DOI:
10.1039/C6RA23036A
(Paper)
RSC Adv., 2016,
6, 114944-114954
Functional characterizations of interactive recombinant PTEN–silica nanoparticles for potential biomedical applications†
Received
15th September 2016
, Accepted 1st December 2016
First published on 5th December 2016
Abstract
The complex architecture of recombinant proteins poses a major challenge in their formulation and delivery. Preservation of function and structural integrity is the fundamental prerequisite to achieve success in biomedical applications. Amid numerous formulations, maneuvering nanoparticles for optimal protein stabilization is attracting great interest. Herein, we provide a systematic characterization of stabilization of a tumor suppressor protein PTEN onto silica nanoparticles for biomedical applications. PTEN, a critical intracellular signaling protein is down regulated in several cancers leading to abnormal cell growth. The tumor suppression property of PTEN relies on its protein and particularly lipid phosphatase activity. Kinetic parameters Km and kcat/Km of purified GST tagged and untagged PTEN towards para-nitrophenylphosphate and phosphatidylinositol 3,4,5-trisphosphate (diC8) exhibited no significant difference suggesting that the GST tag does not hinder the enzymatic property of PTEN. Structural conformation of silica immobilized GST–PTEN was sustained as evident by circular dichroism measurement. Subsequently, GST–PTEN immobilized onto silica nanoparticles retained its phosphatase activity indicating successful stabilization. A protease protection assay indicated improved stability of the silica nanoparticles bound protein. The effect of GST–PTEN–silica nanoconjugate was evaluated on a PTEN null cell line U-87 MG, which revealed significant reduction in cell proliferation. Maintenance of the functional and structural framework of GST–PTEN immobilized silica nanoparticles along with its anti-proliferative role in glioblastoma cell lines boosts its potential application to combat PTEN deficient conditions.
1. Introduction
Ever since the engineering of recombinant insulin nearly three decades ago, there has been an appreciable increase in available protein based biopharmaceutical drugs including peptides, hormones, recombinant proteins and monoclonal antibodies.1 Recombinant protein comes armed with an array of advantages such as, high yield and specificity,2 low immunogenicity and good tolerance. However, the endeavor to use them as regular therapeutic agents faces challenges due to their inherent complex architecture.3 The macromolecules are generally susceptible to rapid structural disintegration and degradation leading to forfeiture of functionality. Intracellular proteins possess additional limitation of poor membrane permeation.1 This necessitates the application of a suitable stabilization approach to make recombinant proteins acceptable drug candidates.4 While a number of formulations are being characterized to stabilize and deliver proteins viz liposome,5 nanoparticles,6 and injections among others, it is extremely crucial to confirm that the interaction between the protein and carrier does not hamper the active state conformation and activity of the protein.
Formulation of proteins for their sustained delivery for pharmaceutical applications has always been a challenging task. Various model as well as therapeutic proteins such as bovine serum albumin, myoglobin, erythropoietin and rIL-2 has been formulated into polymer coated microparticles to achieve their sustained release for biomedical applications.7–11 The stabilization includes novel emulsion and freezing induced phase separation methods which allow formulation of protein without loss of its biological activity.12–18
Recombinant proteins are emerging as a promising tool to combat disease conditions involving deregulation of signaling pathways. One of the vital cellular signaling pathways frequently altered in cancers is the AKT signaling pathway.19–21 AKT/PKB the pivotal node in the pathway is activated in response to growth factors or insulin and is thought to control a plethora of cellular functions including growth and survival, migration, angiogenesis and transcriptional regulation.19,22 The perturbation of AKT circuit is the underlying cause for complex pathological conditions such as, type 2 diabetes and cancer.22–24 A key upstream regulatory molecule of the pathway is PTEN, a dual specificity phosphatase, catalyzing dephosphorylation of D3 position of phosphatidylinositol 3,4,5-trisphosphate (PIP3) to generate phosphatidylinositol 4,5-diphosphate (PIP2).25,26 Since the discovery of PTEN in 1997, there has been substantial amount of research highlighting its importance in suppressing AKT signaling pathway. The phosphatase domain of 403 amino acid protein resides in N terminal region with the signature motif HCXXGXXR spanning from 123–130 residues.27 However, PTEN is mutated in multiple cancers including brain, breast and prostate leading to nonfunctional protein product.28,29 Contemplating the regulatory role of PTEN, it is vital to acquire functional enzyme to capitalize on its biomedical value. In efforts to stabilize PTEN, silica nanoparticles were synthesized and characterized.
Silica nanoparticles gained attention as drug delivery vehicles due to their excellent properties such as, tunable shape and size, biocompatibility and ease of synthesis.30,31 The facile synthesis of silica nanoparticles with diverse structure and morphologies has always attracted researchers to employ silica for a plethora of biomaterial purposes. Interestingly, silica nanoparticles ranging from 25 to 100 nm in size are reported to cross the blood brain barrier.32 In 2011, FDA approved first-in human clinical trial of small sized nonporous silica nanoparticles highlighting their importance in biomedical applications.30 Besides silica nanoparticles can be readily modified providing great scope for application dependent alterations, for example fluorescent silica nanoparticles offer an attractive tool for cell labeling purposes.33 Since a large number of signaling proteins are intracellular proteins, there is an ever increasing demand for synthesis of efficient nano-carriers for delivery. Silica nanoparticles functionalized with n-octadecyltrimethoxysilane have been successfully employed for intracellular delivery of phospho-AKT antibody into MCF-7 cells resulting in regulation of cellular signaling indicating successful delivery.34,35 Owning to their properties silica was the preferred material for intended protein stabilization and delivery. In this present study, we report maintenance of structural and functional integrity of GST–PTEN immobilized onto silica nanoparticles indicating successful stabilization. Bacterially expressed GST tagged PTEN was purified and characterized by the western blot, MALDI TOF–TOF analysis and circular dichroism spectroscopy study. To excise the affinity tag on-column thrombin cleavage was performed at room temperature. Phosphatase activity of both GST tagged and untagged PTEN was determined by employing artificial protein substrate para-nitrophenylphosphate and lipid substrate phosphatidylinositol 3,4,5-trisphosphate (diC8). To achieve stabilization of GST–PTEN, silica nanoparticles were synthesized by modification of Stober's process and subsequently characterized by physical techniques. Secondary structure and enzymatic function of bound recombinant PTEN was thoroughly investigated by circular dichroism spectroscopy study and phosphatase assay. Maintenance of both structural and kinetic parameters fulfils the fundamental prerequisite to accomplish successful biomedical applications. Stability studies conducted by evaluation of phosphatase activity upon protease digestion of free and silica bound GST–PTEN revealed improved protease protection of silica nanoparticles stabilized GST–PTEN. The protein–silica composite was further tested on PTEN null glioblastoma cell line U-87 MG. The results indicated dose dependent reduction of cell viability upon treatment with the protein–silica nanoconjugate. Cell cycle analysis revealed S phase arrest of U-87 MG cells treated with GST–PTEN SNP conjugate. To the best of our knowledge this is the first and complete report illustrating the immobilization of recombinant PTEN onto silica nanoparticles with comprehensive analysis of the structural changes and the kinetic parameters upon immobilization along with functional effect of the nanobioconjugate on glioblastoma cell line, thereby indicating potential futuristic biomedical applications. The significant investigations of the present work has been illustrated in Scheme 1.
