DOI:
10.1039/C6RA21466E
(Paper)
RSC Adv., 2016,
6, 99139-99148
Synthesis, crystal growth and characterization of di-valine maleic – a new nonlinear optical material†
Received
26th August 2016
, Accepted 5th October 2016
First published on 5th October 2016
Abstract
An amino acid salt di-valine maleic (VM) has been synthesized for the first time and single crystals were grown by the controlled evaporation of the aqueous solution at constant temperature (48 ± 0.03) °C. Crystal and molecular structure of VM was determined by single crystal X-ray diffraction analysis. X-ray crystallography reveals that VM possesses different two-dimensional supramolecular networks through hydrogen bonding interactions. Investigation of intermolecular interactions and crystal packing via Hirshfeld surface analysis clearly quantify the interactions within the crystal structure. The physicochemical properties of the grown crystal were characterized by thermal and optical analysis and measurement of hardness. The second harmonic generation efficiency of VM was investigated by powder method and found to be 0.3 times to KDP. The dipole moment, polarizability, first order hyperpolarizability and the HOMO–LUMO energy gap of VM were calculated using B3LYP functional and 6-31G(d,p) basis set to confirm the suitability of the crystal for nonlinear optical applications. First hyperpolarizability was found to be 5 times of urea.
Introduction
Among all nonlinear optical (NLO) materials, organic materials are considered to be more versatile for their potentially high nonlinearities and rapid response compared with inorganic compounds. Other advantages of organic compounds involve amenability for synthesis, multifunctional substitutions, higher resistance to optical damage and maneuverability for device applications, etc. In the organic NLO class, amino acids are naturally chiral species and often crystallize in non-centrosymmetric space groups. Those materials have particular features, such as weak van der Waals and hydrogen bonds, wide transparency ranges in the visible regions and zwitterionic nature of the molecules.1,2
L-Valine is a branched chain amino acid, which has aliphatic nonpolar side chain. It crystallizes in the monoclinic space group P21 with lattice constants a = 9.682(2) Å, b = 5.247(1) Å, c = 11.930(2) Å and β = 90.57°.3 Although the structures of several compounds of organic and inorganic acids combined with L-valine have been reported,3–11 yet to best of our knowledge, very few of them have been reported as NLO materials. Attempts to discover such NLO crystals with relatively large nonlinearity are under progress in our laboratory. Hence, di-valine maleic was successfully synthesized and crystals with relative large size were grown. In the present paper we have discussed the growth of single crystal of di-valine maleic. The cell parameters and crystal structure of di-valine maleic was confirmed by the single-crystal X-ray diffraction analysis and indices of different crystallographic planes of the grown crystals were also determined. This was followed by DTA-TGA analysis, optical transmittance study and second harmonic generation (SHG) efficiency test.
Experimental
Synthesis and crystal growth
To synthesize di-valine maleic (VM), L-valine and maleic acid were added in a stoichiometric ratio of 1
:
1 to a beaker containing double distilled water. All the starting materials were of analytic reagent grade. The resulting mixture is continuously heated and stirred to form a homogeneous solution. At the point when a clear solution is obtained the pH of the solution was measured with a pH meter (Digital μpH METER GOLD 533) and it was found to be 1.02 at temperature 50 °C. The reaction equation is shown below.
2C5H11NO2 + C4H4O4 → 2C5H11NO2·C4H4O4 |
The clear solution was stirred with a magnetic stirrer for 5–6 h, filtered and left for crystallization. Crystalline materials of VM were obtained in 6–7 weeks time. The purity of the VM salt was improved by repeated cycles of dissolution and re-crystallization and confirmed by measuring the melting point at each stage.
For bulk crystal growth we first determined the solubility of VM in water in the temperature range 35–65 °C. For determination of solubility say at 60 °C, the temperature of the solution was kept above 60 °C accompanied with continuous stirring using a motorized magnetic stirrer to ensure homogeneous temperature and concentration throughout the entire volume of the solution. The saturated solution was allowed to reach equilibrium in 24 h at 60 °C in a temperature-controlled water bath and solubility of VM at 60 °C was determined gravimetrically. The same procedure was repeated for other different temperatures. The solubility curve thus obtained is shown in Fig. 1. From the solubility graph we observed that though the solubility temperature coefficient is positive yet its value is very low, hence slow evaporation of solvent is the best technique for the growth of good quality bulk crystal of VM. Accordingly bulk single crystals of VM of size 10 × 3.5 × 1.2 mm3, were grown from its aqueous solution by solvent evaporation method at constant temperature 48 °C using a microprocessor controlled constant temperature bath stabilized with an accuracy of ±0.03 °C. The photograph of the as-grown single crystals of VM is shown in the Fig. 2.
