DOI:
10.1039/C6RA22267F
(Paper)
RSC Adv., 2016,
6, 108404-108410
Accurate description of hybridized local and charge-transfer excited-state in donor–acceptor molecules using density functional theory†
Received
6th September 2016
, Accepted 19th October 2016
First published on 19th October 2016
Abstract
Hybridized local and charge-transfer excited state (HLCT) is a mixed state between locally-excited (LE) state and charge-transfer (CT) state, which is very useful for the molecular design of new-generation organic electroluminescence materials. Accurate description of HLCT excited state is still challenging issue by means of conventional density functional theory (DFT). In this study, we used 2 local functionals (SVWN and PBE), 7 hybrid functionals (BLYP, B3LYP, PBE0, BMK, BHHLYP, M06-2X, M06HF), and one long-range-corrected functional ωB97X to calculate the molecular geometry and excited state property, using diffuse-containing basis sets and the Polarizable Continuum Model (PCM), respectively. The results showed that ωB97X was the most reliable functional for the accurate description of HLCT state at both ground state and excited state.
1. Introduction
For a donor–acceptor (D–A) molecule, there are three possibilities for its low-lying excited state (S1): “Local Emissive (LE)”,1 “Charge Transfer (CT)”,2,3 and “Hybridized Local and Charge-Transfer (HLCT)”.4–10 The LE excitation has a large oscillator-strength, which means that it is possible to detect this excited state spectroscopically. The CT state is a specific case state where the excited electron and hole have significant spatial separation. The oscillator strengths of the CT states are expected to be weak because the overlap of the orbitals which participate in the transition is relatively small. The HLCT state hybridizes the character of LE and CT.4,10 According to the state mixing principle in quantum chemistry,11 the state mixing can be described as a linear combination of two states of CT and LE. When LE and CT lie closely to each other, a state mixing may be triggered, thus it can be expected as a high-efficiency mixing or hybridized state forms, with both high fluorescence radiation and high exciton utilization.4,10 The electronic properties, geometric structures, and spectroscopic constants of molecules in excited electronic states represent fundamental quantities of mostly molecules, but the excited states are usually own a very short lifetime, thus their full experimental characterization is very difficult to detect. Therefore, all information that can be obtain by theory is particularly valuable not only for rationalize the experimentally observed properties of known materials but also for the design and predict those of new ones.
Density functional theory (DFT) offers the best compromise between accuracy and computational performance for typical chromophores of about 100 atoms, and numerous successes of this technique have been reviewed.11–19 Time-dependent density functional theory (TD-DFT) is most extensively used for the computing the structure and properties of excited electronic states at a reasonable computational cost. This method provides an improved treatment of the electron correlation effects relative to other methods like CIS. Despite its pervasiveness and versatility, the TD-DFT is limited and suffers an obvious drawback: the reliability of the results depends significantly on the selected exchange–correlation (xc) functional.20–27 However, the recent literature has shown that, when coupled with suitable functionals (especially hybrid and/or long-range corrected models), the TD-DFT approach provides very good results.28,29
In this work, we aim to ascertain the accuracy of the TD-DFT method which is applied to describe the HLCT state. For this reason, we have chosen a typical HLCT state molecule that has been extensively studied as the research object. Firstly, we compare the geometry of the molecule in the electronic ground state and excited state were optimized by different functionals. Secondly, we explore the optical response (absorption and emission spectrum) of the molecule in different solvents using different functionals. Next, the transition characters are computed aim to assess the performance of the functionals in predicting low-lying excited-state. An important issue of this process is the character of the excited states, in particular, whether the excitation results in a state mainly localized at the chromophore, i.e., a LE state, a CT state, or a HLCT state. Finally, since the cost of this method is relatively low, we are able to extend the study to moderately large systems that are closer to experimental conditions. A series of HLCT molecules are computed in order to establish the accuracy and proper strategies required in DFT for HLCT compounds, and how the new method simplifies the calculations and extends their possibilities. In these studies, an evaluation of the performance of different exchange-correlation density functionals on series of realistic chemical tasks is normally a necessary step prior to their use for the investigation of new problems.
