed peak of Nb/ZSM-5 and Fe–Nb/ZSM-5 was located at 100–400 °C. Furthermore, the peak intensities were in the following order: Fe/ZSM-5 > Nb/ZSM-5 > Fe–Nb/ZSM-5. These results demonstrated that Fe/ZSM-5 possessed the largest amount of basic acid sites and that the simultaneous introduction of Fe and Nb species into ZSM-5 impeded the generation of surface basic acid sites.
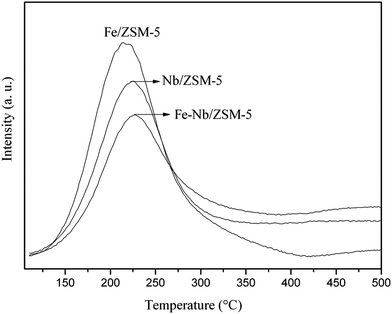 |
| Fig. 7 CO2-TPD results of Fe/ZSM-5, Nb/ZSM-5 and Fe–Nb/ZSM-5 catalysts. | |
3.8 Catalytic activity
The catalytic hydrolysis of HCN in the presence of 0.25 vol% H2O over Fe/ZSM-5, Nb/ZSM-5 and Fe–Nb/ZSM-5 is shown in Fig. 8, and the reaction products, CO and NH3, are listed in Table 2. As shown in Fig. 8, the Fe/ZSM-5 catalyst achieved 26% HCN conversion at 200 °C and 80% HCN conversion at 243–300 °C. The Nb/ZSM-5 catalyst gave lower catalytic hydrolysis activity compared with Fe/ZSM-5. Nb/ZSM-5 achieved 23% HCN conversion at 200 °C and over 80% HCN conversion at 248 °C. Using the Fe–Nb/ZSM-5 catalyst, improved HCN hydrolysis and 80% HCN conversion were attained in the temperature range 231–300 °C. Therefore, this activity in the catalytic hydrolysis of HCN was clearly due to the simultaneous introduction of Fe and Nb species into ZSM-5.
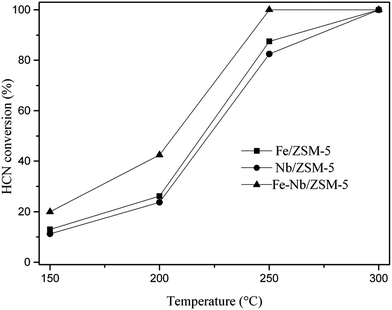 |
| Fig. 8 Catalytic hydrolysis of HCN over Fe/ZSM-5, Nb/ZSM-5 and Fe–Nb/ZSM-5. | |
Table 2 Hydrolysis products, CO and NH3 (both ppm), from the catalytic hydrolysis of HCN over Fe/ZSM-5, Nb/ZSM-5 and Fe–Nb/ZSM-5
Temperature (°C) |
Fe/ZSM-5 |
Nb/ZSM-5 |
Fe–Nb/ZSM-5 |
CO |
NH3 |
CO |
NH3 |
CO |
NH3 |
150 |
8 |
0 |
8 |
0 |
15 |
0 |
200 |
20 |
0 |
19 |
0 |
33 |
0 |
250 |
70 |
0 |
65 |
0 |
78 |
0 |
300 |
78 |
0 |
79 |
0 |
78 |
0 |
As shown in Table 2, HCN was almost quantitatively hydrolyzed to CO using Fe/ZSM-5, Nb/ZSM-5 and Fe–Nb/ZSM-5 catalysts. However, the typical product of this hydrolysis reaction, NH3, was not detected. According to N-balances, it was inferred that NH3 could be oxidized to N2, NO2, N2O and NO. Therefore, NOx (NO and NO2) and N2O were measured, but these typical oxidation products were not detected in the samples. Based on our previous experiments, Fe/ZSM-5 showed almost no activity in the catalytic oxidation of ammonia at low reaction temperatures (below 250 °C). Hence, NH3 was likely to be adsorbed to the catalysts with an equilibrium time of more than 30 min, resulting in no NH3 being observed during HCN hydrolysis. In order to verify our prediction, NH3-TPD was performed over Fe/ZSM-5, Nb/ZSM-5 and Fe–Nb/ZSM-5, and the results are shown in Fig. 9. All samples exhibited two NH3-desorbed peaks: the first in the temperature range 110–350 °C, which was attributed to weak and medium acid sites that could adsorb NH3; and the second centered at 530 °C, corresponding to NH3 adsorbed to strong acid sites. Furthermore, the strong acid sites were related to the released adsorbed NH3 under high temperature treatment. In addition, the strengths of the NH3-desorption peaks for Fe/ZSM-5, Nb/ZSM-5 and Fe–Nb/ZSM-5 were quite high, indicating that the three samples possessed excellent NH3 adsorption capacities, resulting in the absence of NH3 from the catalytic hydrolysis of HCN over Fe/ZSM-5, Nb/ZSM-5 and Fe–Nb/ZSM-5.