 |
| Fig. 1 Solubility curve of divaline maleic in water. | |
 |
| Fig. 2 As grown crystal of divaline maleic. | |
Characterization
Chemical composition of VM was determined by CHN analysis using 2400 Series II (PERKIN ELMER) CHN analyzer. The crystal and molecular structure of VM was determined by single crystal X-ray diffraction analysis. The X-ray data was collected at temperature 293(2) K using a Bruker APEX-II CCD diffractometer with graphite monochromatic MoKα radiation (λ = 0.7107 Å). Data reduction was carried out using the program Bruker SAINT.12 An empirical absorption correction SADABS13 was applied. The structure of the title complex was solved by direct method and refined by the full-matrix least-square technique on F2 with anisotropic thermal parameters to describe the thermal motions of all non-hydrogen atoms using the programs SHELXS97 and SHELXL97
14 respectively. All calculations were carried out using PLATON15 and WinGX system Ver-1.64.16 All hydrogen atoms were located from difference Fourier map and refined isotropically. CCDC 947795 contains the supplementary crystallographic data for this paper.† Molecular Hirshfeld surface17–22 of VM crystal structure has been created based on the electron distribution which has been calculated as the sum of spherical atom electron densities.23,24 The Hirshfeld surface is unique,25 for a particular crystal structure and set of spherical atomic electron densities. For each point on that isosurface, two distances are defined: de, the distance from the point to the nearest nucleus external to the surface, and di the distance to the nearest nucleus internal to the surface. The normalized contact distance (dnorm) based on both de and di, and the van der Waals (vdw) radii of the atom, given by the eqn (1) enables identification of the regions of particular importance to intermolecular interactions.17 The combination of de and di in the form of a 2D fingerprint plot26–29 provides summary of intermolecular contacts in the crystal.17 The Hirshfeld surfaces are mapped with dnorm, and 2D fingerprint plots were generated using Crystal Explorer 3.0.30 |
 | (1) |
Thermogravimetric (TG) and differential thermal analysis (DTA) were carried out on SDTQ 600 V8.2 instrument with a heating rate of 5 °C min−1 from 27 to 800 °C in a nitrogen atmosphere using VM as sample and alumina as reference. Using a polished crystal sample with the thickness of 1.2 mm, the optical transmission spectrum of the VM single crystal was recorded on the VARIAN, CARY 5000 spectrophotometer in the wavelength range from 200 to 2000 nm. The SHG measurements were performed using a Nd-YAG laser with fundamental radiation of 1064 nm as the optical source. Mechanical property of VM was analyzed by measuring hardness on its prominent growth facet. In our study indentations were made on (200) planes of VM with a Vicker indenter attached to a Leco microhardness tester (LM700). Loads were varied from 1 to 15 g with a regular increment of 5 g. Time of indentation is 10 s in all cases. Microhardness value was calculated using the formula given by eqn (2)
|
 | (2) |
where
Hv is the Vicker's hardness number in pascal,
P is the applied load in newton and
d is the diagonal length of the indented impression in meter. Owing to the observed micro cracks at the corners of the impressions made on the crystals, the maximum load applied was 15 g.
Theoretical and computational details
Quantum chemical calculations are precious and inexpensive tools for predicting the molecular NLO properties of materials such as dipole moments, polarizability and hyperpolarizabilities. In a medium the nonlinear optical effects arise when the medium interact with the electromagnetic field of intense laser beam. For an isolated molecule this nonlinear response can be represented by a Taylor series expansion of the dipole moment μi induced by the electric field31 |
 | (3) |
where α is the linear polarizability, μ0 the static dipole moment and β is the first order hyperpolarizability. Here i, j, k denotes either of the x, y, z axes which are orthogonal. The component of the first order hyperpolarizability can be determined using the relation, |
 | (4) |
In presence on an applied field the first hyperpolarizability is a third rank tensor that can be described by a 3 × 3 × 3 matrix. The 27 components of the 3D matrix can be reduced to 10 components due to Kleinman symmetry.32 Using the x, y, z components the total static dipole moment, μ, the mean dipole polarizability (α), the anisotropy of polarizability (Δα) and the mean first static hyperpolarizability (βtot) are defined as
αtot = (αxx + αyy + αzz)/3 |
We performed the calculations of the dipole moment, polarizability, anisotropy of polarizability and first hyperpolarizability using the Gaussian 09W program package.33 All the components of Gaussian outputs are reported in atomic units so the calculated values have to be converted into electrostatic units. Molecular geometry optimization was completed by B3LYP functional and 6-31G(d,p) basis set. The optimized molecular geometry represents an isolated molecule with stationary points in the potential surface; no imaginary frequencies were obtained which confirms the convergence. The first hyperpolarizability and the related properties of VM molecule were calculated using the same basis set, based on finite field approach. We obtained a plot of the frontier molecular orbitals of the title compound to analyze the main atomic contributions for these orbitals. The importance of observing these plots was to determine the chemical reactivity of VM. The frontier orbitals (HOMO and LUMO) determine the way in which the molecule interacts with other species. The HOMO is the orbital that primarily acts as an electron donor and the LUMO is the orbital that largely acts as the electron acceptor.34 The energy gap between these orbital are effectively responsible for the chemical and spectroscopic properties of the molecule.35
Results and discussions
Identification
The chemical composition of VM as predicted by the CHN analysis matches well with the theoretically determined percentage composition and hence the formation of the crystal in single phase is confirmed and the molecular formula is henceforth calculated to be C14H26N2O8 (2C5H11NO2·C4H4O4). Finally the chemical composition and structural formula of VM was determined from the results of single crystal X-ray diffraction analysis. It is observed that the material crystallizes in monoclinic, space group C2, which is recognized as noncentrosymmetric system, thus satisfying one of the most important criteria for a crystal to be second order nonlinear. A summary of crystal data and relevant refinement parameters are given in Table 1.