2. Methodology and computational details
To assess the performances of DFT approaches, we tried to cover a broad and diverse selection of functionals. The benchmark comprises the pure functionals, the LDA functional and the generalized-gradient (GGA) approximations functionals SVWN30 and PBE,31 the hybrid functionals BLYP (0% HF),32 B3LYP (20% HF),33 PBE0 (25% HF),34 BMK (42% HF),35 BHHLYP (50% HF),36 M06-2X (56% HF),37 and M06HF (100% HF),38 and the long-range corrected functional ωB97X.39 In all these hybrid functionals, the fraction of Hartree–Fock (HF) exchange is a constant at each point in space, from 0% to 100%, respectively. By tuning the fraction of HF exchange we hope to gain insight into how the orbital dependence of the exchange function affects the description of electronic excited states. And the third strategy of the long-range corrected functional ωB97X which has been developed to separate the Coulomb operator 1/r into short- and long-range exchange component with the help to the standard error function. The short-range exchange is represented by semi local hybrid functional for the description of the short-range exchange interaction, and the long-range part is expressed with the exact Hartree–Fock (HF) exchange, which correct the long-range asymptotic behavior. The length scale for range separation is controlled by the ω parameter, but it is usually a single constant value. It is typically determined by fitting to a particular training set, and depends strongly on the electronic structure of the particular system. In addition, bulk solvent effects on the ground and the excited states have been taken into account by means of the Polarizable Continuum Model (PCM).40–42 In this paper we are unified with the most widely used basis sets 6-31+G(d,p) with the ground state and excited state calculations. All calculations have been performed with the Gaussian 09.D.01 revision.43
3. Results and discussions
Taking a typical HLCT molecule TPA-AC (Scheme 1)10 as an example, by discussing its geometric structures of ground and excited state, absorption and emission spectra, excited state properties and so on, to determine the suitable functional which describe the properties of the HLCT state most accurate.
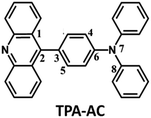 |
| Scheme 1 Representation of the HLCT compound TPA-AC investigated herein. | |
3.1 Effects of ground and excited state geometry
Firstly, we discuss the affect of functionals on two optimized geometrical structures: the ground state (S0) and the first excited states (S1). Frequency calculations were performed to verify if there was imaginary frequency with the lower energy structures or not, and the results demonstrated all the structures located the stationary points on their potential energy surfaces. The selected important bond lengths and dihedral angle between the donor and acceptor planes of TPA-AC in both the S0 and S1 states, which obtained with ten functionals SVWN, PBE, BLYP, B3LYP, PBE0, BMK, BHHLYP, M06-2X, M06HF, and ωB97X (the optimized ω of ground and excited state are list in ESI Part I†), are list in Table 1, separately. And the details of the optimized geometrical structures are given in the ESI Part II.†
Table 1 The important bond length and dihedral angle of TPA-AC obtained with ten functionals in the optimised ground- and excited-state geometries
Functionals |
Distance (Å) |
Dihedral angle (°) |
C2–C3 |
C1–C2–C3–C5 |
Ground-state |
Excited-state |
Ground-state |
Excited-state |
SVWN |
1.4892 |
1.4734 |
64.13 |
91.80 |
PBE |
1.4879 |
1.4751 |
64.19 |
60.81 |
BLYP |
1.4990 |
1.4844 |
65.30 |
92.88 |
B3LYP |
1.4914 |
1.4905 |
66.54 |
87.41 |
PBE0 |
1.4835 |
1.4751 |
64.21 |
60.80 |
BMK |
1.4960 |
1.4641 |
63.37 |
48.12 |
BHHLYP |
1.4857 |
1.4545 |
61.20 |
49.45 |
M062X |
1.4857 |
1.4511 |
68.66 |
46.97 |
M06HF |
1.4947 |
1.4496 |
63.26 |
46.76 |
ωB97X |
1.4953 |
1.4616 |
69.25 |
48.20 |
It can be seen from the Table 1 that different functionals have almost no effect on the geometries of the ground states. For example, the bonds length of C2–C3 have slight change less than 0.01 Å, the change of the dihedral angles C1–C2–C3–C5 are less than 8°. With respect to the excited-state geometries, the C2–C3 bonds are also change slightly, but the change of the dihedral angles C1–C2–C3–C5 are bigger, the biggest is about 45°. These results indicate that electronic excitation by different functionals lead to the large variations between the D and A derivatives, but the configurations in ground state is not sensitive by functionals.
