DOI:
10.1039/C6RA21743E
(Paper)
RSC Adv., 2016,
6, 97194-97198
Reduced electrochemical performances of proton exchange membrane fuel cells due to gaseous diffusion in electrolytes
Received
30th August 2016
, Accepted 3rd October 2016
First published on 5th October 2016
Abstract
In this report, we derive a ‘gas leak mold’ model to investigate gas diffusion in the electrolyte membranes of proton exchange membrane fuel cells. The electrochemical parameters of proton exchange membrane fuel cells, including limiting current density and concentration polarization are studied systematically. In particular, we investigate the correlation between fuel cell performance and diffusion-induced gas leak of proton exchange membranes. This work is expected to facilitate the development of highly-efficient proton exchange membrane fuel cells.
1. Introduction
Among various hydrogen energy devices, fuel cells have gained extensive attention due to advantages including high conversion efficiency, low pollution and high fuel flexibility.1–3 Fuel cells include molten carbonate fuel cells (MCFCs), phosphoric acid fuel cells (PAFCs), solid oxide fuel cells (SOFCs), and proton exchange membrane fuel cells (PEMFCs).4 Owing to the characteristics of low operating temperatures, non-corrosion and high specific capacity, PEMFCs have drawn extensive research interests for portable electronics, electric vehicles (EV), and large-scale stationary power generation applications.5–8 In the operation of PEMFCs, the gas tightness of proton exchange membranes (PEM) is critical for gas isolation, electrical insulation, and proton conduction. In particular, serious performance degradation is induced by gas leak of porous PEMs.9–11 The authors have proposed a series of out-of-cell devices to measure directly and accurately the diffusivities of porous electrodes in SOFCs and lithium–air batteries.12–17 The work improves the understanding and efficient manipulation of gas diffusion in fuel cells. In this work, we investigate gas diffusion, and diffusion-induced performance degradation through quantitative derivation and analysis. In particular, we propose an electrochemical device to evaluate the diffusion-induced variations in limiting current density and concentration polarization in PEMFCs. Our study facilitates the out-of-cell evaluation of PEMs and is expected to improve the electrochemical performances of PEMFCs prior to intact cell assembly.
2. Theoretical analysis
2.1 Design of an out-of-cell gas diffusivity measurement device
We propose an electrochemical device to measure the gas diffusion in the electrolyte of a PEMFC, as shown in Fig. 1a. The device consists of a quartz tube with a dense PEM disc on one side and a PEM disc to be measured on the other side. The device is operated in a 80 °C furnace with the flow of H2 with 21% H2O. As the PEM sample to be measured leaks, H2 and H2O enter the tube from the furnace chamber. A current is applied across the left PEM disc as a H2 pump with Pt meshes on both sides of the dense PEM disc as catalyst. The flux of hydrogen (JH2) is expressed with current density via: |
 | (1) |
where F is the Faraday constant and i is the current density read with the H2 pump. As H2 is pumped into the tube, the concentration gradient of H2 is formed from inside tube to outside tube, and H2 diffusion occurs through the PEM sample. A voltage meter is attached across the dense PEM disc as a H2 sensor. The measured Nernst potential, E, as given by the H2 sensor, is correlated with the concentration of H2, as shown in eqn (2), |
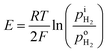 | (2) |
where
and
are the H2 partial pressures inside and outside the quartz tube, respectively. R is the gas constant, and T is the temperature.
is obtained based on eqn (3). At the steady state, the flux of H2 pumped into the tube and the flux of H2 diffusing outside the tube through the PEM sample are equal. Then the following correlation is obtained according to the Fick's law, |
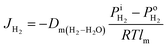 | (3) |
where Dm(H2–H2O) is the effective diffusivity of H2 and H2O in the PEM disc, and lm is the thickness of the PEM sample to be measured. At the steady state,
is correlated with Dm(H2–H2O) and i, according to eqn (1) and (3), as shown in eqn (4). |
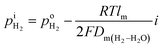 | (4) |
The PEM diffusivity Dm(H2–H2O) can be obtained from the slope of
∼i using eqn (4).