Table 1 Crystal data and structure refinement for VM
Empirical formula |
C7H13N1O4 |
Formula weight |
175.18 |
Temperature |
293(2) K |
Wavelength |
0.71073 Å |
Crystal system, space group |
Monoclinic, C2 |
Unit cell dimensions |
a = 22.287(8) Å; α = 90 deg. |
b = 7.310(2) Å; β = 91.683(12) deg. |
c = 5.3371(17) Å; γ = 90 deg. |
Volume |
869.1(5) Å3 |
Z, calculated density |
4, 1.339 Mg m−3 |
Absorption coefficient |
0.110 mm−1 |
F(000) |
376 |
Crystal size |
0.21 × 0.15 × 0.11 mm |
θ range for data collection |
1.83 to 24.99° |
Limiting indices |
−26 ≤ h ≤ 26, −8 ≤ k ≤ 8, −6 ≤ l ≤ 6 |
Reflections collected/unique |
4058/1521 [R(int) = 0.0468] |
Completeness to θ |
100.0% |
Absorption correction |
Semi-empirical from equivalents |
Max. and min. transmission |
0.99 and 0.98 |
Refinement method |
Full-matrix least-squares on F2 |
Data/parameters |
1521/114 |
Goodness-of-fit on F2 |
1.068 |
Final R indices [I > 2σ(I)] |
R1 = 0.0314, wR2 = 0.0787 |
R indices (all data) |
R1 = 0.0319, wR2 = 0.0792 |
Extinction coefficient |
0.104(6) |
Largest diff. peak and hole |
0.177 and −0.122 e Å−3 |
Structural description
The molecular view36 of the asymmetric unit of VM is shown in Fig. 3. Structural study of VM reveals that the C7 atom of the maleic acid molecule is located in an inversion centre (−x + 2, y, −z + 1), however the valine conformer consists of one independent molecule in the asymmetric unit. The bond-lengths and angles are in normal range and are given in Table 2.
 |
| Fig. 3 ORTEP view and atom numbering scheme of VM with displacement ellipsoids at 30% probability level. Hydrogen atoms are shown as small spheres of arbitrary radii. Symmetry transformations used to generate equivalent atoms: −x + 2, y, −z + 1. | |
Table 2 Bond lengths [Å] and angles [°] for VMa
Bond lengths [Å] |
Bond angles [°] |
Symmetry transformations used to generate equivalent atoms: #1 (−x + 2, y, −z + 1). |
O(2)–C(1) |
1.259(2) |
O(4)–C(6)–O(3) |
124.48(16) |
O(1)–C(1) |
1.245(2) |
O(4)–C(6)–C(7) |
119.97(15) |
O(3)–C(6) |
1.299(2) |
O(3)–C(6)–C(7) |
115.52(14) |
O(4)–C(6) |
1.217(2) |
C(7)#1–C(7)–C(6) |
123.02(19) |
C(6)–C(7) |
1.481(2) |
N(1)–C(2)–C(1) |
109.27(12) |
C(7)–C(7)#1 |
1.319(3) |
N(1)–C(2)–C(3) |
113.46(13) |
N(1)–C(2) |
1.500(2) |
C(1)–C(2)–C(3) |
111.28(14) |
C(2)–C(1) |
1.526(2) |
O(1)–C(1)–O(2) |
126.75(15) |
C(2)–C(3) |
1.540(2) |
O(1)–C(1)–C(2) |
116.10(13) |
C(3)–C(4) |
1.512(3) |
O(2)–C(1)–C(2) |
117.13(13) |
C(3)–C(5) |
1.516(3) |
C(4)–C(3)–C(5) |
111.66(19) |
|
|
C(4)–C(3)–C(2) |
113.68(14) |
|
|
C(5)–C(3)–C(2) |
112.03(17) |
The solid-state structure of VM is stabilized through N–H⋯O, O–H⋯O and weak C–H⋯O hydrogen bonding interactions (Table 3). Since the classical hydrogen bonds dominate the crystal packing, the VM structure allows us to distinguish between the effects of each type of interactions by which we can generate the individual substructures. In the first substructure, the C7 atom of the maleic acid in the molecule at (x, y, z) acts as donor to the carboxylate carbonyl oxygen atom O4 in the molecule at (2 − x, y, 2 − z) to generate a centrosymmetric R22(8) dimeric ring motif (homo synthon) centered at (1, 0, 1) (Fig. 4).