The variations in the geometries from ground states to excited states are very regular by different functionals. For the molecule in the ground states, the bonds length of the C2–C3, ranging from 1.4835 to 1.4990 Å, are slightly regular. And in the excited states, the C2–C3 bonds are shorten by all functionals, indicating singlet exciton mainly locate on the C2–C3 bond. This point will be confirmed by the natural transition orbital (NTO) analysis in following section. On the contrary, the change of the C1–C2–C3–C5 dihedral angles from ground states to excited states result in different functionals are diverse, from about 65° to, about 45°, 60°, and 90° respectively. As shown in the Table 1, the dihedral angles are largen and almost orthogonal by employed the SVWN, BLYP and B3LYP functionals. The PBE and PBE0 functionals result in the dihedral angles slight change in excited state, whereas, from the BMK to M06HF, with increased the fraction of HF exchange, the change of the dihedral angles are decreased and gradually stabilized, and the ωB97X functional with the same trend. The dihedral angle was flattening from ground-state to excited-state, indicating an enhanced π conjugated degree along the backbone. The dihedral angle may have important influence on the excited state properties.
3.2 Absorption and emission properties
To see if the functional have effect on luminescent property, absorption and emission properties of the TPA-AC in different solvents were calculated. The electronic absorption and emission energies were computed by performing vertical S0 → S1 and S1 → S0 transitions from the ground and the excited state optimized geometries, respectively. For the absorption and emission energies, the solvent effects were included by using the PCM approach. It is worth to highlight that the maximum of an absorption or emission band does not necessarily coincide with the vertical energies, but it depends also on the Franck–Condon factors between the ground and the excited state minima and on the possible presence of vibronic interactions with close-lying excited states. However, since in this work were more focused on their production of the spectrum, solvatochromic shifts and the investigation of the functionals for the HLCT state, vibronic contribution to the absorption and emission spectra could be neglected. The performance of the different functionals for excited state properties were first discussed by means of absorption and emission spectra, values were calculated for the deviations of distinct transition character such as spectrum alternations from the experimental benchmark. Fig. 1 shows the difference in absorption and emission spectra of the DFT-calculated results and experiment. In this comparison, the SVWN, PBE, BLYP, B3LYP and PBE0 functionals obtained the poorer results. The performance of the functionals BMK and ωB97X are excellent in the calculation of the absorption spectra, and the functionals M062X and ωB97X closer the experimental results in comparison of the emission spectra, specific values are listed in Table S3.† Integrated the absorption and emission spectra, the ωB97X functional results are the most stable.