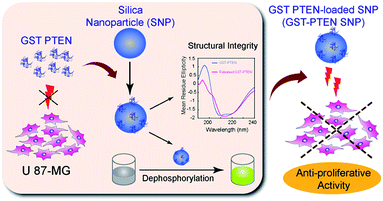 |
| Scheme 1 Schematic outlay of the present work. | |
2. Experimental
2.1 Material
HEPES [N-(2-hydroxyethylo)piperazine-N-(2-ethanesulphonicacid)] and para-nitrophenylphosphate disodium salt (PNPP) were purchased from SRL, isopropyl-β-D-1-thiogalactopyranoside (IPTG) and Luria Bertani (LB) broth were purchased from Himedia, primers were synthesized by IDT technologies Ltd., restriction enzymes from NEB, α-cyano-4-hydroxycinnamic acid, propidium iodide (PI), o-phenylenediamine dihydrochloride (OPD), tetraethyl orthosilicate (TEOS) and DMEM media from Sigma-Aldrich, fetal bovine serum was purchased from Gibco ThermoFisher Scientific, ammonium hydroxide (NH4OH) and ethanol from Merck, thrombin and anti-GST antibody from GE health care, phosphatidylinositol 3,4,5-trisphosphate diC8 (PIP3 diC8) and malachite green assay kit were purchased from Echelon Biosciences Inc., pGEM-T easy vector from Promega, anti-PTEN antibody (raised in rabbit) and HRP conjugated anti-rabbit antibody from CST signalling, RNase from Amresco, tissue culture dishes from Corning Inc., PTEN ORF cloned in pANT_cGST vector was purchased from DNASU (Arizona State University) plasmid repository, Tempe, United States, U-87 MG cell line was obtained from the National Centre for Cell Science (NCCS) cell repository, Pune, India.
2.2 Cloning of human PTEN
The open reading frame of human PTEN was amplified by PCR from pANT_cGST vector procured from DNASU plasmid repository. The detail of PCR conditions is provided in the ESI.† The purified PCR product was then cloned into pGEM-T easy vector. The selected TA clones were digested with BamHI and XhoI and the gene was inserted into bacterial expression vector PGEX-4T-2 that has been previously digested with the same restriction enzymes. PGEX-4T-2 plasmid constructs containing N terminal GST was used to produce GST fusion protein purified by affinity column chromatography.
2.3 Expression, purification and characterization of GST–PTEN
The PGEX-4T-2–PTEN construct was transformed into Escherichia coli BL21 (DE3) for recombinant PTEN protein expression and purification. IPTG induced expression of GST–PTEN was confirmed by SDS-PAGE analysis. Purification of GST tagged PTEN by affinity chromatography is described in detail in the ESI.† Purified, dialyzed and concentrated protein was further used for all characterization and experimental purposes. Bacterially purified GST–PTEN conformation by western blot analysis was performed using anti-PTEN antibody raised in rabbit and anti-rabbit antibody as the primary and secondary antibody, respectively and the blot was developed using chemiluminescent peroxidase substrate. Secondary structure conformational stability and integrity was assessed by circular dichroism (CD). Each spectrum, recorded from 190 nm to 240 nm, was an average of four accumulations. The data points accumulated in millidegree were converted to mean residue ellipticity (expressed in deg cm2 dmol−1) by using the following formula36
where, mean residue weight is molecular weight divided by number of amino acids, pathlength in mm and protein concentration in mg ml−1.
MALDI TOF–TOF analysis was done using 4800 proteomics analyzer with TOF–TOF optics, Applied Biosystems. The results were analyzed using Expasy proteomics Findpept program.37 Detailed procedure of characterization techniques are described in the ESI.†
2.4 Thrombin cleavage and CD spectroscopy of PTEN
To excise the GST tag, on-column thrombin cleavage was performed where PTEN was cleaved from GST while it was still bound to glutathione-agarose beads. The cell lysate after binding and washing with HBS was incubated with thrombin cleavage buffer composed of 50 mM Hepes pH 7.4, 150 mM NaCl and 15 μl of thrombin (0.0004 NIH units μl−1) for 6 h at room temperature. Cleaved PTEN was then eluted and analyzed on 12% SDS-PAGE. Secondary structure spectrum of cleaved PTEN, evaluated by CD spectroscopy, was expressed as mean residue ellipticity (expressed in deg cm2 dmol−1).
2.5 Synthesis and characterization of silica nanoparticles
Silica nanoparticles were synthesized by the protocol described by Clemments et al.38 with slight modifications, procedure described in detail in the ESI.† Synthesis of silica nanoparticles was confirmed by FESEM analysis. Silica nanoparticles alone as well as GST–PTEN bound nanoparticles were characterized by TEM, DLS and zeta potential measurements.
2.6 Immobilization of GST–PTEN on silica NPs
Interaction between protein and nanoparticles can be monitored by various analytical strategies like fluorescence spectroscopy, FTIR spectroscopy, dynamic light scattering, zeta potential and Enzyme Linked Immunosorbent Assay (ELISA). To immobilize GST–PTEN on silica NPs, varying concentrations (6 nM to 30 nM) of GST–PTEN was incubated with constant concentration of silica NPs (0.8 mg ml−1) at room temperature for 2 h. The final volume was made to 1 ml using 25 mM Hepes pH 7.4. Following incubation, the samples were centrifuged at 10
000 rpm for 10 min, and the pellet was re-dispersed in 1 ml Milli-Q water. To determine binding, fluorescence intensity of protein was recorded at a wavelength of 340 nm for an excitation wavelength of 280 nm using Fluoromax-4, Horiba JobinYvon, Edison, NY, USA. Binding percentage was calculated as follows-
To corroborate successful interaction between the recombinant protein and nanoparticles, ELISA was performed. The overall strategy was similar to bead-based ELISA platform, where the silica nanoparticles were used as beads to capture recombinant PTEN. This method was advantageous due to requirement of relatively small amount of protein sample.39 ELISA was performed with maximum binding ratio of protein to nanoparticles. After binding 100 μl of the samples in triplicate were distributed in 1.5 ml eppendorf tubes previously treated with blocking buffer (TBS with 2% BSA) for 12 h. Samples were incubated at room temperature under slow rocking conditions for 4 h and washed with PBS and finally centrifuged. The pellet was re-dispersed in blocking buffer and incubated at room temperature for 2 h. Following washing with TBS, 100 μl of TBS containing anti-PTEN primary antibody raised in rabbit (1
:
1000 dilution) was added to each sample and incubated overnight at 4 °C. Subsequently, the samples were washed with TBS twice followed by addition of HRP conjugated anti-rabbit secondary antibody. After washing with TBS twice, the samples were incubated with o-phenylenediamine dihydrochloride (OPD) substrate. The product developed was measured at 450 nm using Tecan infinite M200 PRO multiplate reader. All centrifugation was performed at 8000 rpm for 5 min. Protein binding was also studied by Fourier Transform Infrared (FTIR) spectroscopy, zeta potential and DLS measurements. A detailed description of these experiments is provided in the ESI.†
2.7 Release studies of protein from silica NPs
To study cumulative protein release the ratio of protein to nanoparticle exhibiting maximum binding percentage was selected. After binding, the sample was centrifuged at 10
000 rpm for 10 min and the pellet was re-distributed in 10 mM Tris pH 7.4. The sealed tubes were placed in water bath maintaining temperature at 37 °C. Samples taken out at specific time intervals ranging from 1 to 72 h were centrifuged at 10
000 rpm for 10 min. The supernatant was collected and fluorescence of protein was probed to determine the amount of protein released using Fluoromax-4, Horiba Jobin Yvon, Edison, NY, USA.