Table 3 Hydrogen bonding geometry of VM (Å, °)
D–H⋯A |
d(D–H) |
d(H⋯A) |
d(D⋯A) |
D–H⋯A |
Symmetry |
N(1)–H(1A)⋯O(1) |
0.89 |
2.14 |
3.022(2) |
168 |
x, y, −1 + z |
N(1)–H(1B)⋯O(4) |
0.89 |
1.98 |
2.853(2) |
167 |
−1 + x, −1 + y, −1 + z |
N(1)–H(1C)⋯O(1) |
0.89 |
1.95 |
2.814(2) |
163 |
1/2 − x, −1/2 + y, 1 − z |
O(3)–H(3)⋯O(2) |
0.82 |
1.71 |
2.522(2) |
172 |
3/2 − x, 1/2 + y, 1 − z |
C(5)–H(5C)⋯O(4) |
0.96 |
2.56 |
3.456(4) |
155 |
−1 + x, −1 + y, −1 + z |
C(7)–H(7)⋯O(4) |
0.93 |
2.57 |
3.448(2) |
158 |
2 − x, y, 2 − z |
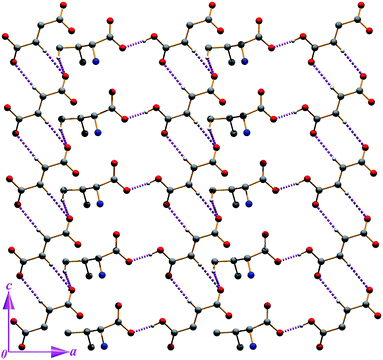 |
| Fig. 4 Formation 2D assembly through weak C–H⋯O hydrogen bonds in VM. | |
Since the C7 atom is located in the inversion centre, on either side of the conformer generates dimeric ring motif propagating along (0 0 1) direction (Fig. 4). Interestingly, valine molecule plays an important role in binding the parallel layers of the dimeric ring motifs of maleic acid conformer. The methyl carbon C5 of the valine conformer in the molecule at (x, y, z) acts as donor to the carbonyl oxygen O4 of maleic acid in the molecule at (−1 + x, −1 + y, −1 + z) possess a weak hydrogen bonding interaction. In another end of the valine molecule, the carboxylate oxygen atom O2 of valine molecule acts as an acceptor to the carboxylate oxygen O3 of maleic acid at (3/2 − x, 1/2 + y, 1 − z), thus connecting the parallel layers of dimeric maleic acid conformers. Hence at each junction of the layers, valine molecules connect them by forming R65(28) ring motifs and propagates in (1 0 1) plane (Fig. 4).
In another substructure, the amino nitrogen atom N1 at (x, y, z) acts as donor to the carboxylate oxygen atom O1 in the molecule at (x, y, −1 + z) and this oxygen atom acts as acceptor to the N1 atom of the partner molecule through symmetry operation (1/2 − x, −1/2 + y, 1 − z), thus a tetrameric R44(14) ring motif is generated among the valine conformers of the structure. Repetition of this tetrameric ring motif builds a supramolecular network structure in (0 1 1) plane which is generated only through N–H⋯O hydrogen bonding interactions (Fig. 5).
 |
| Fig. 5 2D supramolecular network structure in (0 1 1) plane generated through N–H⋯O hydrogen bonding interactions. | |
In another substructure (Fig. 6), the amine N1 atom of valine molecule interacts with its carboxylate oxygen atom O1 along with the carbonyl oxygen atom O4 of the partner conformer. The amine nitrogen N1 at (x, y, z) acts as donor both to the carboxylate oxygen at (1/2 − x, −1/2 + y, 1 − z) and also to the carbonyl oxygen O4 of partner conformer at (−1 + x, −1 + y, −1 + z) through dual N–H⋯O hydrogen bonds. Thus a R88(38) ring motif is generated and repetition of this ring motif lead the molecules to propagate along (1 1 0) plane (Fig. 6).
 |
| Fig. 6 Another 2D supramolecular network structure in (1 1 0) plane generated through N–H⋯O hydrogen bonding interactions. | |
Hirshfeld surface
The X-ray crystallographic analysis reveals that the asymmetric unit of VM comprised of valine and maleic acid conformers. The Hirshfeld surfaces17–22 of the VM structure was analyzed to clarify the nature of the intermolecular interactions and is illustrated in Fig. 7 showing the surfaces that have been mapped over a dnorm. During Hirshfeld surface calculation, the valine and maleic acid conformers have been analyzed individually to discern different types of intermolecular interactions and to obtain quantitative insight into the intermolecular interactions in molecular solids.
 |
| Fig. 7 Hirshfeld surface mapped with dnorm for VM. | |
For maleic acid conformer, the symmetrically generated counterpart has not been considered in the dnorm surface calculation. The Hirshfeld surfaces have been mapped over dnorm (−0.83 to 1.38 Å) and (−1.22 to 1.33 Å) for valine and maleic acid conformers respectively (Fig. 7). The surfaces are shown as transparent to allow visualization of the molecular moiety around which they were calculated. The dnorm surfaces reveal the close contacts of hydrogen bond donors and acceptors, but other close contacts are also evident. In dnorm surfaces, the large circular depressions (deep red) are the indicators of hydrogen bonding contacts whereas other visible spots are due to H⋯H contacts. The dominant O–H⋯O intermolecular interactions appear as distinct spikes in the 2D fingerprint plots26–29 (Fig. 8) of the valine conformer in the region 1.53 Å < (de + di) < 1.62 Å whereas the spikes appearing in the region 1.53 Å < (de + di) < 1.86 Å belong to maleic acid conformer. The O⋯H contributions have the larger variety (21.1% in valine and 41.9% in maleic acid) than its H⋯O counterparts (28.0% in valine and 14.0% in maleic acid) for each molecule of the conformers. The proportions of O⋯H/H⋯O interactions comprising 49.1%, and 55.8% of the total Hirshfeld surface area for each molecule of valine and maleic acid conformer respectively (Fig. 8).
