DOI:
10.1039/C6RA21326J
(Paper)
RSC Adv., 2016,
6, 103740-103746
An electronic tongue based on conducting electrospun nanofibers for detecting tetracycline in milk samples
Received
25th August 2016
, Accepted 22nd October 2016
First published on 25th October 2016
Abstract
The development of novel and portable chemical sensors aimed at the food industry is of prime importance for food safety issues, and nanomaterial science can greatly contribute to this task. In this context, a careful choice of the sensing material is essential for achieving high performance sensor arrays, such as those employed in nanostructured electronic tongues (e-tongues). In the current work, an impedimetric e-tongue based on gold interdigitated microelectrodes (IDEs) modified with polyamide 6/polyaniline (PA6/PANI) electrospun nanofibers was developed, characterized and used to detect tetracycline (TC) residue in fat and skimmed milk samples. By analyzing the electrical resistance data collected by the e-tongue, which were treated by Principal Component Analysis (PCA), the e-tongue was able to identify the presence of TC residues (from 1 to 300 ppb) in fat and skimmed milk samples. The results obtained demonstrate the ability of the approach of modifying IDEs with conducting electrospun nanofibers to be used as sensing units in the e-tongue, aiming to analyze complex matrices such as milk without any prior pre-treatment.
1. Introduction
Food safety problems, especially those caused by food contamination, have received great attention from researchers, as they cause deleterious effects and affect human health.1,2 In this regard, the presence of antibiotics residues in foodstuffs creates a potential hazard for the consumer and may cause several consequences such as bacterial resistance and allergic side effects.3–5
Tetracyclines (TC), a class of broad-spectrum and effective antibiotics, are widely used in veterinary medicine for treatment of infections in food-producing animals,6 and have become one of the greatest concerns regarding food contaminants.7 Milk and dairy products industries are the sectors most affected by the presence of these antibiotic residues, which can lead to considerable losses in fermented products.8 Currently, a variety of analytical methods have been developed for the detection of TC residues, including capillary electrophoresis,9 colorimetric methods,10 chemiluminescence11 and high performance liquid chromatography.12 However, some of the above mentioned methods are time-consuming and require expensive apparatuses. Therefore, the development of simple, low-cost, sensitive and rapid methods for detecting TC residues in milk remains an important requirement for food safety monitoring.
Over the last years, the electronic tongue (e-tongue) technology has been used to assess the quality of a variety of foods.13–16 An e-tongue is usually comprised of an array of nonspecific or low-selectivity sensors (sensing units) that display high cross-sensitivity. Such type of sensor, under exposure to the specific analyte or chemical species, provides a characteristic response pattern closely associated with the characteristics of the sample analyzed.13 One crucial step for designing the sensing units of an e-tongue lies on the choice of the sensor active layer. In the last decade, novel materials and methodologies have been proposed for such task. For instance, strategies based on the modification of the electrode surfaces with nanomaterials, such as nanoparticles,17,18 carbon nanomaterials19 and nanofibers20 have been reported. The remarkable high specific surface area and large porosity bring electrospun nanofibers highly attractive to design ultrasensitive sensors. Various materials such as polymers, ceramics and carbon-based compounds can be used to produce uniform electrospun fibers with well-controlled sizes, compositions, and morphologies.21 In particular, electrospun nanofibers based on conductive polymers are potential candidates for applications in sensing devices,22–24 due to their high electrical conductivity. In this regard, one of the most prominent conducting polymer is polyaniline (PANI) because of its ease of synthesis, environmental stability and intrinsic redox reaction.25 However, obtaining pristine PANI nanofibers by electrospinning is difficult due to its very low solubility when doped, even when using high polar solvents.26 In order to overcome this challenge, the use of a polymer that can be easily electrospun to form uniform nanofibers, such as polyamide 6 (PA6),22,27 blended to small amounts of PANI, is an excellent strategy to obtaining conducting electrospun fibers.