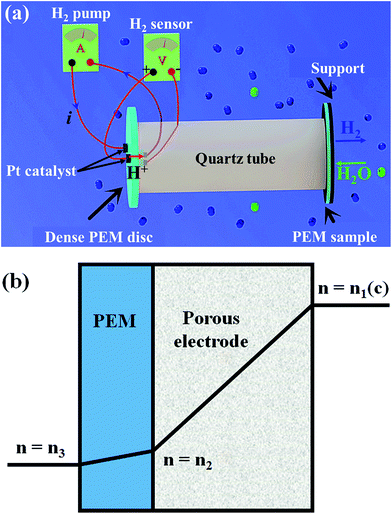 |
| Fig. 1 (a) Schematic of out-of-cell diffusivity measurement device: a current meter as gas pump and a voltage meter across a standard dense PEM disc, a quartz tube and an anode attached with a PEM sample to be measured. Blue dots and green dots represent H2 and H2O molecules. (b) The gas partial pressure variation across the porous electrode and the PEM sample with gas leak. | |
The O2 diffusivity through the PEM sample, Dm(O2–H2O), can also be measured with the electrochemical device placed in the furnace filled with O2 and H2O. A voltage is applied across the dense PEM to pump H+ into the tube, and H+ is formed from the hydrolysis of water on Pt catalyst outside the tube:
In the tube, H+ reacts with O2 to produce H2O. The reduction of O2 partial pressure inside the tube,
, leads to O2 diffusion across the PEM sample to be measured. At the steady state, the partial pressure of O2 inside the tube is derived similarly, as shown in eqn (6).
|
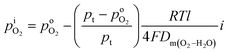 | (6) |
In the quartz tube,

≈ 1 −

and

is calculated from the
eqn (6).

is rather small, and can be obtained using the equilibrium constant (
Keq) based on
eqn (7).
Keq is given in
eqn (8).
|
2H2 (g) + O2 (g) = 2H2O (g)
| (7) |
|
 | (8) |
Then, the PEM diffusivity
Dm(O2–H2O) is obtained from the slope of

∼
i using
eqn (4).
2.2 Analysis of the influence of PEM leak diffusion on the performance of PEMFCs
The cathode limiting current density (ics) is the current density as the O2 partial pressure at the cathode/PEM interface,
, is zero, and given in eqn (9):4 |
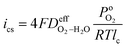 | (9) |
where
is the effective H2–H2O diffusion coefficient of the porous anode,
is the partial pressure of O2 outside the anode, and lc is the thickness of the anode. In the anode, H2/H2O diffuses to the anode/PEM interface in the porous anode. H2 molecules are converted into hydrogen protons, and incorporated into the PEM with water vapor for further diffusion towards the cathode. The limiting current density (ias) in a PEMFC is obtained,16 as shown in eqn (10), |
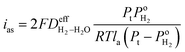 | (10) |
where
is the effective H2–H2O diffusion coefficient of the porous anode,
is the partial pressure of H2 outside the anode, Pt is the total pressure, and la is the thickness of the anode.
Based on the Nernst equation, the concentration polarizations (CPa for anode and CPc for cathode) are expressed in eqn (11) and (12).