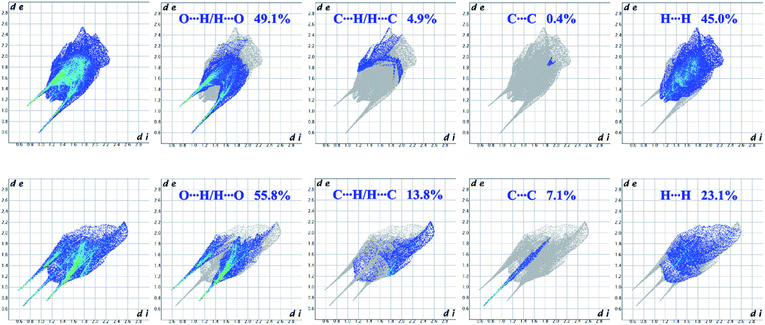 |
| Fig. 8 Fingerprint plots of VM: full (left) and resolved into various contacts showing the percentages of contacts contributed to the total Hirshfeld surface of molecules. | |
In the title structure, no significant C–H interaction has been observed with C–H close contacts varying from 4.9% (valine) to 13.8% (maleic acid). Since the C7 atom of the maleic acid conformer is located in an inversion centre, the C⋯C close contact comprise 7.1% of the total Hirshfeld surface area of the molecule whereas the valine molecule comprises only 0.4% of the total Hirshfeld surface area. A significant difference between the molecular interactions in valine and maleic acid conformer in terms of H⋯H interactions is reflected in the distribution of scattered points in the fingerprint plots, which comprises 45.0% and 23.1% in valine and maleic acid conformers respectively.
Thermal properties
The TGA thermogram of the grown crystal is shown in Fig. 9. From TGA graph we see that the initial decomposition of the compound starts at 137.53 °C. Since this temperature is beyond 100 °C, the chance of existence of water of crystallization or physically absorbed water in VM crystal is ruled out. The endothermic peak around 231.41 °C corresponds to the melting point of VM. Sharpness of the peak indicates the good crystallinity of the material.37 The sharp slope of the TG curve up to melting point indicates that the compound decomposes completely on melting. The DTA-TGA analysis does not show any kind of phase transition of VM crystal.
 |
| Fig. 9 TGA-DTA thermogram of divaline maleic. | |
Optical properties
Transmittance studies. The observed UV-vis-NIR spectrum obtained from a transparent and colorless crystal of thickness 1.2 mm of di-valine maleic (VM) is shown in Fig. 10. The transmittance lies in the range of 55–65% over the entire range of transmission (320–1860 nm). Thus both the fundamental and the second harmonic wavelength of the Nd-YAG laser can easily transmit through the crystal. The lower cut-off wavelength for the crystal is 279.9 nm.
 |
| Fig. 10 Transmittance spectrum of divaline maleic crystal. Inset: Tauc's plot. | |
To obtain information about the optical band gap (Eg) of our grown crystal, the absorption coefficient (α) is first obtained from the transmission spectrum as described by the following equation
|
 | (5) |
where
t is the thickness and
T the transmittance of the sample. These absorption coefficient values were used to determine the optical energy gap (
Eg) near the absorption edge using the Tauc's equation
38where
A is a constant,
Eg is the optical band gap,
h is the Planck constant,
ν the frequency of the incident photon and
m is a constant which characterizes the nature of band transition.
m can have values of 1/2, 3/2, 2 and 3 depending on the nature of the electronic transition responsible for the absorption.
m = 1/2 for allowed direct transition,
m = 3/2 for forbidden direct transition and
m = 3 for forbidden indirect transition, while
m = 2 refer to indirect allowed transitions.
39 Among all possible transitions,
m = 1/2 is found to be more suitable for VM crystal since it gives the best linear curve in the band edge and hence the transition is direct transition. The variation of (
αhν)
2 with
hν (Tauc plot) for VM crystal is shown in the inset of
Fig. 10. The value of
Eg for the sample is calculated by extrapolating the linear region of the curve to meet the energy axis at (
αhν)
2 = 0. The value of
Eg thus obtained is found to be 3.82 eV.
Second harmonic generation efficiency measurement. The non-linear optical property was confirmed by the second harmonic generation (SHG) efficiency test of the grown crystals by the Kurtz powder technique.40 The grown crystal was finely powdered to a uniform particle size of 120–150 μm and filled in the capillary tube. The capillary is subjected to a fundamental Q-switched Nd:YAG laser beam of wavelength 1064 nm, 8 ns pulse width and energy 6.3 mJ per pulse. Potassium dihydrogen phosphate (KDP) was taken as a reference material. Emission of green light confirms the second harmonic generation by VM crystal and this light of wavelength 532 nm was finally detected by a photomultiplier tube (PMT) and displayed on the oscilloscope (CRO). It observed that the measured SHG efficiency of VM was 0.3 times of potassium di-hydrogen phosphate (KDP).