In this work, we report the development of a rapid and accurate sensing platform based on conducting electrospun nanofibers aimed at the qualitative analysis of milk samples contaminated with TC. We fabricated an array of sensors based on electrospun PA6/PANI nanofibers deposited onto gold interdigitated microelectrodes (IDEs), which was used in an impedimetric e-tongue system, as illustrated in Scheme 1(i). The experimental data was treated by Principal Component Analysis (PCA), and allowed the identification of the presence of TC residues in fat and skimmed milk (Scheme 1(ii)).
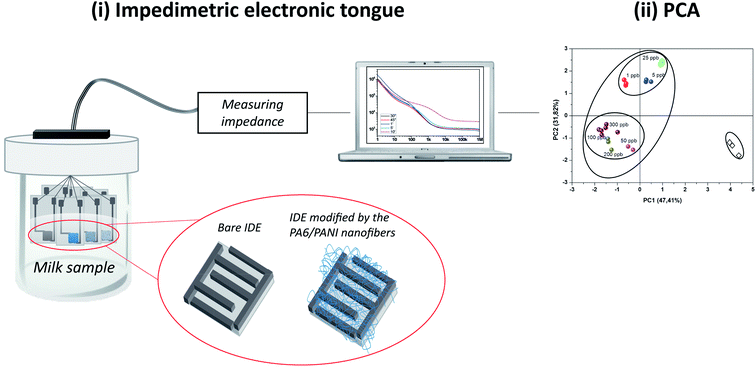 |
| Scheme 1 Experimental setup based on an (i) impedimetric electronic tongue composed of an array of interdigitated electrodes (IDE's) modified by electrospun nanofibers of PA6/PANI, which was employed for analyzing milk samples regarding the presence of antibiotic tetracycline. (ii) The experimental data collected with the impedimetric electronic tongue was treated by Principal Components Analysis. | |
2. Experimental
2.1 Materials
Tetracycline ≥98% (TC), polyaniline (PANI) (emeraldine base, Mw = 20
000 g mol−1), polyamide 6 (PA6, Mw = 20
000 g mol−1) and 1,1,1,3,3,3-hexafluoro-2-propanol (HFIP) were purchased from Sigma-Aldrich. Gold interdigitated microelectrodes (IDEs), each one comprising 50 pairs of fingers with finger width and distance between fingers of 10 μm, were fabricated by conventional photolithography at the Polytechnic School of the University of São Paulo (EPUSP).
2.2 Electrospinning of PA6 and PA6/PANI nanofibers
PA6 and PA6/PANI solutions were prepared by dissolving the polymers in HFIP under stirring for 5 h at room temperature. PA6 solution was prepared in concentrations of 5% (w/v in respect to the solvent), while PA6/PANI solution was prepared using 5% of PA6 (w/v in respect to the solvent) and distinct concentrations of PANI: 0.25, 0.5, 1, 2.5 and 5% (w/w in respect to PA6), yielding the samples named PA6, PA6/PANI 0.25%, PA6/PANI 0.5%, PA6/PANI 1.0%, PA6/PANI 2.5%, PA6/PANI 5.0%, respectively. The nanofibers were obtained with a homemade electrospinning apparatus using a feed rate of 0.01 mL h−1, an electric voltage of 16 kV, while a working distance of 5 cm was kept between the syringe and the metallic collector. The inner diameter of steel needle was 0.8 mm. Random nanofibers were directly electrospun onto the IDEs surface, using a rotating speed of 300 rpm, to obtain the modified microelectrodes. The IDEs were attached at the same position on the metallic collector for all experiments with an optimized collection time of 45 seconds.
2.3 Nanofibers characterization
The morphology of the samples was evaluated by a Scanning Electron Microscope (SEM, JEOL 6510), while the average fiber diameter was estimated using the SEM images with the aid of an image analysis software (Image J, National Institutes of Health, USA).
The Raman spectra in the range 800–2000 cm−1 were collected using a FTIR spectrometer (Bruker Vertex 70) coupled to a FT-Raman module using an excitation wavelength at 1064 nm of a Nd:YAG laser; 128 scans were used for each measurement at room temperature.