2.8 Protein phosphatase assay
Assessment of in vitro phosphatase activity was done using artificial substrate, para-nitrophenylphosphate as substrate. Optimal temperature for enzyme activity was determined by conducting PNPP phosphatase assay at different temperatures ranging from 25 °C to 45 °C for 60 min in buffer composed of 25 mM Hepes pH 7.4, 10 mM DTT and 1 μg of purified GST tagged and untagged enzyme. The optimal temperature range for both GST tagged and untagged PTEN was in the range of 35 °C to 40 °C. Subsequently, activation energy was calculated from the data obtained for temperature dependent PNPP assay. Phosphatase activity assay of GST tagged, untagged and silica immobilized enzyme was performed at 37 °C in the same buffer composition as mentioned above. Stop solution added after the specified incubation period served dual purpose; terminate the reaction and intensify the colour of the product that can be measured by colorimetric analysis. Under alkaline conditions the product para-nitrophenol is converted to para-nitrophenolate which was measured by absorbance at 405 nm using Perkin Elmer Victor X3 multiplate reader. Only substrate control was kept to eliminate potential nonspecific phosphate release. The amount of phosphate released was estimated against a standard curve by linear regression analysis.40
2.9 Lipid phosphatase assay
Lipid phosphatase assay was performed using water soluble phosphatidylinositol 3,4,5-trisphosphate diC8 (PIP3 diC8) ranging from 15 to 150 μM in 25 μl of reaction mixture consisting of 25 mM Hepes pH 7.4, 10 mM DTT, 2 mM EDTA and 1 μg of purified GST tagged, untagged and silica immobilized enzyme. The phosphate released was quantified by colorimetric assay using malachite green solution. Detection of phosphate is based on formation of complex between free phosphate and molybdate (malachite green) which was quantified by absorbance at 660 nm using Perkin Elmer Victor X3 multiplate reader. The amount of phosphate released was determined by standard linear regression analysis.
2.10 Protease protection assay
Free and silica bound GST–PTEN were incubated with 0.5 μg ml−1 of proteinase K for 30 min at 37 °C. After proteinase K digestion the samples were analyzed by phosphatase assay using artificial protein substrate PNPP. Phosphatase assay of the treated and untreated protein samples was performed using 50 mM PNPP at 37 °C for 60 min following colorimetric quantification of the product formed at 405 nm.
2.11 Cell viability assay
Glioblastoma cell line U-87 MG seeded in 96 well plate at a density of 4000 cells per well in DMEM media supplemented with 10% FBS was allowed to attach overnight. PTEN expression was checked in U-87 MG cell line, prior to treatment, by using gene specific primers. There was no expression of PTEN in the cells as documented in literature. Following attachment, media was discarded and fresh serum media containing varying concentrations of silica nanoparticles alone and GST–PTEN bound silica nanoparticles were added to the seeded cells. After 48 h incubation, serum media containing 5 μl of 5 mg ml−1 MTT solution was added to each well and the plates were incubated at 37 °C under humidified atmosphere of 5% CO2. Subsequently, the media was discarded and DMSO was added to dissolve the formazan crystals formed by enzymatic conversion by live cells. The product was measured by absorbance at 570 nm along with background measurement at 690 nm using multiplate reader (Tecan, Infinite M200PRO). Cell viability (%) relative to the control untreated cells was calculated using the following formula-
2.12 Cell cycle analysis
The effect of the bioconjugate on the cell cycle pattern of U-87 MG cells was determined by flow cytometry using propidium iodide (PI) DNA staining dye. Glioblastoma cell line U-87 MG seeded in 60 mm tissue culture Petri plates at a density of 105 cells per ml were allowed to attach overnight. Prior to treatment the cells were synchronized by serum starvation for 48 h. Cells were then treated with GST–PTEN alone, silica nanoparticles alone and GST–PTEN bound silica nanoparticles for 48 h in serum DMEM media. The cell collected by trypsinization were fixed by adding chilled 70% ethanol drop wise while vortexing the pellet, and stored at −20 °C until further analysis.
The fixed samples were centrifuged and washed with chilled 1× PBS following incubation with RNase at 37 °C for 1 h. Samples were then incubated with PI until analysis using BD FACS Calibur. The data collected was examined using ModFiT LT software.
3. Results and discussion
3.1 Purification of recombinant PTEN
Human PTEN clone in PGEX-4T-2 vector was confirmed by restriction digestion using BamHI and XhoI (Fig. S1A†). The sequence was confirmed by sequencing using gene specific primers. The PGEX-4T-2–PTEN construct was then transformed into E. coli BL21 for recombinant protein expression. Optimal conditions for expression of the recombinant protein were 0.5 mM IPTG concentration, 4 h induction time at a temperature of 26 °C. At small scale of 5 ml secondary culture when the bacterial cells were lysed and analyzed on SDS gel, most of the induced protein was found to be in the insoluble fraction with a small amount in the soluble fraction (Fig. S1B†). However, upon increasing the culture volume to 500 ml, considerable amount of induced PTEN was found in the soluble fraction. Therefore, the supernatant fraction was used without solubilizing the pellet so as to avoid the use of detergents during the course of purification. Purification was carried out using glutathione-agarose affinity chromatography. A band of around 81 kDa corresponding to GST–PTEN was observed when the purified fraction was run on 12% SDS PAGE (Fig. 1A). A representative image has also been presented in the ESI (Fig. S2A†) that depicted homogenous purification of recombinant PTEN. Protein yield determined by Bradford assay was approximately 5 mg per liter of E. coli culture. For purified PTEN, after on-column thrombin cleavage, a band around 55 kDa was observed on 12% SDS PAGE (Fig. 1B).