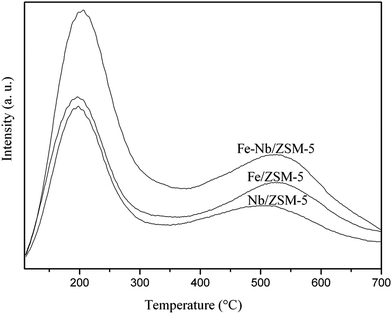 |
| Fig. 9 NH3-TPD results of Fe/ZSM-5, Nb/ZSM-5 and Fe–Nb/ZSM-5 catalysts. | |
3.9 Discussion
3.9.1 Effects of structures and surface species on HCN hydrolysis activity. This study focused on the effects of chemical valence, alkalinity, adsorption properties and existing Nb and Fe states on hydrolysis activity over the ZSM-5 catalysts modified with Fe or Nb species. Kharas et al.28 found that the generation of metal oxide crystallites was too large to deactivate the catalyst. The XRD results showed excellent dispersion of the Fe and Nb species in the ZSM-5 catalysts. Combined with the UV-Vis, BET and XPS results, this confirmed that a strong interaction between ZSM-5 and Nb species occurred in Nb/ZSM-5. However, Fe addition led to an interaction between Fe and Nb species, which decreased the forces between ZSM-5 and Nb. For Fe/ZSM-5, the strength of band at 400–700 nm was much higher than that of Fe–Nb/ZSM-5, which indicated that increased amounts of large Fe2O3 particles formed over Fe/ZSM-5. The generation of metal oxide crystallites has been reported to deactivate the catalyst owing to their large sizes.28 When the Nb species was added, the peak intensity of Fe–Nb/ZSM-5 at 210–375 °C was markedly increased in comparison with those of Fe/ZSM-5 and Nb/ZSM-5, implying that Fe–Nb/ZSM-5 possessed greater quantities of isolated Fe3+, Nb5+, oligomeric FexOy and NbxOy clusters. Hence, Fe–Nb/ZSM-5 possessed a large BET specific area and high total pore volume, which favored pore diffusion of the reactant gas, and contributed to improving the hydrolysis activity. Furthermore, the introduction of Nb contributed to Fe3+ formation. The catalytic hydrolysis of HCN to CO and NH3 took place over Fe3+ sites.1,29 Therefore, increased Fe3+ concentrations resulted in the generation of more active sites, causing hydrolysis activity to be promoted. Fe–Nb/ZSM-5 possessed the highest surface concentration of Fe3+ (78%). Therefore, Fe–Nb/ZSM-5 should have the best activity in the catalytic hydrolysis of HCN, which was in good agreement with catalytic hydrolysis activity observed. The H-ZSM-5 catalyst has been reported12 to show quite poor activity below 300 °C in the hydrolysis reaction. However, the Nb/ZSM-5 catalyst showed the excellent activity at 150–300 °C, suggesting that Nb5+ species were active sites for the catalytic hydrolysis of HCN. The UV-Vis results indicated that more Nb5+ and oligomeric Nb2O5 clusters were observed in Fe–Nb/ZSM-5, which might be another reason for its superior hydrolysis activity. In brief, the differences discussed above were derived from the interaction between the Fe and Nb species in the ZSM-5 catalysts.Redox properties played an important role in the catalytic hydrolysis of HCN. Zhang et al.30 showed that catalytic activity was dramatically affected by the dispersion of metal species over supported metal catalysts. Kim et al.31 also demonstrated that excellent dispersion of the active species on the support contributed to outstanding catalytic performance. Consequently, well-dispersed Fe and Nb species were important factors in the superior catalytic hydrolysis of HCN. Furthermore, lower reduction temperatures and higher reduction peak intensities were observed for Fe–Nb/ZSM-5. In correlation with the XRD, XPS and UV-Vis results, the interaction between the Fe and Nb species was beneficial to the generation of abundant Fe3+, Nb5+, oligomeric FexOy and NbxOy clusters, and contributed to the good dispersion of Fe and Nb species, resulting in the excellent redox properties of Fe–Nb/ZSM-5. This was another potential reason for the superior catalytic hydrolysis of HCN over Fe–Nb/ZSM-5.