The X-ray diffractograms were recorded using a Shimadzu XRD 600 diffractometer operating at 30 kV, 30 mA and CuKα radiation (k = 1.540 Å). The samples were prepared by placing a small section of the electrospun mat on the glass sample holder.
Differential scanning calorimetry (DSC) analyses were performed on a Q100 TA calorimetric analyzer under nitrogen atmosphere, at a flow rate of 50 mL min−1. The samples were heated from −80 to 270 °C at a heating rate of 10 °C min−1 using aluminum pans.
2.4 Milk samples
UHT milks with different fat contents (skimmed and fat) were purchased from a local supplier. The milk samples were prepared by diluting 25 μL of each milk sample in 100 mL of distilled water. Contamination of the milk samples was accomplished by mixing proper amounts of a standard solution of TC to the milk samples, reaching final concentrations of 1, 5, 25, 50, 100, 200 and 300 ppb.
2.5 Sensor application
Electrical characterization of each individual sensing unit of the electronic tongue was performed using an impedance gain phase analyzer (Solartron, model 1260, UK). The electrical data, collected by the impedance analyzer, were acquired in the frequency range from 1 Hz up to 1 MHz, using as electrical voltage 200 mV.
The sensor array composing the e-tongue was comprised of seven sensing units: a bare IDE, an IDE coated with PA6 nanofibers and five IDEs coated with PA6/PANI 0.25%, PA6/PANI 0.5%, PA6/PANI 1.0%, PA6/PANI 2.5%, PA6/PANI 5.0% nanofibers, respectively. Before using the modified electrodes as sensing units in the e-tongue system, several measurements were performed with several bare electrodes immersed in distilled water to guarantee similar electrical responses. The electrodes were left soaked into the milk solutions for 10 min prior to the beginning of the electrical measurements. Five independent data acquisitions using the e-tongue were performed with each sensing unit immersed in the uncontaminated and contaminated milk samples. The measurements started with the pure milk samples and then from the lowest (1 ppb) to the highest (300 ppb) concentration of TC in the milk solutions. The sensors could be reused in further experiments by carefully washing the sensing units with distilled water, in order to remove molecules entrapped within the films. The electrical resistance values obtained at 1 kHz were treated and analyzed using Principal Component Analysis (PCA), a statistical method that reduces the dimensionality of data (without loss of information), assisting in recognizing, grading, and verifying correlation between the samples.28
3. Results and discussion
3.1 Nanofibers characterization
The average diameter of each nanofiber sample (PA6, PA6/PANI 0.25%, PA6/PANI 0.5%, PA6/PANI 1.0%, PA6/PANI 2.5%, PA6/PANI 5.0%) produced by electrospinning is shown in Fig. 1(a). It is possible to observe a remarkable decrease in the fiber diameter as the PANI content increases from 0% to 1%, reaching a plateau for higher concentrations of PANI. As illustration, Fig. 1(b) and (c) show the SEM images of samples PA6 (276 ± 74 nm) and PA6/PANI 5.0% (137 ± 53 nm), both revealing a smooth surface, but a remarkable difference of diameters. This behavior was also found by Li et al.29 and may be attributed to an increase in the charge density as the PANI content increases. All these features contributed to the superior stretching of the polymer jet during the electrospinning process.