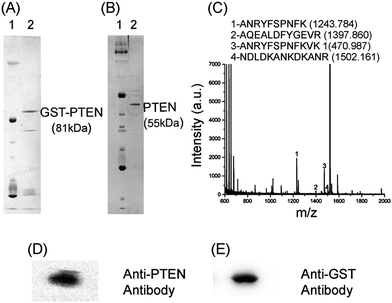 |
| Fig. 1 Purification and characterization of GST–PTEN. (A) SDS PAGE profile of GST–PTEN, lane 1 shows 2–212 kDa protein ladder and lane 2 shows purified GST–PTEN. (B) SDS PAGE profile of PTEN, lane 1 shows 2–212 kDa protein ladder and lane 2 shows purified PTEN. (C) MALDI TOF–TOF analysis of purified GST–PTEN. (D) Western blot with anti-PTEN antibody. (E) Western blot with anti-GST antibody. | |
3.2 Characterization of recombinant protein
The purified recombinant protein was further analyzed by MALDI TOF–TOF and circular dichroism.
The MS/MS profile obtained by MALDI TOF–TOF analysis of trypsin digested peptide fragments inspected using findpept expasy tool, generated match of the peptide sequence NDLDKANKDKANRYFSPNFKVK with sequence query coverage of 16.13% (Fig. 1C). Protein purified to homogeneity was investigated by the western blotting using anti-PTEN antibody and anti-GST antibody which identified a band corresponding to 81 kDa (Fig. 1D and E). The full blot image is presented in the ESI (Fig. S2B†).
Secondary structure of GST–PTEN and PTEN was characterized by far UV circular dichroism spectroscopy (Fig. S3†). The spectrum investigated by Yang's reference revealed the content of secondary structural elements to be 24.5% α-helix, 25.6% β-sheet and 27.8% random coil for GST–PTEN and 20.8% α-helix, 22.7% β-sheet, 34.3% random coil for PTEN. The result was in accordance with the available crystal structure of PTEN in PDB (1D5R), where the estimated secondary structural elements were 19.603% α-helix and 24.5% β-sheet.
3.3 Generation and characterization of silica NPs
Silica nanoparticles were synthesized by slight modification of the protocol developed by Clemments et al.
The size and shape of the nanoparticles were examined by FESEM, TEM, and DLS analysis. FESEM analysis of the silica nanoparticles displayed synthesis of spherical particles with average diameter of 60 ± 10 nm (Fig. S4A†).
The size and morphology of nanoparticles was further confirmed by TEM, which validated the FESEM results with average diameter of 55 ± 10 nm (Fig. 2A). Average diameter was calculated from several images consisting of 100 particles using Image J software (Fig. 2C). The hydrodynamic diameter of the nanoparticles determined by DLS was found to be 116.5 nm.
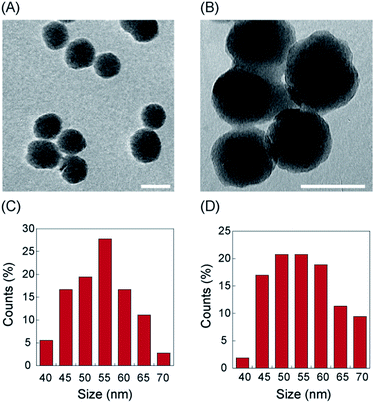 |
| Fig. 2 (A) TEM image of silica nanoparticles (scale bar 50 nm, average diameter 55 ± 10 nm). (B) TEM image of GST–PTEN bound silica NPs (scale bar 50 nm, average diameter 58 ± 10 nm). (C) Particle size distribution of silica nanoparticles calculated using Image J software. (D) Particle size distribution of GST–PTEN bound silica NPs calculated using Image J software. | |
3.4 Immobilization and release of protein from silica NPs
Adsorption of GST–PTEN on silica nanoparticles was determined by fluorescence spectroscopy, DLS measurements, ELISA and FTIR study. TEM images of GST–PTEN immobilized silica nanoparticles revealed no significant change in the average size and morphology of the nanoparticles after binding of the protein (Fig. 2B). Average diameter was calculated from several images consisting of 100 particles using Image J software (Fig. 2D). The average diameter was in the range of 58 ± 10 nm.
Binding percentage was calculated by probing the intrinsic fluorescence of protein in 25 mM Hepes pH 7.4 (Fig. 3A). The maximum binding percentage was calculated to be 49%, at a protein concentration of 12 nM (Fig. S4B†).
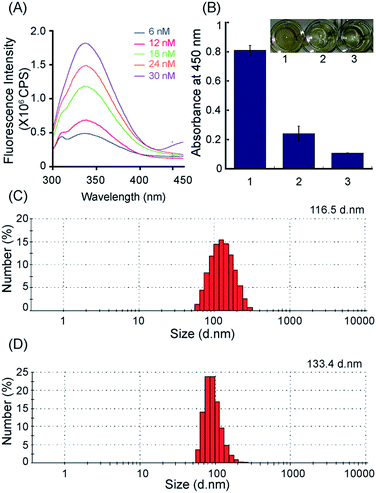 |
| Fig. 3 (A) Immobilization of GST–PTEN onto silica nanoparticles as determined by probing the intrinsic fluorescence of protein. (B) ELISA for analyses of binding of GST–PTEN to silica nanoparticles, 1 is GST–PTEN immobilized on silica nanoparticles. 2 is only silica nanoparticles and 3 is only buffer. (C) Dynamic light scattering data of silica nanoparticles (average diameter of 116.5 nm). (D) Dynamic light scattering data of GST–PTEN bound silica nanoparticle (average diameter of 133.4 nm). | |
Bead-based ELISA was performed using anti-PTEN primary antibody, HRP conjugated secondary antibody and o-phenylenediamine dihydrochloride (OPD) as substrate.
The product measured at 450 nm showed substantial difference between GST–PTEN SNP and SNP control, indicating successful interaction between the recombinant PTEN and the silica nanoparticles (Fig. 3B).
Hydrodynamic diameter of GST–PTEN immobilized silica nanoparticles was found to be 133.4 nm.
The increase in the diameter as compared to free silica nanoparticles was indicative of binding of protein with the nanoparticles (Fig. 3C and D).
Zeta potential measurements show a slight shift in the zeta potential of the nanoparticles upon incubation with GST–PTEN indicating protein–nanoparticle interaction (Fig. S5†). The FTIR spectrum of silica nanoparticles demonstrated peaks at 3441 cm−1, 1096 cm−1 and 803 cm−1 corresponding to Si–OH stretching, Si–O–Si asymmetric stretching and Si–O–Si bending, respectively.41 The FTIR spectrum of recombinant PTEN displayed signature peaks at 1642 cm−1 (amide I), 1538 cm−1 (amide II), 1318 cm−1 (amide III), 668 cm−1 (amide IV) arising from C
O stretching, CN stretching, NH bending, OCN bending, respectively.42,43
Observation of peaks corresponding to characteristic bands of peptide linkage in the FTIR spectra of recombinant protein incubated with silica nanoparticles indicated the binding of protein to nanoparticles (Fig. S6†).
To investigate release, protein bound silica nanoparticles were re-dispersed in 10 mM Tris pH 7.4 and incubated at 37 °C.
The release profile demonstrated an increase in release with increasing time with 55% release of recombinant PTEN within 72 h (Fig. 4A). The release pattern ensures rapid release of the protein within stipulated time of 72 h at physiological pH.