Mechanical properties
The variation of Vickers hardness number (Hv) with load (P) for the plane (200) of di-valine maleic (VM) crystal of thickness 0.99 mm is shown in Fig. 11. The figure shows that the hardness value increases with increasing load. Thus the plane considered for study exhibit reverse indentation size effect (ISE).41
 |
| Fig. 11 Variation of microhardness with load of divaline maleic crystal. Inset: Meyer's plot. | |
In the case of the reverse ISE a specimen does not offer resistance or undergo elastic recovery, but undergoes relaxation involving a release of the indentation stress away from the indentation site. This leads to a larger indentation size which gives rise to a lower hardness at low loads.41 When the indenter just touches the surface of the crystal, dislocations are generated in the indenter region, so microhardness increases initially. Above a particular load, the microhardness attains a constant value due to the rearrangement of dislocations and mutual interactions of dislocations.42 The hardness and strength critically depend on the ease with which dislocations move. To understand the existence of ISE, we used the classical power law (7) known as Meyer's law,
where
A is a power fit constant, and the exponent
n is the work hardening coefficient or the Meyer's index. The plot of log
P vs. log
d is shown in inset of
Fig. 11. This curve is linear fitted and its slope gives the Meyer's index number ‘
n’ of the sample. Combining
eqn (2) and
(7) we have
From relation (9) it is clear that for normal ISE the value of ‘n’ should be less than 2. In the present study, the value of n is 2.958 which being greater than 2 confirms the reverse ISE. From careful observations on various materials, Onitsch43 and Hanneman pointed out that n lie between 1 and 1.6 for hard materials, and it is more than 1.6 for soft materials. In the present study, n being greater than 2 confirms the prediction of Onitsch43 that the material is soft and follows reverse ISE. From the hardness value, the yield strength (σv) can be calculated using the relation for Meyer's index n > 2,44
|
 | (10) |
where
σv is the yield strength in MPa,
Hv is the Vicker's hardness number in kg mm
−2. Also the elastic stiffness constant (
C11) calculated using Wooster's
45 empirical formula [
C11 =
Hv7/4] for different load is tabulated in
Table 4. This gives an idea about stiffness of bonding between neighbouring atoms.
Table 4 The values of elastic stiffness constant (C11) and the yield strength (σv) at different loads
Load (g) |
Yield strength (σv) × 109 (Pa) |
Stiffness constant (C11) × 1016 (Pa) |
1 |
15.34 |
8.47 |
5 |
24.74 |
19.55 |
10 |
33.49 |
33.23 |
15 |
36.19 |
38.04 |
Computational studies
The optimization of the geometry of VM molecule was completed by using B3LYP functional and 6-31G(d,p) basis set. The dipole moment, polarizability and hyperpolarizability of the optimized structure were then calculated with the same functional and basis set and listed in Table 5. The value of dipole moment is maximum (3.3606 D approx.) along x-direction. Table 5 shows the calculated polarizability of VM molecule, αij, have non zero values and are dominated by the diagonal components. The theoretical calculation seems to be more helpful in the determination of particular components of β tensor than in establishing the real values of β. The biggest value of hyperpolarizability is noted along βxxx direction and subsequently delocalization of electron cloud is more in this particular direction. The calculated first-order hyperpolarizability (βtot) of VM is 4.4456 × 10−30 esu, which is nearly 5 times that of urea [0.8103 × 10−30 esu]. This high value of β and the non-zero value of μ are responsible for the second order nonlinear optical properties such as SHG.
Table 5 The dipole moment (μ), polarizability (α) and first hyperpolarizability (β) of VM derived from computational calculations
Dipole moment (μ) debye |
μx |
3.3606 |
μy |
1.7952 |
μz |
2.5396 |
μtot |
4.5788 |
![[thin space (1/6-em)]](https://www.rsc.org/images/entities/char_2009.gif) |
Polarizability (α) × 10−23 esu |
αxx |
1.0334 |
αxy |
−0.0641 |
αyy |
0.9855 |
αtot |
1.5958 |
αxz |
−0.0108 |
αyz |
−0.0073 |
αzz |
0.8483 |
Δα |
0.1675 |
![[thin space (1/6-em)]](https://www.rsc.org/images/entities/char_2009.gif) |
Hyperpolarizability (β) × 10−30 esu |
βxxx |
1.9985 |
βxxy |
0.6042 |
βxyy |
0.8027 |
|
|
βyyy |
0.7489 |
βxxz |
0.5543 |
βxyz |
0.4933 |
βtot |
4.4456 |
βyyz |
0.6769 |
βxzz |
0.7513 |
βyzz |
0.5685 |
|
|
βzzz |
0.6260 |
|
|
|
|
|
|
The plot of the frontier molecular orbitals, HOMO and LUMO are shown in Fig. 12. The charge transfer character of the HOMO–LUMO transition is demonstrated by the topology of these orbitals.
 |
| Fig. 12 HOMO–LUMO plot of VM molecule. | |
It is found that the HOMO is localized mainly over the carboxyl group of the valine molecule while the LUMO are mostly delocalized over the C–C bonds of the maleic acid molecule. The energy eigenvalues and the energy gap for the HOMO and the LUMO as calculated at B3LYP/6-31G(d,p) level is shown in Fig. 12. The value of the energy gap is 2.9657 eV. This low HOMO–LUMO energy gap explains the probable intermolecular charge transfer (ICT) taking place inside the molecule enhancing the molecular hyperpolarizability. This asserts the suitability of the grown crystal for NLO application.