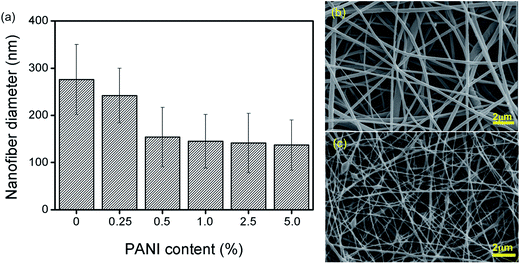 |
| Fig. 1 (a) Nanofiber diameters using different PANI concentrations. SEM images of (b) PA6 and (c) PA6/PANI 5.0% nanofiber samples. | |
Fig. 2 shows the Raman spectra for the electrospun nanofibers with different PANI concentrations. The spectra are dominated by the spectral features of PANI, once PA6 has low Raman optical activity under the experimental conditions employed (black curve in Fig. 2). Characteristic PANI bands at 1602 (C–C stretching of benzenoid rings), 1506 (C
N stretching of quinoid rings), 1375 (
C–N+· stretching mode of semiquinone radical) and 1172 cm−1 (C–H deformation of the quinoid ring) were observed for all PA6/PANI nanofibers. The intensity of these bands tends to increase as the PANI content increases, corroborating that a larger amount of PANI is within the PA6 nanofiber bulk. The semiquinone radical band usually appears in the range 1320–1340 cm−1 for pure PANI and it is characteristic of doped PANI (emeraldine oxidation state).30 The red shift observed for the blended nanofibers indicates an interaction of PANI with PA6 matrix and was also observed by Fatyeyeva et al. for conducting polymer films based on blends of polyamides and polyaniline.31
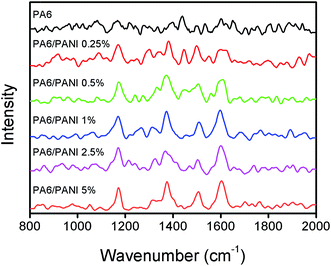 |
| Fig. 2 RAMAN spectra of PA6 and PA6/PANI nanofibers with different PANI concentrations. | |
Fig. 3(a) shows the XRD diffractograms of PA6 and PA6/PANI electrospun nanofibers. PA6 can crystallize in α (monoclinic, stable, planar zigzag conformation) and γ (pseudo-hexagonal, metastable, helix conformation) phases. The α-phase diffracts prominently the planes (200) and (002), which corresponds to 2θ of 20° and 22°, respectively, while the γ phase has a predominant diffraction peak at plane (200) which corresponds to 2θ = 21°.32 It can be observed that in all the samples, the γ phase is the main crystalline phase, while the intensity of the α-peaks changed with the addition of PANI. This result indicates that the presence of PANI does not appear to interfere on the type of obtained crystalline phases of PA6, but only on the relative distribution between the phases.
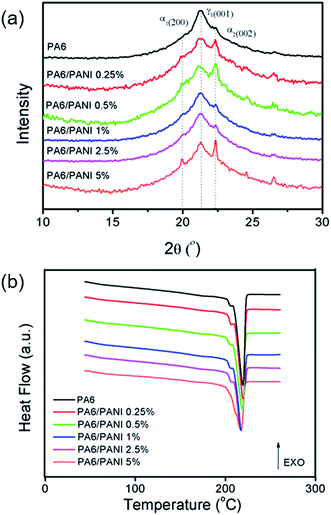 |
| Fig. 3 (a) XRD patterns and (b) DSC curves of PA6 and PA6/PANI nanofibers with different PANI concentrations. | |
The evaluation of the thermal behavior of the nanofibers was performed by DSC technique (Fig. 3(b)). The DSC curve for pure PA6 nanofibers displays two endothermic events Tm1 (204 °C) and Tm2 (220 °C), corresponding to melting of the γ and α phase crystals, respectively, which is in agreement with the X-ray results presented above. The same behavior was observed for the PA6/PANI nanofibers. The melting temperatures show essentially no change with increase of PANI content, indicating that the addition of PANI (up to 5%) appears not to cause a deep change to the crystallization process of PA6.