Acidic HCN is widely accepted to be prone to adsorption to basic acid sites, and so the reaction product, NH3, was more easily desorbed. Therefore, surface basicity contributed to the enhancement of catalytic hydrolysis of HCN. However, the Fe–Nb/ZSM-5 catalyst with a lower concentration of basic sites exhibited better hydrolysis activity than Fe/ZSM-5 and Nb/ZSM-5, especially at low temperature catalytic activity, which seemed contradictory to the above theory. MoO3–TiO2, possessing an acidic surface due to the addition of MoO3, was active as a HCN hydrolysis catalyst.1 Therefore, both surface basicity and acidity contribute to the catalytic hydrolysis of HCN, but NH3, the hydrolysis reaction product, bound to acid sites was usually not detected due to the large NH3 storage capacity. According to the CO2-TPD and NH3-TPD results, the introduction of acidic oxides, such as FexOy and NbxOy, into the amphoteric H-ZSM-5 catalyst would cover the basic acid sites, leading to the formation of acid sites. The acid sites on the surface of zeolite catalysts were proven to facilitate methanamide formation, which was then deaminated to generate ammonium and formic acid, resulting in enhanced hydrolysis activity and NH3 storage capacity.1 Furthermore, the large FexOy and NbxOy particle sizes inhibited the generation of acid sites and impeded catalytic hydrolysis of HCN. In addition, the interaction between the Fe and Nb species in Fe–Nb/ZSM-5 contributed to the formation of larger quantities of Fe3+, Nb5+ and small particle sizes of FexOy and Nb2O5, which were responsible for the improved surface acidity. This could significantly affect the catalytic hydrolysis of HCN.
3.9.2 Reaction pathway of HCN catalytic hydrolysis. Cant et al.32 suggested that methanamide could directly decompose into NH3 and CO over zeolite-based catalysts above 250 °C. However, the reaction pathway was considered less plausible because of the high thermal stability of methanamide. Combined with the results in Table 2 and Fig. 8, a few ppm of CO were lost compared with the amount of HCN degradation, which might be due to the formation of formic acid, as previously reported.1 Therefore, the reaction pathway of HCN hydrolysis over Fe/ZSM-5, Nb/ZSM-5 and Fe–Nb/ZSM-5 catalysts might be as follows: HCN + H2O → methanamide → ammonium formate → formic acid → H2O + CO.1 Based on the above discussion, a possible detailed reaction pathway for HCN hydrolysis could be described as follows: |
HC(O)NH2 + H2O → HC(O)ONH4
| (2) |
|
HC(O)ONH4 + H2O → HC(O)OH + NH3
| (3) |
4 Conclusion
Fe/ZSM-5, Nb/ZSM-5 and Fe–Nb/ZSM-5 catalysts prepared using an impregnation method were investigated in the catalytic hydrolysis of HCN in the presence of H2O. Fe–Nb/ZSM-5 showed the best HCN hydrolysis activity among the catalysts, achieving 80% HCN conversion at 231–300 °C. XRD results indicated that the Fe and Nb species were highly dispersed on the ZSM-5 catalyst. UV-Vis results implied that Fe–Nb/ZSM-5 possessed a greater abundance of isolated Fe3+, Nb5+, oligomeric FexOy and NbxOy clusters. BET results revealed that Fe–Nb/ZSM-5 had an excellent pore structure. XPS results showed that an interaction between the Fe and Nb species existed, and that the introduction of Nb could contribute to the concentration of Fe3+. Furthermore, Fe3+ and Nb5+ were both active sites in the catalytic hydrolysis of HCN. CO2-TPD and NH3-TPD results demonstrated that both surface basic and acidic sites participated in the catalytic process. The reaction pathway for HCN and H2O was as follows: HCN + H2O → methanamide → ammonium formate → formic acid → H2O + CO.
Acknowledgements
This work is supported by the National Natural Science Foundation of China (No. 21307047) and Academic Newcomer Award of Yunnan Province.