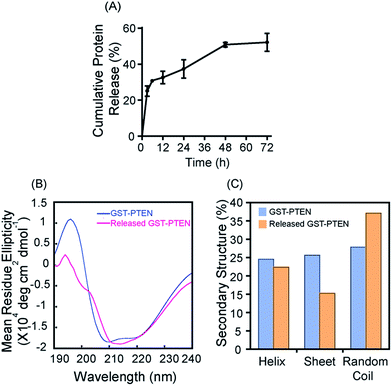 |
| Fig. 4 (A) Release profile of GST–PTEN over a period of 72 h in 10 mM Tris pH 7.4 at 37 °C. (B) CD spectra of purified GST–PTEN and released GST–PTEN. (C) Comparative study of content of secondary structural elements of purified GST–PTEN and GST–PTEN released from silica nanoparticles. | |
However, surface interactions can be further tuned to obtain sustained release for prolonged period.44 Modifications such as polymeric microencapsulation of the protein may also significantly improve the sustained release of the protein as in case of sustained release of erythropoietin, a recombinant protein used for anemia treatment, from PLGA microspheres for prolonged period suitable for pharmaceutical applications.11
3.5 Protein structural analysis
Structural framework of protein holds the essence for its function. Adsorption of proteins on solid surfaces may induce structural changes depending upon nature and size of nanomaterials.45,46 The structural changes influenced by interaction of protein with the nanoparticles dictate the functional behaviour of the protein. Hence, preservation of macromolecule structure is essential to achieve intended biological applications. Stability of the protein can be further enhanced by conjugation of protein to co-solvents such as glycerol or polyethylene glycol (PEG).9 Stabilization of protein is crucial during protein delivery to avoid aggregation upon release in aqueous medium to maintain its biological applications.
To track the structural changes induced by immobilization of GST–PTEN onto silica nanoparticles secondary structures of free GST–PTEN and GST–PTEN released from silica nanoparticles in 10 mM Tris pH 7.4 were analyzed by circular dichroism (Fig. 4B). Structural elements were determined using Yang's reference47 (Fig. 4C), which essentially revealed no pronounce difference between the secondary structural elements of released GST–PTEN (22.4% α-helix, 15.2% β-sheet) compared to free GST–PTEN (24.5% α-helix, 25.6% β-sheet) indicating nominal structural reorganization upon interaction with silica nanoparticles.45
Decrease in helical content may be attributed to the adsorption of protein onto the nanoparticles as reported previously.45 Structural changes in protein influenced by interaction with silica nanoparticles have been previously documented for BSA and myoglobin.46 Both the proteins displayed nanoparticle size dependent conformational change with no significant structural alteration for smaller sized nanoparticles. However, there is no such study for interaction of the GST–PTEN with silica nanoparticles.
Additional experiment was performed to assess the conformational change of the protein immediately upon interaction with the nanoparticles (Fig. S7†). Monitoring shift at 222 nm of protein bound to silica nanoparticles by circular dichroism study revealed that the initial conformational change was slow indicating that the interaction between the recombinant protein and silica nanoparticles did not rapidly perturb the folding and stability of the protein. The analysis of secondary structural elements of free GST–PTEN and released GST–PTEN revealed that the interaction between the protein and the nanoparticles did not cause profound change in the structural properties of GST–PTEN released from nanoparticles surface. Structural reorganization is inevitable during protein–nanoparticle interactions. Nonetheless in case of interaction between GST–PTEN and silica nanoparticles the minimal framework reorganization, ubiquitous for all protein–nanoparticle interactions, provided a strong motivation for further kinetic evaluation of the bound protein.
3.6 Evaluation of enzyme kinetics
PTEN, a dual specificity phosphatase, contains the signature motif HCXXGXXR in consensus with the family of PTPase.26 Although PTEN dephosphorylates artificial protein substrates such as para-nitrophenylphosphate; it has higher catalytic affinity towards lipid substrates.26 Assaying PTEN requires a reducing environment40 to retain the active-site cysteine residue in its reduced state.48 Phosphatase activity of PTEN towards PNPP and PIP3 diC8 was determined (Fig. 5). Assay results indicated that activity (nmole of phosphate released) over increasing concentration of substrate fits Michaelis–Menten kinetics.
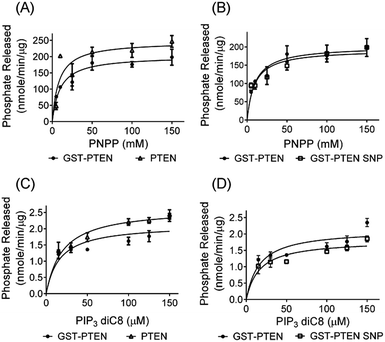 |
| Fig. 5 Kinetic profile towards PNPP of (A) GST tagged and untagged PTEN. (B) Free and silica immobilized GST tagged PTEN, kinetic profile towards diC8 PIP3 of (C) GST tagged and untagged PTEN. (D) Free and silica immobilized GST tagged PTEN. | |
Kinetic parameters Km and kcat/Km were assessed using GraphPad Prism software. The kinetic analysis essentially revealed no significant difference between the catalytic efficiency of GST–PTEN and PTEN towards both artificial protein substrate and lipid substrate, suggesting that the presence of GST tag did not hinder the function of the protein.
PTEN catalysis was extensively evaluated using lipid substrates such as, inositol phosphate I(1,3,4,5)P4 and diC6 PIP3. The kinetic parameters Km and kcat/Km reported for inositol phosphate I(1,3,4,5)P4 and diC6 PIP3 were 98.6 μM and 67 μM and 0.005 and 0.038 min−1 μM−1, respectively.26,49
The experimentally obtained kinetic parameters of GST–PEN are comparable to previous reports with Km of 16.40 μM and kcat/Km of 0.0108 min−1 μM−1. Thus, comparative study of the kinetic values confirms that GST–PTEN is catalytically active and can be subjected to immobilization onto silica nanoparticles. Additional considerations for retaining GST is that the affinity tag may also assist in protein folding, protein immobilization onto nanoparticle, facile one step purification and stabilization of the enzyme.50,51 To eliminate potential interference of GST in enzyme kinetic reactions, phosphatase activity of GST was examined using para-nitrophenylphosphate (PNPP) as substrate. The results indicate no phosphatase activity of the GST protein (Fig. S8†). Immobilization of PTEN onto silica nanoparticles was also conducted, which revealed a maximum binding percentage of 30% (Fig. S9†) as compared to maximum binding percentage of 49% for GST–PTEN immobilization suggesting that GST may facilitate in protein–nanoparticle interaction. Therefore, it was decided to proceed with GST tagged PTEN for immobilization onto silica nanoparticles. Surface morphology, size and type of nanoparticles greatly influence the activity of the adsorbed protein. In many cases, interaction between nanoparticles and protein causes dramatic loss of enzymatic activity.52,53 Nevertheless, maintenance of released GST–PTEN structure could possibly entail maintenance of native function as well. Therefore, we embarked on the study of phosphatase activity of immobilized protein towards the same substrates PNPP and PIP3 diC8. It was interesting to observe that the phosphatase activity of immobilized GST–PTEN followed Michaelis–Menten kinetics with kinetic values as illustrated in Table 1.