3.2 Electrical characterization
In order to investigate how the amount of the nanofiber deposited onto the IDE influences its electrical response, several electrospinning fiber collection times were evaluated. The amount of deposited fibers is a decisive factor for the sensor performance, since the electrode should present a not very high electrical resistance, but at the same time a large contact area between the sensing layer and target analyte is required. Fig. 4 shows the SEM images and the electrical resistance response at 1 kHz of the IDEs modified with PA6/PANI (5%) nanofibers using distinct deposition times. Responses were analyzed at 1 kHz, because capacitance/resistance measurements at this frequency are sensitive to changes in the film properties due to the liquid sample.33 The electrical resistance in distilled water remains the same until 1 minute and then increases as more nanofibers are deposited onto the surface of the IDEs (Fig. 4(f)). The different resistance levels are due to the different amounts of randomly oriented nanofibers deposited, which may decrease the interaction at the electrode/solution interface (Fig. 4a–e). This behavior is illustrated in Fig. 4(g), which shows a IDE picture (left side) and a scheme of the side view (right side) of an electrode and the possible organization of the fibers at three different deposition times (30 s, 45 s and 10 min). From the results obtained, 45 s was chosen as the optimized deposition time due to the lower electrical resistance presented by the electrode and the homogenous and suitable amount of fibers deposited onto the electrode surface.
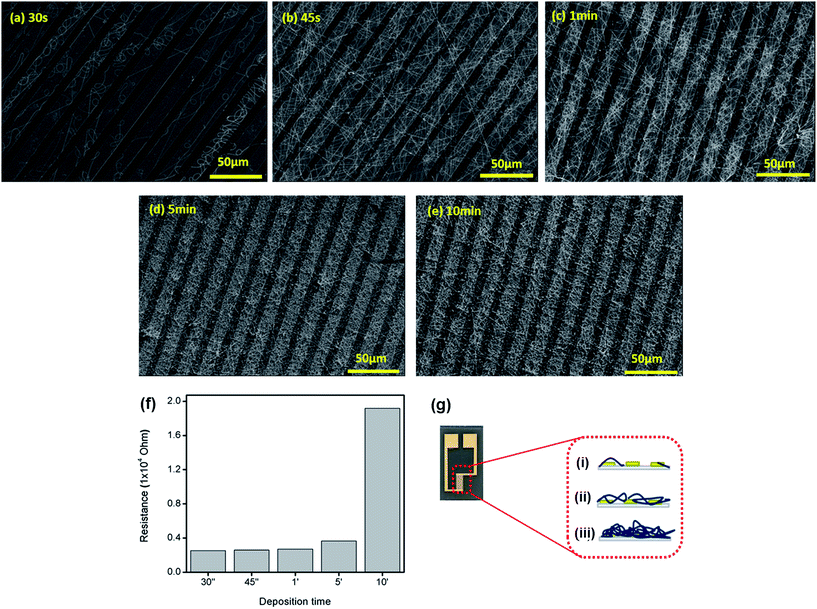 |
| Fig. 4 (a–e) SEM images and (f) resistance response of the IDEs after modification with PA6/PANI (5%) nanofibers using different deposition times. (g) IDE picture (left side) and side view scheme (right side) of an interdigitated electrode and the possible organization of the random nanofibers deposited at (i) 30 s, (ii) 45 s and (iii) 10 min. | |
3.3 Milk analyses – e-tongue measurements
As an attempt to develop a reliable chemical sensor for analyzing milk samples contaminated with TC residues, an e-tongue was assembled, composed of a bare IDE, an IDE modified with PA6 nanofibers and five IDEs coated with PA6/PANI 0.25%, PA6/PANI 0.5%, PA6/PANI 1.0%, PA6/PANI 2.5%, PA6/PANI 5.0% nanofibers, respectively. The evaluation of milk samples with different fat content (skimmed and fat) and different TC concentrations (1–300 ppb) was carried out by electrical impedance measurements and the electrical resistance data were recorded at 1 kHz for all sensing units. To determine the power of discrimination and separation of the proposed sensor array, principle component analysis (PCA) approach was used for pattern recognition using the electrical resistance data collected by the e-tongue. By using orthogonal transformation, PCA converts a group of observations of likely correlated variables into a group of values of linearly-uncorrelated variables (called principal components). PCA presents the pattern of similarities of variables as points on a graph; the direction in the feature space along with the projections that have the largest variance are indicated by the first principal component, while the direction that maximizes variance among all directions orthogonal to the first principal component is indicated by the second principal component.34 This type of method is essential to exploiting the cross-sensitivity from different sensing units in arrays, such as in e-tongues and e-noses.35
The PCA plots obtained from the measurements with the sensing units immersed in fat milk samples shown in Fig. 5(a) demonstrate that contaminated solutions can be clearly distinguished from uncontaminated samples, even at very low concentrations (1 ppb). For instance, we note that pure milk sample is located on the positive side of PC1 and the negative side of PC2. Samples with distinct TC contents are easily distinguished, and the TC content can be correlated with PC2, where the samples contaminated with high TC levels are located on the negative side of PC2, while the milk samples with low concentrations of TC are grouped on the positive side of PC2. It is worth mentioning that the data variance was largely accounted by PC1 + PC2 (80%).