References
- O. Kröcher and M. Elsener, Hydrolysis and oxidation of gaseous HCN over heterogeneous catalysts, Appl. Catal., B, 2009, 92, 75–89 CrossRef.
- P. Dagaut, P. Glarborg and M. U. Alzueta, The oxidation of hydrogen cyanide and related chemistry, Prog. Energy Combust. Sci., 2008, 34, 1–46 CrossRef CAS.
- H. B. Zhao, R. G. Tonkyn, S. E. Barlow, C. H. F. Peden and B. E. Koel, Fractional factorial study of HCN removal over a 0.5% Pt/Al2O3 catalyst: effects of temperature, gas flow rate, and reactant partial pressure, Ind. Eng. Chem. Res., 2006, 45, 934–939 CrossRef CAS.
- H. B. Zhao, R. G. Tonkyn, S. E. Barlow, B. E. Koel and C. H. F. Peden, Catalytic oxidation of HCN over a 0.5% Pt/Al2O3 catalyst, Appl. Catal., B, 2006, 65, 282–290 CrossRef CAS.
- P. N. Brown, G. G. Jayson, G. Thompson and M. C. Wilkinson, Adsorption characteristics of impregnated activated charcoal cloth for hydrogen cyanide, J. Colloid Interf. Sci., 1987, 116, 211–220 CrossRef CAS.
- R. N. Nickolov and D. R. Mehandjiev, Comparative study on removal efficiency of impregnated carbons for hydrogen cyanide vapors in air depending on their phase composition and porous textures, J. Colloid Interface Sci., 2004, 273, 87–94 CrossRef CAS PubMed.
- T. M. Oliver, K. Jugoslav, P. Aleksandar and D. Nikola, Synthetic activated carbons for the removal of hydrogen cyanide from air, Chem. Eng. Process., 2005, 44, 1181–1187 CrossRef CAS.
- O. Kröcher, M. Elsener and E. Jacob, A model gas study of ammonium formate, methanamide and guanidinium formate as alternative ammonia precursor compounds for the selective catalytic reduction of nitrogen oxides in diesel exhaust gas, Appl. Catal., B, 2009, 88, 66–82 CrossRef.
- Y. Gao, X. Chen, J. Zhang, H. Asakura, T. Tanaka, K. Teramura, D. Ma and N. Yan, Popping of graphite oxide: application in preparing metal nanoparticle catalysts, Adv. Mater., 2015, 27, 4688–4694 CrossRef CAS PubMed.
- T. Miyadera, Selective reduction of NOx by ethanol on catalysts composed of Ag/Al2O3 and Cu/TiO2 without formation of harmful by-products, Appl. Catal., B, 1998, 16, 155–164 CrossRef CAS.
- J. D. F. Marsh, W. B. S. Newling and J. Rich, The catalytic hydrolysis of hydrogen cyanide to ammonia, J. Appl. Chem., 1952, 2, 681–684 CrossRef CAS.
- T. Nanba, A. Obuchi, S. Akaratiwa, S. Liu, J. Uchisawa and S. Kushiyama, Catalytic hydrolysis of HCN over H-ferrierite, Chem. Lett., 2000, 29, 986–987 CrossRef.
- N. W. Cant and I. O. Y. Liu, The mechanism of the selective reduction of nitrogen oxides by hydrocarbons on zeolite catalysts, Catal. Today, 2000, 63, 133–146 CrossRef CAS.
- I. O. Y. Liu and N. W. Cant, The formation and reactions of hydrogen cyanide under the conditions of the selective catalytic reduction of NO by isobutane on Cu-MFI, J. Catal., 2000, 195, 352–359 CrossRef CAS.
- S. W. Buckner and R. M. Pope, C–H bond activation versus ring cleavage in the gas phase ionmolecule reactions of Nb+ and Ta+ with toluene and picoline, Int. J. Mass Spectrom., 1999, 182/183, 197–201 CrossRef CAS.
- R. Zhang, Y. Li and T. Zhen, Ammonia selective catalytic reduction of NO over Fe/Cu-SSZ-13, RSC Adv., 2014, 4, 52130–52139 RSC.
- X. Feng, Y. Cao, L. Lan, C. L. Lin, Y. S. Li, H. D. Xu, M. C. Gong and Y. Q. Chen, The promotional effect of Ce on CuFe/beta monolith catalyst for selective catalytic reduction of NOx by ammonia, Chem. Eng. J., 2016, 302, 697–706 CrossRef CAS.