Conclusions
An organic nonlinear optical material, di-valine maleic (VM) has been synthesized and grown by slow evaporation of solvent method at room temperature and structurally characterized by single crystal X-ray diffraction. VM displays two-dimensional supramolecular frameworks in the solid-state and Hirshfeld surface analysis was successfully deployed for quantification of intermolecular interactions. Crystal of size 10 × 3.5 × 1.2 mm3 was obtained. The VM crystal has a wide transparency range in the entire visible and NIR range. The second harmonic generation efficiency of VHM is 0.3 times of KDP. The thermal behaviour of the grown crystals proved the absence of water of crystallization. From the mechanical measurements, it was observed that the hardness of VM follows reverse indentation size effect. The value of work hardening coefficient is 2.958. Calculated first order hyperpolarizability (βtot) of VM is 4.4456 × 10−30 esu, which is nearly 5 times that of urea [0.8103 × 10−30 esu]. The low value of HOMO–LUMO gap might have enhanced the molecular hyperpolarizability too. Moreover the decrease in HOMO–LUMO gap explains charge transfer from electron donor to electron acceptor groups. Due to such specific properties we can consider VM crystal as a potential NLO material.
Acknowledgements
Authors are grateful to the DST-funded National Single Crystal X-ray Diffraction facility at the Department of Inorganic Chemistry, Indian Association for the Cultivation of Science, India, for data collection. S. K. Seth acknowledges University Grants Commission (New Delhi) India for Major Research Project [42-830/2013 (SR)].
Notes and references
- J. Zyss, Molecular Nonlinear Optics Materials, Physics and Devices, Academic Press, New York, 1994 Search PubMed.
- J. F. Nicoud, R. J. Twieg, D. S. Chemla and J. Zyss, in Nonlinear Optical Properties of Organic Molecules and Crystals, Academic Press, London, 1987 Search PubMed.
- B. Dalhus and C. H. Gorbitz, Acta Chem. Scand., 1996, 50, 544–548 CrossRef CAS.
- S. Moitra, S. K. Seth and T. Kar, J. Cryst. Growth, 2010, 312, 1977–1982 CrossRef CAS.
- S. Moitra and T. Kar, J. Cryst. Growth, 2008, 310, 4539–4543 CrossRef CAS.
- T. K. Kumar, R. S. Selvaraj, S. Janarthanan, Y. C. Rajan, S. Selvakumar, S. Pandi, M. S. Selvakumar and D. P. Anand, Eur. Phys. J.: Appl. Phys., 2010, 5, 20401 CrossRef.
- R. Parthasarathy, Acta Crystallogr., 1966, 21, 422–426 CrossRef CAS PubMed.
- S. Asath Bahadur, R. K. Rajaram, M. Nethaji and S. Natarajan, Z. Kristallogr., 1993, 203, 93–100 Search PubMed.
- S. Pandiarajan, B. Sridhar and R. K. Rajaram, Acta Crystallogr., Sect. E: Struct. Rep. Online, 2001, 57, o466–o468 CAS.
- K. Anitha, B. Sridhar and R. K. Rajaram, Acta Crystallogr., Sect. E: Struct. Rep. Online, 2004, 60, o1530–o1532 CAS.
- L. Bendheif, N. Benali-Cherif, L. Benguedouar, K. Bouchouit and H. Merazig, Acta Crystallogr., Sect. E: Struct. Rep. Online, 2003, 59, o141–o142 CAS.
- Bruker, SAINT, version 6.36a, Bruker-AXS Inc., Madison, Wisconsin, USA, 2002 Search PubMed.
- Bruker, SMART, version 5.625 and SADABS, version 2.03a, Bruker AXS Inc., Madison, Wisconsin, USA, 2001 Search PubMed.
- G. M. Sheldrick, Acta Crystallogr., Sect. A: Found. Crystallogr., 2008, 64, 112–122 CrossRef CAS PubMed.
- A. L. Spek, PLATON, Molecular Geometry Program, J. Appl. Crystallogr., 2003, 36, 7–13 CrossRef CAS.
- L. J. Farrugia, J. Appl. Crystallogr., 1999, 32, 837–838 CrossRef CAS.
- M. A. Spackman and J. J. McKinnon, CrystEngComm, 2002, 4, 378–392 RSC.
- J. J. McKinnon, D. Jayatilaka and M. A. Spackman, Chem. Commun., 2007, 3814–3816 RSC.
- S. K. Seth, D. Sarkar, A. D. Jana and T. Kar, Cryst. Growth Des., 2011, 11, 4837–4849 CAS.
- S. K. Seth, D. Sarkar and T. Kar, CrystEngComm, 2011, 13, 4528–4535 RSC.
- S. K. Seth, D. Sarkar, A. Roy and T. Kar, CrystEngComm, 2011, 13, 6728–6741 RSC.