Table 1 Kinetic parameters for catalysis by GST–PTEN, PTEN and silica bound GST–PTEN
Substrate |
Sample |
Km (μM) |
kcat/Km (min−1 μM−1) |
PNPP |
GST–PTEN |
6881 |
0.16 × 10−3 |
PTEN |
3767 |
0.22 × 10−3 |
GST–PTEN SNP |
6102 |
0.15 × 10−3 |
PIP3 diC8 |
GST–PTEN |
16.40 |
0.0108 |
PTEN |
19.90 |
0.0110 |
GST–PTEN SNP |
16.31 |
0.0092 |
The kinetic parameters of immobilized GST–PTEN were comparable to its free counterpart implying retention of functional integrity upon immobilization.
The results are also consistent with evidences available that smaller sized nanoparticles are known to uphold the native properties of the protein as compared to larger sized nanoparticles of 100 nm or above.52
Our studies bestow a positive lead towards utilization of small sized silica nanoparticles as a suitable vehicle for stabilization and delivery of recombinant PTEN for potential remedial functions.
3.7 Protease protection assay
Following proteinase K digestion of free and silica bound GST–PTEN, the samples were analyzed by phosphatase assay to understand the effect of the digestion on the enzymatic capacity of the protein. The overall strategy of the experiment is explained in Fig. 6A. Protease treated and untreated GST–PTEN and treated silica bound GST–PTEN was subjected to PNPP catalysis using 50 mM of the substrate at 37 °C for 60 min. The assay results indicated that the catalysis of treated silica bound GST–PTEN was higher as compared to treated free GST–PTEN. Bound GST–PTEN may not be as easily accessible to proteinase K for digestion as compared to the free enzyme. Therefore, lower digestion of the bound enzyme replicated in higher phosphatase activity as compared to free enzyme (Fig. 6B). Statistical analysis was performed by ANOVA using GraphPad Prism software with statistical significance denoted by * (p < 0.05).
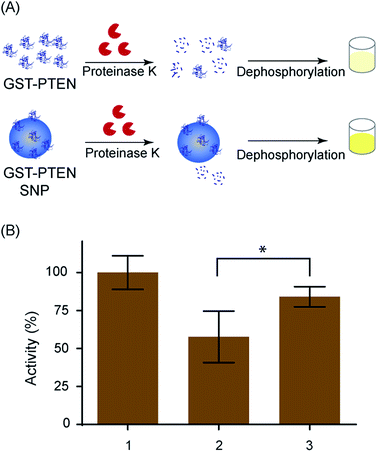 |
| Fig. 6 (A) Scheme explaining the strategy of protease protection assay. (B) Phosphatase assay of proteinase K treated and untreated samples, 1 is untreated GST–PTEN, 2 is treated GST–PTEN and 3 is treated silica bound GST–PTEN. | |
3.8 Cell viability assay
PTEN alterations in gliomas and particularly glioblastomas result in translation of truncated and non-functional protein products. PTEN expression was checked in glioblastoma cell line U-87 MG and no expression of PTEN was detected (Fig. S10†). PTEN overexpression by gene therapy approaches has shown promising results in inhibiting tumor growth both in vitro and in vivo.54,55 However, the stabilization and direct delivery of exogenous PTEN protein using suitable delivery vehicle is an area of research still unexplored. The idea of gene therapy is ultimately the expression of the gene product. Therefore, direct delivery of the functional PTEN protein may produce the desired effects earlier and may be beneficial in many cases where the host is unable to translate the functional protein from the gene.34
To attain this, GST–PTEN was immobilized onto silica nanoparticles and the protein-nanoparticle interactions were thoroughly investigated. Silica stabilized GST–PTEN displayed sound structure, function and stability, therefore the subsequent judicious step was to study the effect of this nanoconjugate on cancer cell lines. U-87 MG cells were treated with varying concentration of GST–PTEN alone for 48 h, which displayed no significant reduction in the viability of the treated cells (Fig. S11†). PTEN null U-87 MG cells were treated with varying concentrations of silica nanoparticles (varying from 0.9 mg ml−1 to 5.4 mg ml−1) and silica bound GST–PTEN (protein concentration varying from 0.375 μg ml−1 to 2.25 μg ml−1) for 48 h. Silica bound GST–PTEN exhibited dose dependent reduction of cell viability as indicated by the assay (Fig. 7A). Although, gene therapy and protein therapy are two different strategies to combat disease conditions, the present illustration of anti-proliferative activity of PTEN protein with significantly higher reduction in 48 h treatment as compared to gene therapy56,57 modules indicates potential therapeutic implication of this mode of treatment. The slight reduction in viability of cells treated with silica nanoparticles could be due to their high concentration and small size. Silica nanoparticles have been reported to induce size and concentration dependent toxicity with small sized nanoparticles ranging from 25 nm to 75 nm being more toxic as compared to larger nanoparticles. However, comparative analysis between the silica nanoparticles and the recombinant protein bound nanoparticles showed protein bound nanoparticles were more effective in reducing the viability of the cells. Statistical analysis was performed by ANOVA using GraphPad Prism software with statistical significance denoted by * (p < 0.05). A number of signalling pathways are deregulated in multi drug resistance U-87 MG. This makes their treatment difficult with application of a single drug. However, reduction in viability by silica bound GST–PTEN paves the way for potential application of the protein in a co-therapy module.
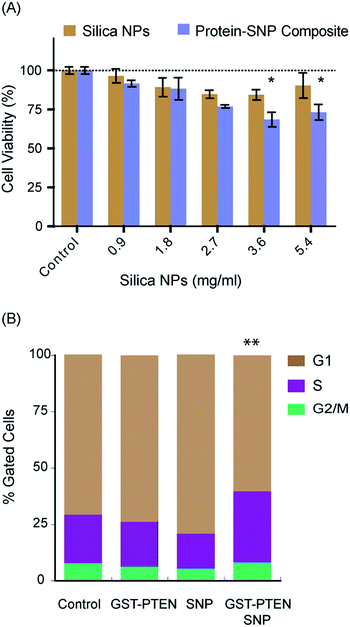 |
| Fig. 7 (A) Assessment of viability of U-87 MG cells upon treatment with free and protein bound silica nanoparticles for 48 h by MTT assay. (B) Cell cycle analysis of U-87 MG cells by flow cytometry upon treatment with free and GST–PTEN bound silica nanoparticles for 48 h. | |
3.9 Cell cycle analysis
To understand the effect of GST–PTEN SNP bioconjugate on the cell cycle progression of U-87 MG cells, the treated and untreated cells were incubated with PI and analyzed using flow cytometry. PI, a fluorescent dye, is capable of binding and thereby labelling DNA stiochiometrically. Analysis of silica bound GST–PTEN treated U-87 MG cells displayed S phase arrest, with a 1.47 fold increase compared to untreated cells (Fig. 7B). It is already well documented that the PTEN regulated AKT signalling pathway,58 is involved in S phase cell cycle regulation.59 Therefore, the reduction in the viability of the treated cells proposes silica bound GST–PTEN as a potential candidate for biomedical applications. Statistical analysis was performed by ANOVA using GraphPad Prism software with statistical significance denoted by * (p < 0.05), ** (p < 0.01).