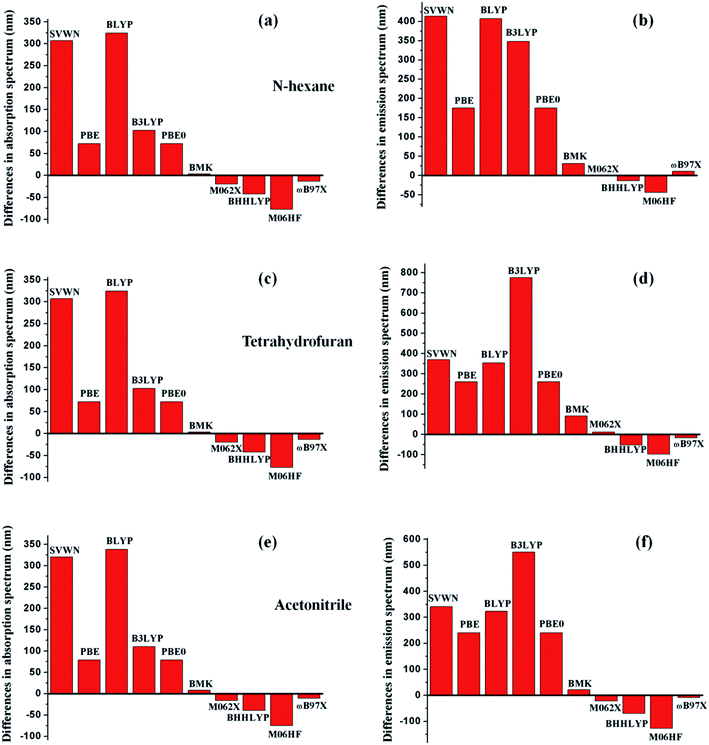 |
| Fig. 1 Difference in absorption and emission spectrum of TPA-AC in solvents with the experimental values employed different functionals. | |
3.3 Transition character
HLCT state is a special exited state which wave functions of D and A have a part of overlap, instead of absolute separation or overlap in pure CT or LE state. Owing to the large overlap from LE contribution, the HLCT transition becomes allowed and efficient, which is confirmed from the large oscillator strengths of the S1 state. Thus HLCT state is an effective pathway to obtain the high-efficiency CT character luminescence. As mentioned above, the TPA-AC is a typical HLCT molecule which has been demonstrated by the fluorescent solvatochromic experiment.5 Here, we will use the DFT approach to analyze its excited state transition properties, to detect which one functional can accurately describe this special excited state. In this section, we carried out the natural transition orbital (NTO) calculation for excited states of TPA-AC by different functionals. The dominant “Hole” → “Particle” contributions and the associated weights for the first excite (S0 → S1) of the TPA-AC were illustrated in Fig. 2.
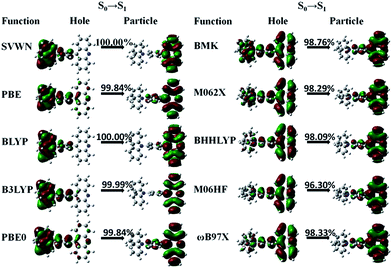 |
| Fig. 2 NTOs of S1 for TPA-AC by different functionals. | |
In the S1 states which determine the luminescence properties, the functionals SVWN, BLYP, and B3LYP display a complete CT transition character, it could be affect by the larger dihedral angle between D and A in the excited state. The NTO of the other functionals PBE and from the PBE0 to the M06HF with the increase the fraction of the HF, the S1 state exhibit the distinct HLCT feature, especially for the BMK and M062X exhibit the more complete state mixing. This can be understood from the fact that a DFT calculation using a functional with no or a small amount of HF exchange tends to make the electron distribution more localized in the excited state due to the self-interaction. In addition, the S1 state of the ωB97X functional exhibits the obvious HLCT character too.
It is also possible to calculate transition character with a polarizable solvent models, in this case, once the solute and solvent structure have been optimized for the initial state of the solute, each state energy and wave function is calculated with the same solvent structure, but where the solvent molecules' charges are replaced by gas-phase charges plus a molecular polarizability. In this work we used the Polarizable Continuum Model (PCM) model in all cases. The different transition energies calculated by functionals in solution are detailed in Table S3.† As shown in the Fig. 2, the HLCT transitions of the S1 state for the TPA-AC are described successfully by employed the BMK, BHHLYP, M06-2X, M06HF and ωB97X functionals. Therefore, in this part, only these functionals data will be compared with the experiment in solvents. The NTOs are illustrated in Fig. 3.