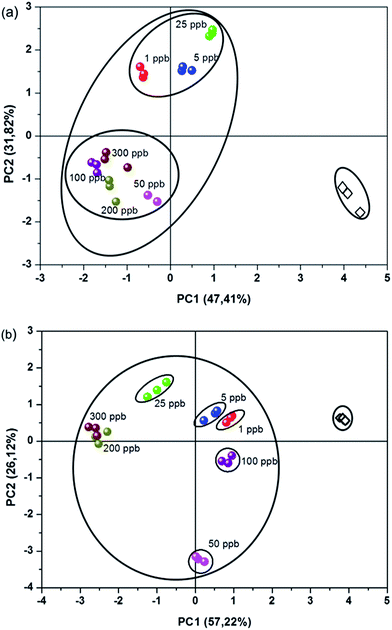 |
| Fig. 5 PCA plots obtained from electrical resistance values recorded at 1 kHz and 200 mV for: (a) fat and (b) skimmed milk samples uncontaminated (open lozenge) and contaminated with different TC concentrations – from 1 up to 300 ppb (color circles). The data variance for PC1 and PC2 is included in the figure. | |
Fig. 5(b) shows the PCA plot obtained for the skimmed milk samples. The score plots for each milk sample indicated that the non-contaminated sample could also be distinguished from the contaminated samples. Furthermore, the samples could be separated by concentration, indicating that the proposed array of sensor was not limited to a single type of milk. Moreover, the data variance was mainly accounted by the two first principal components, where (PC1 + PC2) responded to 83% of the total information collected by the array (Fig. 5).
Therefore, it can be concluded that the e-tongue composed by sensors based on electrospun nanofibers were capable of distinguishing milk samples with distinct tetracycline concentrations. This sensor array shows a high sensitivity, capable of detecting concentrations of 1 ppb of tetracycline, which is comparable to results achieved by other sophisticated analytical techniques, such as HPLC.36,37
4. Conclusions
A novel nanostructured platform based on PA6/PANI electrospun nanofibers was successful assembled onto gold interdigitated microelectrodes and used as sensing units of an impedimetric electronic tongue, aiming at detecting tetracycline residues in bovine milk samples. The sample preparation procedure without extensive steps led to a less time consuming and sensitive method that could be applied in routine milk analysis. Furthermore, the results present here demonstrate the ability of our nanostructured sensor to detect tetracycline in fat and skimmed milk at concentration levels well below the maximum residue level allowed for TC (100 ppb), according to the Brazil National Health Surveillance Agency (ANVISA).38
Acknowledgements
The authors acknowledge the financial support from FAPESP (Grants numbers: 2014/16789-5 and 2012/23880-3), CNPq, CAPES, MCTI-SisNano, Rede Agronano and Embrapa from Brazil.
References
- Z. Xiaobo, H. Xiaowei and M. Povey, Analyst, 2016, 141, 1587–1610 RSC.
- R. J. Fussell, M. Garcia Lopez, D. N. Mortimer, S. Wright, M. Sehnalova, C. J. Sinclair, A. Fernandes and M. Sharman, J. Agric. Food Chem., 2014, 62, 3651–3659 CrossRef CAS PubMed.