4. Conclusions
Existence of strong correlation between structure and function for all biological entities implies that both structure and function needs to be conserved to meet successful delivery formulation. In this present work, we have purified a critical tumor suppressor protein PTEN. Both GST tagged and untagged proteins were characterized by biophysical techniques and enzymatic assay. Detailed analysis of kinetic parameters towards para-nitrophenylphosphate and PIP3 diC8 suggested that the native phosphatase activity of both tagged and untagged PTEN was preserved after bacterial purification and their kinetic efficiencies were comparable. Subsequently, successfully immobilization of GST–PTEN onto silica nanoparticles was validated by fluorescence spectroscopy, FTIR spectroscopy, ELISA and DLS. The nanosystem ensured efficient release of the protein with intact structural integrity as examined by circular dichroism spectroscopy. Immobilization of recombinant PTEN onto silica nanoparticles demonstrated preservation of dual phosphatase activity when compared to the free enzyme indicating that immobilization does not alter the enzymatic activity of GST–PTEN. Higher phosphatase activity of protease treated bound GST–PTEN as compared to free protein imply better stability of the protein upon adsorption onto the nanoparticles. Establishment of anti-proliferative role of bacterially purified exogenous recombinant PTEN immobilized on silica nanoparticles on resistant cell line U-87 MG could empower future application of PTEN based therapy. Thus, the results attained addresses the major challenges in protein stabilization and paves the way for using immobilized PTEN in biomedical applications.
Acknowledgements
We greatly acknowledge financial supports of the Department of Biotechnology, Government of India, for DBT Programme Support (BT/PR13560/COE/34/44/2015) grant. We also acknowledge partial support of the Department of Biotechnology (DBT-NER/Health/47/2015) and the Department of Electronics and Information Technology, Government of India (No. 5(9)/2012-NANO (Vol. II)). Instrumentation support of the Centre for Nanotechnology and the Central Instruments Facility (CIF) at IIT Guwahati is also acknowledged.
References
- S. Mitragotri, P. A. Burke and R. Langer, Nat. Rev. Drug Discovery, 2014, 13, 655–672 CrossRef CAS PubMed.
- B. Leader, Q. J. Baca and D. E. Golan, Nat. Rev. Drug Discovery, 2008, 7, 21–39 CrossRef CAS PubMed.
- S. D. Putney and P. A. Burke, Nat. Biotechnol., 1998, 16, 153–157 CrossRef CAS PubMed.
- Y. Lu, J. Yang and E. Sega, AAPS J., 2006, 8, E466–E478 CrossRef CAS PubMed.
- L. P. Herrera Estrada and J. A. Champion, Biomater. Sci., 2015, 3, 787–799 RSC.
- R. H. Utama, Y. Guo, P. B. Zetterlund and M. H. Stenzel, Chem. Commun., 2012, 48, 11103–11105 RSC.
- H. Zhao, F. Wu, Y. Cai, Y. Chen, L. Wei, Z. Liu and W. Yuan, Int. J. Pharm., 2013, 450, 235–240 CrossRef CAS PubMed.
- D. Xu, Z. Hu, J. Su, F. Wu and W. Yuan, Nano-Micro Lett., 2012, 4, 118–123 CrossRef CAS.
- W. Yuan, Y. Geng, F. Wu, Y. Liu, M. Guo, H. Zhao and T. Jin, Int. J. Pharm., 2009, 366, 154–159 CrossRef CAS PubMed.
- S. Yang, W. Yuan and T. Jin, Expert Opin. Drug Delivery, 2009, 6, 1123–1133 CrossRef CAS PubMed.
- Y. Geng, W. Yuan, F. Wu, J. Chen, M. He and T. Jin, J. Controlled Release, 2008, 130, 259–265 CrossRef CAS PubMed.
- Z. Hu, M. Liao, Y. Chen, Y. Cai, L. Meng, Y. Liu, N. Lv, Z. Liu and W. Yuan, Int. J. Nanomed., 2012, 7, 5719–5724 CAS.
- G. Liu, X. Hong, M. Jiang and W. Yuan, Int. J. Nanomed., 2012, 7, 4559–4569 CAS.
- W. Yuan and Z. Liu, Int. J. Nanomed., 2012, 7, 257–270 CrossRef CAS PubMed.
- W. Yuan, Y. Zhang, F. Wu, H. Li, Y. Cai, Y. Zhang, M. Han and T. Jin, Colloids Surf., B, 2010, 79, 326–333 CrossRef CAS PubMed.
- W. Yuan, F. Wu, M. Guo and T. Jin, Eur. J. Pharm. Sci., 2009, 36, 212–218 CrossRef CAS PubMed.
- W. Yuan, F. Wu, Y. Geng, S. Xu and T. Jin, Int. J. Pharm., 2007, 339, 76–83 CrossRef CAS PubMed.
- T. Jin, J. Zhu, F. Wu, W. Yuan, L. L. Geng and H. Zhu, J. Controlled Release, 2008, 128, 50–59 CrossRef CAS PubMed.
- L. Zhu, B. Derijard, K. Chakrabandhu, B.-S. Wang, H.-Z. Chen and A.-O. Hueber, Cancer Lett., 2014, 354, 355–364 CrossRef CAS PubMed.
- D. A. Altomare and J. R. Testa, Oncogene, 2005, 24, 7455–7464 CrossRef CAS PubMed.
- J. Luo, B. D. Manning and L. C. Cantley, Cancer Cell, 2003, 4, 257–262 CrossRef CAS PubMed.
- B. D. Manning and L. C. Cantley, Cell, 2007, 129, 1261–1274 CrossRef CAS PubMed.
- B. R. Balsara, J. Pei, Y. Mitsuuchi, R. Page, A. Klein-Szanto, H. Wang, M. Unger and J. R. Testa, Carcinogenesis, 2004, 25, 2053–2059 CrossRef CAS PubMed.
- S. N. Malik, M. Brattain, P. M. Ghosh, D. A. Troyer, T. Prihoda, R. Bedolla and J. I. Kreisberg, Clin. Cancer Res., 2002, 8, 1168–1171 Search PubMed.
- M. P. Myers, J. P. Stolarov, C. Eng, J. Li, S. I. Wang, M. H. Wigler, R. Parsons and N. K. Tonks, Proc. Natl. Acad. Sci. U. S. A., 1997, 94, 9052–9057 CrossRef CAS.
- T. Maehama and J. E. Dixon, J. Biol. Chem., 1998, 273, 13375–13378 CrossRef CAS PubMed.
- J.-O. Lee, H. Yang, M.-M. Georgescu, A. Di Cristofano, T. Maehama, Y. Shi, J. E. Dixon, P. Pandolfi and N. P. Pavletich, Cell, 1999, 99, 323–334 CrossRef CAS PubMed.