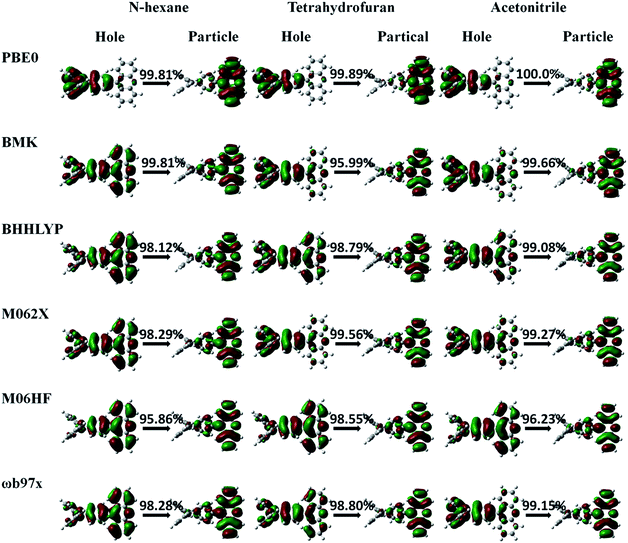 |
| Fig. 3 NTOs of S1 for TPA-AC in solvents by different functionals. | |
The solvatochromic experiment has been proved that the TPA-AC molecule exhibited large PL redshifts from low-polarity n-hexane to high-polarity acetonitrile, and according to the Lippert–Mataga equation, the dipole moment of the S1 state in the n-hexane was very large (μe = 14.0 D) which is attributed to the HLCT state, and the solvent more polar and the dipole moment more larger. As shown in the Fig. 3, it is found that the electronic cloud of “Hole” and “Particle” of all the studied functionals mainly distributes in the whole π-conjugated backbone and the central chromophore, respectively. In contrast to PBE0 and M06HF functionals, the smallest and biggest fraction of HF exchange of them, the electronic cloud display a complete CT and LE transition character in solvents and almost same as each other in the different solvent. On the other hand, the functionals exhibit the HLCT character, with the suitable fraction of HF exchange, include BMK, BHHLYP and M062X, but in the high polar solvents tetrahydrofuran and acetonitrile, the “Hole” and “Particle” were almost not change by BMK and M062X functionals, this indicate that these two functionals predicted failure in these high polar solvent. The electron cloud distribution shows the trend of a gradual change which employ the BHHLYP functional, with increasing solvent polarity, the electron distribution of S1 state from the HLCT state gradually turned into almost full CT state. It can be confirm the experiment results in the hybrid functionals. It is worth mentioning that the ωB97X functional is also reproduce the experimental results perfectly.
In above study, the ground-state and excited-state properties of the HLCT state D–A molecule TPA-AC was investigated by employing DFT and (PCM-) TD-DFT methods using the SVWN, PBE, BLYP, B3LYP, PBE0, BMK, BHHLYP, M06-2X, M06HF and ωB97X functionals in vacuum and solvent circumstances. Firstly, no significant differences are found in the ground state geometry optimizations by any functional. This indicated that the function have little impact on the ground state configurations. Then, when the SVWN, PBE, BLYP, B3LYP, PBE0, BMK, BHHLYP, M06-2X, M06HF and ωB97X functionals are included in the excited-state calculations, ωB97X were found to generate the transition band closest to the experimental result, for both absorption and emission spectra, followed by the M062X and BMK. The BHHLYP and M06HF functionals greatly overestimate the excitation energies and the SVWN, PBE, BLYP, B3LYP and PBE0 functionals underestimate them considerably. In addition, the BHHLYP and ωB97X functionals were descript the HLCT transition character accurately. From all these values, we suggests that TD-ωB97X functional in general will give good results for HLCT states. Of course, only one HLCT state molecule has been considered in the present investigation. Care should be taken before applying this functional to other types of structures.
3.4 Extensive applicability
In order to verify whether the ωB97X functional can accurate and comprehensive description the HLCT state, in the following sections, we will used this functional to calculated a series of HLCT state molecule. There are TPA-PA, TPA-AN, TPA-QAP, TPA-BZP and TPA-NZP, and their HLCT state characters were verified by fluorescent solvatochromic experiment, molecular structures are shown in Scheme 2.10
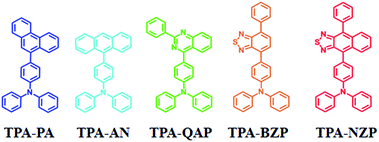 |
| Scheme 2 Representation of the HLCT compounds investigated herein. | |
In this part, the ground and excited state geometries structures and photophysical properties of these complexes are investigated by the ωB97X functional in combination with 6-31+G(d,p) basis sets. Table 2 list the calculated maximum absorption and emission spectra of this series HLCT molecules in vacuum and solvents, along with the experimental data. It is obvious that the calculated values are consistent with the experimental values, especially that absorption wavelength. For the emission band, the performance of the functional is less than the absorption; however, the differences between the calculated and experiment are small. From the above research we conclude that the ωB97X is an ideal functional for calculated the HLCT molecule.