- A. M. Ruiz, G. Dezanneau, J. Arbiol, A. Cornet and J. R. Morante, Insights into the structural and chemical modifications of Nb additive on TiO2 nanoparticles, Chem. Mater., 2004, 16, 862–871 CrossRef CAS.
- L. Sheppard, T. Bak, J. Nowotny, C. C. Sorrell, S. Kumar, A. R. Gerson, M. C. Barnes and C. Ball, Effect of niobium on the structure of titanium dioxide thin films, Thin Solid Films, 2006, 510, 119–124 CrossRef CAS.
- M. Trejda, A. Tuel, J. Kujawa, B. Kilos and M. Ziolek, Niobium rich SBA-15 materials-preparation, characterisation and catalytic activity, Microporous Mesoporous Mater., 2008, 110, 271–278 CrossRef CAS.
- S. Dzwigaj, Y. Millot, C. Méthivier and M. Che, Incorporation of Nb(V) into BEA zeolite investigated by XRD, NMR, IR, DR UV-Vis, and XPS, Microporous Mesoporous Mater., 2010, 130, 162–166 CrossRef CAS.
- L. Ma, H. Z. Chang, S. J. Yang, L. Chen, L. X. Fu and J. H. Li, Relations between iron sites and performance of Fe/HBEA catalysts prepared by two different methods for NH3-SCR, Chem. Eng. J., 2012, 209, 652–660 CrossRef CAS.
- L. Wang, W. Li, G. S. Qi and D. Weng, Location and nature of Cu species in Cu/SAPO-34 for selective catalytic reduction of NO with NH3, J. Catal., 2012, 289, 21–29 CrossRef CAS.
- B. Guan, H. Lin, L. Zhu, B. Tian and Z. Huang, Effect of ignition temperature for combustion synthesis on the selective catalytic reduction of NOx with NH3 over Ti0.9Ce0.05V0.05O2−δ nanocomposites catalysts prepared by solution combustion route, Chem. Eng. J., 2012, 181–182, 307–322 CrossRef CAS.
- M. Anilkumar and W. F. Hoelderich, A one step synthesis of caprolactam out of cyclohexanone by combinded ammoximation and Beckmann rearrangement over Nb-MCM-41 catalysts, Appl. Catal., B, 2015, 165, 87–93 CrossRef CAS.
- J. L. Cao, Y. Wang, X. L. Yu, S. R. Wang, S. H. Wu and Z. Y. Yuan, Mesoporous CuO–Fe2O3 composite catalysts for low-temperature carbon monoxide oxidation, Appl. Catal., B, 2008, 79, 26–34 CrossRef CAS.
- F. D. Liu and H. He, Structure–activity relationship of iron titanate catalysts in the selective catalytic reduction of NOx with NH3, J. Phys. Chem. C, 2010, 114, 16929–16936 CAS.
- K. C. C. Kharas, Performance, selectivity and mechanism in Cu-ZSM-5 lean-burn catalyst, Appl. Catal., B, 1993, 2, 207–224 CrossRef CAS.
- S. Brandenberger, O. Kröcher, A. Tissler and R. Althoff, The state of the art in selective catalytic reduction of NOx by ammonia using metal-exchanged zeolite catalysts, Catal. Rev.: Sci. Eng., 2008, 50, 492–531 CAS.
- T. Zhang, J. Liu, D. X. Wang, Z. Zhao, Y. C. Wei, K. Cheng, G. Y. Jiang and A. J. Duan, Selective catalytic reduction of NO with NH3 over HZSM-5-supported Fe–Cu nanocomposite catalysts: the Fe–Cu bimetallic effect, Appl. Catal., B, 2014, 148–149, 520–531 CrossRef CAS.
- Y. J. Kim, H. J. Kwon, I. Heo, I.-S. Nam, B. K. Cho, J. W. Choung, M.-S. Cha and G. K. Yeo, Mn–Fe/ZSM5 as a low-temperature SCR catalyst to remove NOx from diesel engine exhaust, Appl. Catal., B, 2012, 126, 9–21 CrossRef CAS.
- N. W. Cant, A. D. Cowan, I. O. Y. Liu and A. Satsuma, The reactions of possible intermediates in the selective catalytic reduction of nitrogen oxides by hydrocarbons, Catal. Today, 1999, 54, 473–482 CrossRef CAS.
|
This journal is © The Royal Society of Chemistry 2016 |
Click here to see how this site uses Cookies. View our privacy policy here.