- J. Li, C. Yen, D. Liaw, K. Podsypanina, S. Bose, S. I. Wang, J. Puc, C. Miliaresis, L. Rodgers, R. McCombie, S. H. Bigner, B. C. Giovanella, M. Ittmann, B. Tycko, H. Hibshoosh, M. H. Wigler and R. Parsons, Science, 1997, 275, 1943–1947 CrossRef CAS PubMed.
- P. A. Steck, M. A. Pershouse, S. A. Jasser, W. K. Yung, H. Lin, A. H. Ligon, L. A. Langford, M. L. Baumgard, T. Hattier, T. Davis, C. Frye, R. Hu, B. Swedlund, D. H. Teng and S. V. Tavtigian, Nat. Genet., 1997, 15, 356–362 CrossRef CAS PubMed.
- L. Tang and J. Cheng, Nano Today, 2013, 8, 290–312 CrossRef CAS PubMed.
- M. Benezra, O. Penate-Medina, P. B. Zanzonico, D. Schaer, H. Ow, A. Burns, E. DeStanchina, V. Longo, E. Herz, S. Iyer, J. Wolchok, S. M. Larson, U. Wiesner and M. S. Bradbury, J. Clin. Invest., 2011, 121, 2768–2780 CAS.
- S. Hanada, K. Fujioka, Y. Inoue, F. Kanaya, Y. Manome and K. Yamamoto, Int. J. Mol. Sci., 2014, 15, 1812–1825 CrossRef PubMed.
- S. J. Soenen, B. Manshian, S. H. Doak, S. C. De Smedt and K. Braeckmans, Acta Biomater., 2013, 9, 9183–9193 CrossRef CAS PubMed.
- S. S. Bale, S. J. Kwon, D. A. Shah, A. Banerjee, J. S. Dordick and R. S. Kane, ACS Nano, 2010, 4, 1493–1500 CrossRef CAS PubMed.
- Y. Niu, M. Yu, J. Zhang, Y. Yang, C. Xu, M. Yeh, E. Taran, J. J. C. Hou, P. P. Gray and C. Yu, J. Mater. Chem. B, 2015, 3, 8477–8485 RSC.
- N. J. Greenfield, Nat. Protoc., 2006, 1, 2876–2890 CrossRef CAS PubMed.
- C. K. Yip, T. G. Kimbrough, H. B. Felise, M. Vuckovic, N. A. Thomas, R. A. Pfuetzner, E. A. Frey, B. Brett Finlay, S. I. Miller and N. C. J. Strynadka, Nature, 2005, 435, 702–707 CrossRef CAS PubMed.
- A. M. Clemments, P. Botella and C. C. Landry, ACS Appl. Mater. Interfaces, 2015, 7, 21682–21689 CAS.
- N. Scholler, M. Crawford, A. Sato, C. W. Drescher, K. C. O'Briant, N. Kiviat, G. L. Anderson and N. Urban, Clin. Cancer Res., 2006, 12, 2117–2124 CrossRef CAS PubMed.
- L. Spinelli and N. R. Leslie, Methods, 2015, 77–78, 51–57 CrossRef CAS PubMed.
- E. Moncada, R. Quijada and J. Retuert, Nanotechnology, 2007, 18, 335606 CrossRef.
- A. Barth, Biochim. Biophys. Acta, Bioenerg., 2007, 1767, 1073–1101 CrossRef CAS PubMed.
- J. Kong and S. Yu, Acta Biochim. Biophys. Sin., 2007, 39, 549–559 CrossRef CAS PubMed.
- X. Huang and C. S. Brazel, J. Controlled Release, 2001, 73, 121–136 CrossRef CAS PubMed.
- P. Billsten, M. Wahlgren, T. Arnebrant, J. McGuire and H. Elwing, J. Colloid Interface Sci., 1995, 175, 77–82 CrossRef CAS.
- P. Satzer, F. Svec, G. Sekot and A. Jungbauer, Eng. Life Sci., 2015, 16, 238–246 CrossRef PubMed.
- Y.-H. Chen and J. T. Yang, Biochem. Biophys. Res. Commun., 1971, 44, 1285–1291 CrossRef CAS PubMed.
- S. B. Johnston and R. T. Raines, PLoS One, 2015, 10, e0116898 Search PubMed.
- D. Bolduc, M. Rahdar, B. Tu-Sekine, S. C. Sivakumaren, D. Raben, L. M. Amzel, P. Devreotes, S. B. Gabelli and P. Cole, eLife, 2013, 2, e00691 Search PubMed.
- K. Terpe, Appl. Microbiol. Biotechnol., 2003, 60, 523–533 CrossRef CAS PubMed.
- S. Harper and D. W. Speicher, Methods Mol. Biol., 2011, 681, 259–280 CAS.
- A. A. Vertegel, R. W. Siegel and J. S. Dordick, Langmuir, 2004, 20, 6800–6807 CrossRef CAS PubMed.
- J. E. Gagner, M. D. Lopez, J. S. Dordick and R. W. Siegel, Biomaterials, 2011, 32, 7241–7252 CrossRef CAS PubMed.
- H. Wu, S. Wang, D. Weng, H. Xing, X. Song, T. Zhu, X. Xia, Y. Weng, G. Xu, L. Meng, J. Zhou and D. Ma, Cancer Lett., 2008, 271, 205–214 CrossRef CAS PubMed.
- M. Tanaka and H. B. Grossman, Gene Ther., 2003, 10, 1636–1642 CrossRef CAS PubMed.
- D. Li, Y. Zhang, Y. Xie, J. Xiang, Y. Zhu and J. Yang, Cancer Gene Ther., 2013, 20, 251–259 CrossRef CAS PubMed.
- M. Tanaka, D. Koul, M. A. Davies, M. Liebert, P. A. Steck and H. B. Grossman, Oncogene, 2000, 19, 5406–5412 CrossRef CAS PubMed.
- L. C. Cantley and B. G. Neel, Proc. Natl. Acad. Sci. U. S. A., 1999, 96, 4240–4245 CrossRef CAS.
- S. Maddika, S. R. Ande, E. Wiechec, L. L. Hansen, S. Wesselborg and M. Los, J. Cell Sci., 2008, 121, 979–988 CrossRef CAS PubMed.
Footnote |
† Electronic supplementary information (ESI) available: Experimental details of cloning, protein purification and characterization of GST–PTEN, silica nanoparticle synthesis and immobilization techniques, results of cloning and protein induction, protein purification, western blot and Circular Dichroism (CD), FESEM of silica nanoparticles, binding percentage of GST–PTEN onto silica, zeta potential and FTIR spectra of free and GST–PTEN bound silica nanoparticles, monitoring shift at 222 nm by CD, phosphatase assay of GST protein, binding percentage of PTEN onto silica nanoparticles, gel image of expression study of PTEN on U 87-MG cells, MTT assay of GST–PTEN on U 87-MG cells. See DOI: 10.1039/c6ra23036a |
|
This journal is © The Royal Society of Chemistry 2016 |
Click here to see how this site uses Cookies. View our privacy policy here.