Table 2 The absorption and emission spectrum of TPA-PA, TPA-AN, TPA-QAP, TPA-BZP and TPA-NZP in solvents, experimental values are in the parentheses
|
|
n-Hexane |
Tetrahydrofuran |
Acetonitrile |
TPA-PA |
Abs(nm) |
327.57(335) |
321.61(336) |
321.59(334) |
PL(nm) |
406.42(388) |
435.27(423) |
447.91(456) |
TPA-an |
Abs(nm) |
375.40(370) |
378.84(368) |
378.27(366) |
PL(nm) |
455.60(428) |
466.87(468) |
510.23(522) |
TPA-QAP |
Abs(nm) |
373.00(382) |
377.77(387) |
378.77(384) |
PL(nm) |
449.27(436) |
489.78(501) |
503.08(534) |
TPA-BZP |
Abs(nm) |
419.26(434) |
418.46(437) |
417.25(423) |
PL(nm) |
548.12(545) |
595.67(600) |
662.17(632) |
TPA-NZP |
Abs(nm) |
518.03(500) |
516.00(501) |
514.24(495) |
PL(nm) |
592.76(597) |
668.17(643) |
693.11(648) |
4. Conclusions
The description of hybridized local and charge-transfer excited state (HLCT) complexes is highly challenging for standard density functionals. Using a typical HLCT molecules TPA-AC, we benchmarked 2 local functionals (SVWN and PBE), 7 hybrid functionals (BLYP, B3LYP, PBE0, BMK, BHHLYP, M06-2X, M06HF), and one long-range-corrected functional ωB97X in the framework of the simulation of ground and excited state properties. Solvent effects have been carefully simulated in order to obtain theoretical best estimates that can be straightforwardly compared to the experimental absorption and emission spectra. For each functional, fully consistent calculations (all structures, absorption and emission spectra, and transition energies computed with the selected approach) have been achieved. The overall results suggest that the long-range corrected exchange-correlation functional (ωB97X) captures the characteristics of the HLCT excited states in a more rational way than the other functionals, the second are BMK and M06-2X. The SVWN, PBE, BLYP, B3LYP, PBE0 provide poorer results for the absorption and emission spectra. We further use a series of HLCT molecules successfully verified the ωB97X functional.
We emphasize that the study is conducted on a limited system and that further work is needed to find the optimal combination of functionals and basis sets for optimizations and excitations.
Acknowledgements
We are grateful for financial support from National Science Foundation of China (grant number 91233113, 51273078, 51473063, 51203091) and National Basic Research Program of China (973 Program grant number 2013CB834705, 2013CB834801, 2015CB655003).
References
- M. Pope and C. E. Swenberg, Electronic Processes in Organic Crystals and Polymers, Oxford University Press, Oxford, 1999 Search PubMed.
- Z. R. Grabowski, K. Rotkiewicz and W. Rettig, Chem. Rev., 2003, 103, 3899 CrossRef PubMed.
- A. D. Gorse and M. Pesquer, J. Phys. Chem., 1995, 99, 4039 CrossRef CAS.
- W. J. Li, D. D. Liu, F. Z. Shen, D. G. Ma, Z. M. Wang, Y. Xu, B. Yang, Y. G. Ma and T. Feng, Adv. Funct. Mater., 2012, 22, 2797 CrossRef CAS.
- W. J. Li, Y. Y. Pan, R. Xiao, Q. M. Peng, S. T. Zhang, D. G. Ma, F. Li, F. Z. Shen, Y. H. Wang, B. Yang and Y. G. Ma, Adv. Funct. Mater., 2014, 24, 1609 CrossRef CAS.