|
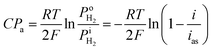 | (11) |
|
 | (12) |
For PEMFCs, the electrode thickness is typically in the range of 100–350 μm and the catalyst layer thickness is ∼10 μm.18–22 The catalyst layer is thin and the gas diffusion within the catalyst layer can be neglected. Then, we assume that the cell is full of saturated water vapor, and
is equal to
. Then, eqn (12) is simplified as:
|
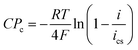 | (13) |
As presented in Fig. 1b, if the polymer electrolyte membrane is not gas tight, gas flux in the membrane is expected to induce extra concentration polarization. We assume that the flux in the PEM is J2 and the flux at the end of the electrode is J1. The gas species are then divided into two components, which are reaction flux and leakage flux. According to the reaction–flux correlation, the reaction mass is expressed as,
|
 | (14) |
where
J1 and
J2 are the fluxes in the cathode and the PEM, respectively,
n1 and
n2 are the gas concentrations outside and inside the electrode, respectively,
l and
l1 is the thicknesses of the electrode and the PEM, and
Dm is the diffusivity in the PEM. In
eqn (14), the mass of leaking gas is far below that in the cathode so that we assume
n3 = 0. The gas concentration outside the electrode is fixed since the fuel gas is steadily imported into the operating condition. The gas concentrations inside and outside the electrode can be expressed as,
|
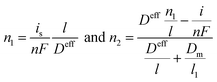 | (15) |
where
is is the limiting current density. Limiting current density is defined as the current density with zero oxygen concentration (
i.e.,
n2) inside the electrode. This condition corresponds to the reaction without gas leak. The concentration polarization in the anode can be written as,
|
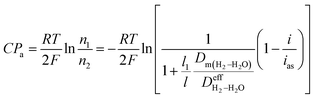 | (16) |
where
Dm(H2–H2O) is the hydrogen leaking diffusivity. The concentration polarization in the cathode combining the oxygen leaking diffusivity (
Dm(O2–H2O)) is expressed in
eqn (17).
|
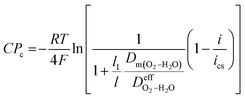 | (17) |
3. Results and discussion
With the assumption that the PEMs are ideally gas-tight membranes, plots of limiting current density versus electrode thickness (l) at different electrode diffusivities are given in Fig. 2a and b. ias and ics decrease rapidly first and then decrease gradually with increasing electrode thickness. With a fixed electrode thickness, ias and ics increase with increasing electrode diffusivity, indicating that a thin electrode provides a short diffusion path and more efficient diffusion. In addition, with fixed electrode diffusivities, cathodes can be made thinner compared with anodes when ias is equal to ics.
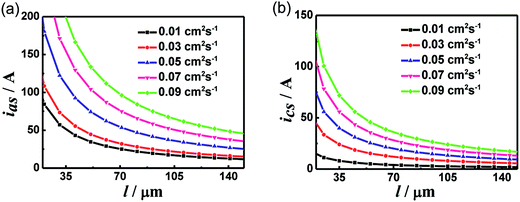 |
| Fig. 2 Plots of limiting current density versus the electrode thickness for anode (a) and cathode (b) with different electrode diffusivities, T = 353 K, Pt = 1 atm and PEM diffusivity = 0. | |
Fig. 3a and b show the CPa-versus-l and CPc-versus-l plots with different electrode diffusivities. For both anodes and cathodes, CP increases with increasing electrode thickness. CPa and CPc appear to be rather sensitive to diffusivity variation. With a fixed electrode thickness, CPa and CPc decrease with increasing electrode diffusivity, but the changes in CPa and CPc decrease substantially as the electrode diffusivity exceeds 0.05 cm2 s−1. This suggests that low CP is attainable by reducing the electrode thickness or increasing the electrode diffusivity. Comparing Fig. 3a with b, CPa increases more rapidly with increasing l compared to CPc as the diffusivity is set as 0.01 cm2 s−1 and the electrode thickness is above 100 μm. Once the diffusivity and electrode thickness exceed the critical values, diffusion becomes particularly inefficient. For the electrode with a large electrode diffusivity, the plots of CPa-versus-l are similar to that of CPc-versus-l. As a consequence, anodes are generally designed to be thicker compared with cathodes, leading to larger CPa compared with CPc. Such analytical results indicate that reducing the CPa is highly-favorable for reducing the overall cell polarization.