- P. Manna, S. K. Seth, M. Mitra, A. Das, N. J. Singh, S. R. Choudhury, T. Kar and S. Mukhopadhyay, CrystEngComm, 2013, 15, 7879–7886 RSC.
- M. A. Spackman and P. G. Byrom, Chem. Phys. Lett., 1997, 267, 215–220 CrossRef CAS.
- J. J. McKinnon, A. S. Mitchell and M. A. Spackman, Chem.–Eur. J., 1998, 4, 2136–2141 CrossRef CAS.
- J. J. McKinnon, M. A. Spackman and A. S. Mitchell, Acta Crystallogr., Sect. B: Struct. Sci., 2004, 60, 627–668 CrossRef PubMed.
- A. L. Rohl, M. Moret, W. Kaminsky, K. Claborn, J. J. Mckinnon and B. Kahr, Cryst. Growth Des., 2008, 8, 4517–4525 CAS.
- S. K. Seth, I. Saha, C. Estarellas, A. Frontera, T. Kar and S. Mukhopadhyay, Cryst. Growth Des., 2011, 11, 3250–3265 CAS.
- P. Manna, S. K. Seth, A. Das, J. Hemming, R. Prendergast, M. Helliwell, S. R. Choudhury, A. Frontera and S. Mukhopadhyay, Inorg. Chem., 2012, 51, 3557–3571 CrossRef CAS PubMed.
- S. K. Seth, CrystEngComm, 2013, 15, 1772–1781 RSC.
- S. K. Wolff, D. J. Grimwood, J. J. McKinnon, D. Jayatilaka and M. A. Spackman, CrystalExplorer 3.0, University of Western Australia, Perth, Australia, 2007 Search PubMed.
- D. Sajan, J. Hubert, V. S. Jayakumar and J. Zaleski, J. Mol. Struct., 2006, 785, 43–53 CrossRef CAS.
- D. A. Kleinman, Phys. Rev., 1962, 126, 1977–1979 CrossRef CAS.
- M. J. Frisch, G. W. Trucks, H. B. Schlegel, G. E. Scuseria, M. A. Robb, J. R. Cheeseman, G. Scalmani, V. Barone, B. Mennucci, G. A. Petersson, H. Nakatsuji, M. Caricato, X. Li, H. P. Hratchian, A. F. Izmaylov, J. Bloino, G. Zheng, J. L. Sonnenberg, M. Hada, M. Ehara, K. Toyota, R. Fukuda, J. Hasegawa, M. Ishida, T. Nakajima, Y. Honda, O. Kitao, H. Nakai, T. Vreven, J. A. Montgomery Jr, J. E. Peralta, F. Ogliaro, M. Bearpark, J. J. Heyd, E. Brothers, K. N. Kudin, V. N. Staroverov, T. Keith, R. Kobayashi, J. Normand, K. Raghavachari, A. Rendell, J. C. Burant, S. S. Iyengar, J. Tomasi, M. Cossi, N. Rega, J. M. Millam, M. Klene, J. E. Knox, J. B. Cross, V. Bakken, C. Adamo, J. Jaramillo, R. Gomperts, R. E. Stratmann, O. Yazyev, A. J. Austin, R. Cammi, C. Pomelli, J. W. Ochterski, R. L. Martin, K. Morokuma, V. G. Zakrzewski, G. A. Voth, P. Salvador, J. J. Dannenberg, S. Dapprich, A. D. Daniels, O. Farkas, J. B. Foresman, J. V. Ortiz, J. Cioslowski and D. J. Fox, Gaussian 09, Revision B.01, Gaussian Inc., Wallingford, CT, 2010 Search PubMed.
- G. Gece, Corros. Sci., 2008, 50, 2981–2992 CrossRef CAS.
- P. W. Atkins, Physical Chemistry, Oxford University Press, Oxford, 2001 Search PubMed.
- L. J. Farrugia, ORTEP III, version 1.06, Department of Chemistry, University of Glasgow, Glasgow, Scotland, U.K., 1997 Search PubMed.
- A. S. H. Hameed, G. Ravi, R. Dhanasekaran and P. Ramasamy, J. Cryst. Growth, 2000, 212, 227–232 CrossRef.
- J. Tauc, Amorphous and Liquid Semiconductors Amorphous and Liquid Semiconductors, Plenum, New York, 1974 Search PubMed.
- M. A. Kaid and A. Ashour, Appl. Surf. Sci., 2007, 253, 3029–3033 CrossRef CAS.
- S. K. Kurtz and T. T. Perry, J. Appl. Phys., 1968, 39, 3798–3813 CrossRef CAS.
- K. Sangwal, Mater. Chem. Phys., 2000, 63, 145–152 CrossRef CAS.
- S. A. Bahadur and V. Ramakrishnan, Proceedings of the Symposium on Crystal Nucleation, Solution Growth and Surface Morphology, 1990, p. 100 Search PubMed.
- E. M. Onitsch, Mikroskopie, 1950, 95, 12–14 CAS.
- J. P. Cahoon, W. H. Bhoughton and A. R. Katzuk, Metall. Trans., 1971, 2, 1979–1983 CAS.
- W. A. Wooster, Rep. Prog. Phys., 1953, 16, 62–82 Search PubMed.
|
This journal is © The Royal Society of Chemistry 2016 |