- S. Tang, W. J. Li, F. Z. Shen, D. D. Liu, B. Yang and Y. G. Ma, J. Mater. Chem., 2012, 22, 4401 RSC.
- S. T. Zhang, W. J. Li, L. Yao, Y. Y. Pan, B. Yang, Y. G. Ma, F. Shen and R. Xiao, Chem. Commun., 2013, 49, 11302 RSC.
- L. Yao, S. T. Zhang, R. Wang, W. J. Li, F. Z. Shen, B. Yang and Y. G. Ma, Angew. Chem., 2014, 126, 2151 CrossRef.
- Y. Y. Pan, W. J. Li, S. T. Zhang, L. Yao, C. Gu, H. Xu, B. Yang and Y. G. Ma, Adv. Opt. Mater., 2014, 2, 510 CrossRef CAS.
- W. J. Li, Y. Y. Pan, L. Yao, H. C. Liu, S. T. Zhang, C. Wang, F. Z. Shen, B. Yang, Y. G. Ma and P. Lu, Adv. Opt. Mater., 2014, 2, 892–901 CrossRef CAS.
- N. J. Turro, Modern Molecular Photochemistry, University Science Books, Sausalito, CA, 1991 Search PubMed.
- M. R. Silva-Junior, M. Schreiber, S. P. A. Sauer and W. J. Thiel, Chem. Phys., 2008, 129, 104103 Search PubMed.
- M. R. Silva-Junior, M. Schreiber, S. P. A. Sauer and W. J. Thiel, Chem. Phys., 2010, 133, 174318 Search PubMed.
- D. Jacquemin, V. Wathelet, E. A. Perpète and C. J. Adamo, J. Chem. Theory Comput., 2009, 5, 2420 CrossRef CAS PubMed.
- D. Jacquemin, E. A. Perpète, I. Ciofini, C. Adamo, R. Valero, Y. Zhao and D. G. J. Truhlar, Chem. Theory Comput., 2010, 6, 2071 CrossRef CAS PubMed.
- D. Jacquemin, E. A. Perpete, I. Ciofini and C. J. Adamo, J. Chem. Theory Comput., 2010, 6, 1532 CrossRef CAS PubMed.
- E. Runge and E. K. U. Gross, Phys. Rev. Lett., 1984, 52, 997 CrossRef CAS.
- M. E. Casida, in Time-Dependent Density-Functional Response Theory for Molecules, ed. D. P. Chong, World Scientific, Singapore, 1995, Recent Advances in Density Functional Methods, vol. 1, p. 155 Search PubMed.
- A. D. Laurent, C. Adamo and D. Jacquemin, Phys. Chem. Chem. Phys., 2014, 16, 14334 RSC.
- A. Dreuw and M. Head-Gordon, Chem. Rev., 2005, 105, 4009 CrossRef CAS PubMed.
- M. J. G. Peach, A. J. Cohen and D. J. Tozer, Phys. Chem. Chem. Phys., 2006, 8, 4543 RSC.
- Y. Zhao and D. G. Truhlar, J. Phys. Chem. A, 2006, 110, 13126 CrossRef CAS PubMed.
- D. Jacquemin, E. A. Perpète, O. A. Vydrov, G. E. Scuseria and C. Adamo, J. Chem. Phys., 2007, 127, 94102 CrossRef PubMed.
- M. Matsuura, H. Sato, W. Sotoyama, A. Takahashi and M. Sakurai, J. Mol. Struct.: THEOCHEM, 2008, 860, 119 CrossRef.
- D. Jacquemin, E. A. Perpète, G. E. Scuseria, I. Ciofini and C. Adamo, Chem. Phys. Lett., 2008, 465, 226 CrossRef CAS.
- M. J. G. Peach, P. Benfield, T. Helgaker and D. J. Tozer, J. Chem. Phys., 2008, 128, 44118 CrossRef PubMed.
- M. R. Silva-Junior, M. Schreiber, S. P. A. Sauer and W. Thiel, J. Chem. Phys., 2008, 129, 104103 CrossRef PubMed.