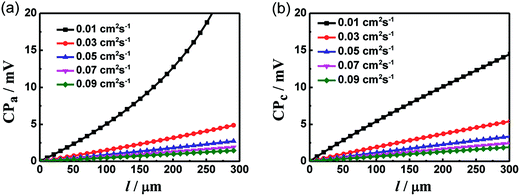 |
| Fig. 3 Plots of anode (a) and cathode (b) concentration polarization versus the electrode thickness with T = 353 K, Pt = 1 atm, i = 1 A cm−2, Dm = 0 and different diffusivities of electrode (Deff). | |
By introducing gas diffusion, gas leak in PEMs induces reduced performances of PEMFCs. As the PEM diffusivity is below the electrode diffusivity by a few orders, a logarithmic coordinate is employed in Fig. 4 for analysis. Intriguingly, as the fuel gas flux is consumed thoroughly in the reaction, the concentration inside the electrode (i.e., n2 in eqn (14)) decreases to zero. In the meanwhile, concentration polarization is correlated with the gas leak of the PEM. As shown in Fig. 4a and c, the polarization loss increases with increasing PEM diffusivity. The same conditions hold in Fig. 4b and d, which illustrates that the decrease of PEM thickness induces a high concentration polarization. A system with small Deff and large electrode thickness is sensitive to the gas permeability of the PEM. We then define a correction factor k:
|
 | (18) |
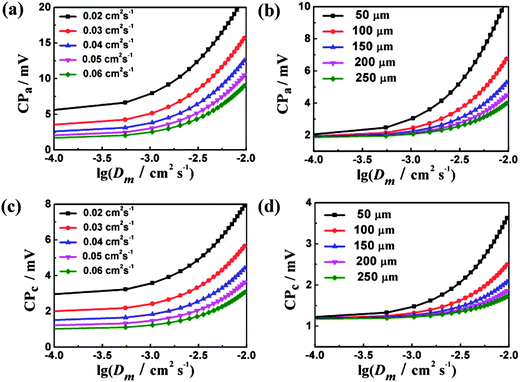 |
| Fig. 4 Plots of anode and cathode polarization versus lg(Dm (cm2 s−1)) with T = 353 K, Pt = 1 atm, l = 0.2 mm and i = 1 A cm−2 at different electrode diffusivity (a, c) and thickness of PEM (b, d). | |
The concentration polarization with gas leak is determined by k. As shown in Fig. 4b and d, the increasing dependence with the increase of PEM diffusivity is with fixed electrode diffusivity. The actual increasing feature is due to the increase of the ratio between PEM and electrode diffusivities. In particular, a thin electrode provides short gas diffusion channels to decrease the polarization loss while thick PEMs tend to limit gas leak. The concentration polarization increases with respect to increasing ratio of the two diffusivities, as shown in Fig. 4a and c. The overall contributions of small electrode thickness and large electrode diffusivity are desirable for reducing the influence of gas leak. In addition, an appropriate PEM thickness is required to balance ion conductivity and polarization. Due to the same correction factor employed in eqn (16) and (17), the dependences of cathode and anode CPs on Dm appear to be similar.
Indeed, PEM diffusivity on performance degradation is typically small. However, the influence of PEM diffusivity on the cell performance cannot be ignored as the PEM diffusivity exceeds the critical value, as shown in Fig. 4a–d.23–25 Below the critical value, CP varies subtly as Dm changes, and the gas leak induced by the leak of polymer electrolyte is negligible. Above the critical value, the concentration polarization increases rapidly with increasing lg(Dm). Once the PEM diffusivity exceeds the applied range, gas leak becomes pronounced. In addition, the critical diffusivity value is thickness and diffusivity dependent. As shown in Fig. 4, the critical values of Dm at different thicknesses vary substantially. For instance, with a fixed Deff of 0.03 cm2 s−1, the effective PEM diffusivity varies from 0 to ∼0.002 cm2 s−1 as the thickness changes from 5 mm to 50 μm.
In real-life applications of PEMFCs, the impact of gas leak in proton exchanging films on the performances of SOFCs changes as the electrode thickness, porosity, tortuosity, pore size, and operating conditions (temperature, moisture and output current) vary. Despite different focuses as found in our work and the experimental investigations in the literature, our derivations and calculations in this work are expected to provide useful insights into the efficient design and realization of electrolyte materials for high-performance PEMFCs.
4. Conclusion
In this paper, analytical equations associated with is and CP in PEMFCs were derived. According to the analysis, the critical diffusivity values of PEMs are obtained at cell operation conditions. Below the critical values, gas leak effects of PEMs on the performance of PEMFCs are negligible. In addition, the gas tightness of PEMs has been characterized by the diffusivity measured by a proposed out-of-cell electrochemical device. This work allows for the evaluation on the feasibility of PEMs before intact assembly and, thus, facilitates the development of highly-efficient PEMs.