- D. Fan, Y. P. Yi, Z. D. Li, W. J. Liu, Q. Peng and Z. G. Shuai, J. Phys. Chem. A, 2015, 119, 5233 CrossRef CAS PubMed.
- Q. Peng, Y. L. Niu and Q. H. Shi, J. Chem. Theory Comput., 2013, 9, 1132 CrossRef CAS PubMed.
- S. H. Vosko, L. Wilk and M. Nusair, Can. J. Phys., 1980, 58, 1200 CrossRef CAS.
- J. P. Perdew, K. Burke and M. Ernzerhof, Phys. Rev. Lett., 1997, 78, 1396 CrossRef CAS.
- B. Miehlich, A. Savin, H. Stoll and H. Preuss, Chem. Phys. Lett., 1989, 157, 200–206 CrossRef CAS.
- A. D. Becke, J. Chem. Phys., 1993, 98, 5648 CrossRef CAS.
- C. Adamo and V. Barone, J. Chem. Phys., 1999, 110, 6158 CrossRef CAS.
- A. D. Boese and J. M. L. Martin, J. Chem. Phys., 2004, 121, 3405 CrossRef CAS PubMed.
- A. D. Becke, J. Chem. Phys., 1993, 98, 1372 CrossRef CAS.
- Y. Zhao and D. G. Truhlar, Theor. Chem. Acc., 2008, 120, 215 CrossRef CAS.
- Y. Zhao and D. G. Truhlar, J. Phys. Chem. A, 2006, 110, 13126 CrossRef CAS PubMed.
- J.-D. Chai and M. Head-Gordon, J. Chem. Phys., 2008, 128, 084106 CrossRef PubMed.
- J. Tomasi, B. Mennucci and R. Cammi, Chem. Rev., 2005, 105, 2999 CrossRef CAS PubMed.
- M. Cossi, G. Scalmani, N. Rega and V. Barone, J. Chem. Phys., 2002, 117, 43 CrossRef CAS.
- M. Cossi and V. Barone, J. Chem. Phys., 2000, 112, 2427 CrossRef CAS.
- M. J. Frisch, G. W. Trucks, H. B. Schlegel, G. E. Scuseria, M. A. Robb, J. R. Cheeseman, G. Scalmani, V. Barone, B. Mennucci, G. A. Petersson, H. Nakatsuji, M. Caricato, X. Li, H. P. Hratchian, A. F. Izmaylov, J. Bloino, G. Zheng, J. L. Sonnenberg, M. Hada, M. Ehara, K. Toyota, R. Fukuda, J. Hasegawa, M. Ishida, T. Nakajima, Y. Honda, O. Kitao, H. Nakai, T. Vreven, J. A. Montgomery Jr, J. E. Peralta, F. Ogliaro, M. Bearpark, J. J. Heyd, E. Brothers, K. N. Kudin, V. N. Staroverov, R. Kobayashi, J. Normand, K. Raghavachari, A. Rendell, J. C. Burant, S. S. Iyengar, J. Tomasi, M. Cossi, N. Rega, J. M. Millam, M. Klene, J. E. Knox, J. B. Cross, V. Bakken, C. Adamo, J. Jaramillo, R. Gomperts, R. E. Stratmann, O. Yazyev, A. J. Austin, R. Cammi, C. Pomelli, J. W. Ochterski, R. L. Martin, K. Morokuma, V. G. Zakrzewski, G. A. Voth, P. Salvador, J. J. Dannenberg, S. Dapprich, A. D. Daniels, Ö. Farkas, J. B. Foresman, J. V. Ortiz, J. Cioslowski and D. J. Fox, Gaussian 09, Revision D.1, Gaussian, Inc., Wallingford CT, 2009 Search PubMed.
Footnote |
† Electronic supplementary information (ESI) available: The optimised ω of TPA-AC in ground and excited state; the optimised geometries of ground and excited state employed different functionals; the excited-state properties of TPA-AC in different solvents employed different functionals. See DOI: 10.1039/c6ra22267f |
|
This journal is © The Royal Society of Chemistry 2016 |