Acknowledgements
The work was supported by the Fundamental Research Funds for the Chinese Central Universities (Grant No. ZYGX2014J088 and Grant No. ZYGX2015Z003), the National Natural Science Foundation of China (Grant No. 21403031) and the Science & Technology Support Funds of Sichuan Province (Grand No. 2016GZ0151).
References
- N. Shaigana, W. Qua, D. G. Iveyb and W. C. Ivey, J. Power Sources, 2010, 195, 1529 CrossRef.
- E. Antolini, Appl. Energy, 2011, 88, 4274 CrossRef CAS.
- T. Elmer and S. B. Riffat, Progress in Sustainable Energy Technologies, ed. I. Dincer, A. Midilli and H. Kucuk, Springer International Publishing, Switzerland, 2014, vol. II, pp. 247–271 Search PubMed.
- R. O'Hayre, S. W. Cha, W. Colella and F. B. Prinz, Fuel Cell Fundamentals, John Wiley & Sons, Weinheim, 2006 Search PubMed.
- A. J. Appleby, Fuel Cells: Trends in Research and Application, Hemisphere Publishing Corp, Washington DC, 1987 Search PubMed.
- D. Linden, Handbook of Batteries and Fuel Cells, McGraw-Hill, New York, 1984 Search PubMed.
- J. W. Lim and D. G. Lee, Int. J. Hydrogen Energy, 2012, 37, 12504 CrossRef CAS.
- B. G. Kim, J. W. Lim and D. G. Lee, Int. J. Hydrogen Energy, 2011, 36, 3087 CrossRef CAS.
- Y. Sugawara, H. Gyoten and M. Uchida, US Pat. 6818339, 2004.
- S. Fujii, N. Hase and K. Hatoh, US Pat. 7318974, 2008.
- A. Geiger, Characterization and development of direct methanol fuel cells, Swiss Federal Institute of Technology Zurich, 2002 Search PubMed.
- W. He, K. J. Yoon, R. S. Eriksen, S. Gopanlan, S. N. Basu and U. B. Pal, J. Power Sources, 2010, 195, 532 CrossRef CAS.
- L. Ye, W. Lv and Y. Mao, Int. J. Hydrogen Energy, 2014, 39, 15057 CrossRef CAS.
- W. He, B. Wang, H. Zhao and Y. Jiao, J. Power Sources, 2011, 196, 9985 CrossRef CAS.
- W. He and B. Wang, Adv. Energy Mater., 2012, 2, 329 CrossRef CAS.
- F. Zhao, T. J. Armstrong and A. V. Virkar, J. Electrochem. Soc., 2003, 150, A249 CrossRef CAS.
- W. He, X. Wang and L. Ye, ChemElectroChem, 2014, 1, 2032 CrossRef.
- B. R. Sivertsen and N. Djilali, J. Power Sources, 2005, 141, 65 CrossRef CAS.
- L. Wang, A. Husar, T. Zhou and H. Liu, Int. J. Hydrogen Energy, 2003, 28, 1263 CrossRef CAS.
- C. H. Hung, C. H. Chiu, S. P. Wang, I. Chiang and H. Yang, Int. J. Hydrogen Energy, 2012, 37, 12805 CrossRef CAS.
- C. Y. Jung, T. H. Kim and S. C. Yi, ChemSusChem, 2014, 7, 466 CrossRef CAS PubMed.
- S. P. Jiang, Int. J. Hydrogen Energy, 2012, 37, 449 CrossRef CAS.
- F. A. De Bruijn, V. A. T. Dam and G. J. M. Janssen, Fuel Cells, 2008, 8, 3 CrossRef CAS.
- W. Liu, K. Ruth and G. Rusch, J. New Mater. Electrochem. Syst., 2001, 4, 227 CAS.
- R. Borup, J. Meyers, B. Pivovar, Y. S. Kim, R. Mukundan, N. Garland and N. Iwashita, Chem. Rev., 2007, 107, 3904 CrossRef CAS PubMed.
Footnote |
† These authors contributed equally to this work. |
|
This journal is © The Royal Society of Chemistry 2016 |