- X. Wang, S. Dong, P. Gai, R. Duan and F. Li, Biosens. Bioelectron., 2016, 82, 49–54 CrossRef CAS PubMed.
- R. V. Oliveira, A. C. De Pietro and Q. B. Cass, Talanta, 2007, 71, 1233–1238 CrossRef CAS PubMed.
- E. Zacco, J. Adrian, R. Galve, M. P. Marco, S. Alegret and M. I. Pividori, Biosens. Bioelectron., 2007, 22, 2184–2191 CrossRef CAS PubMed.
- B. Bengtsson, H. E. Unnerstad, T. Ekman, K. Artursson, M. Nilsson-Öst and K. P. Waller, Vet. Microbiol., 2009, 136, 142–149 CrossRef CAS PubMed.
- S. E. Katz and M. S. Brady, Food Biotechnol., 2000, 14, 147–171 CrossRef CAS.
- S. A. de Albuquerque Fernandes, A. P. A. Magnavita, S. P. B. Ferrao, S. A. Gualberto, A. S. Faleiro, A. J. Figueiredo and S. V. Matarazzo, Environ. Sci. Pollut. Res., 2014, 21, 3427–3434 CrossRef PubMed.
- I. S. Ibarra, J. A. Rodriguez, J. M. Miranda, M. Vega and E. Barrado, J. Chromatogr. A, 2011, 1218, 2196–2202 CrossRef CAS PubMed.
- M. Ramezani, N. M. Danesh, P. Lavaee, K. Abnous and S. M. Taghdisi, Biosens. Bioelectron., 2015, 70, 181–187 CrossRef CAS PubMed.
- B. X. Jia, Y. L. Liu, Y. X. Qi and K. Li, Chin. J. Antibiot., 2009, 34, 425–428 CAS.
- C. Nebot, M. Guarddon, F. Seco, A. Iglesias, J. M. Miranda, C. M. Franco and A. Cepeda, Food Control, 2014, 46, 495–501 CrossRef CAS.
- A. Wadehra and P. S. Patil, Anal. Methods, 2016, 8, 474–480 RSC.
- X. Cetó, N. H. Voelcker and B. Prieto-Simón, Biosens. Bioelectron., 2016, 79, 608–626 CrossRef PubMed.
- S. Magdalena, P. Wisniewska, T. Dymerski, J. Namiesnik and W. Wardencki, J. Agric. Food Chem., 2014, 62, 1423–1448 CrossRef PubMed.
- C. M. Daikuzono, C. A. R. Dantas, D. Volpati, C. J. L. Constantino, M. H. O. Piazzetta, A. L. Gobbi, D. M. Taylor, O. N. Oliveira and A. Riul, Sens. Actuators, B, 2015, 207, 1129–1135 CrossRef CAS.
- L. A. Mercante, V. P. Scagion, A. Pavinatto, R. C. Sanfelice, L. H. C. Mattoso and D. S. Correa, J. Nanomater., 2015, 2015, 890637 Search PubMed.
- A. Manzoli, F. M. Shimizu, L. a. Mercante, E. C. Paris, O. N. Oliveira, D. S. Correa and L. H. C. Mattoso, Phys. Chem. Chem. Phys., 2014, 16, 24275–24281 RSC.
- A. C. De Sá, A. Cipri, A. González-Calabuig, N. R. Stradiotto and M. Del Valle, Sens. Actuators, B, 2016, 222, 645–653 CrossRef.
- J. E. Oliveira, V. P. Scagion, V. Grassi, D. S. Correa and L. H. C. Mattoso, Sens. Actuators, B, 2012, 171–172, 249–255 CrossRef CAS.
- B. Ding, M. Wang, X. Wang, J. Yu and G. Sun, Mater. Today, 2010, 13, 16–27 CrossRef CAS.
- R. S. Andre, A. Pavinatto, L. A. Mercante, E. C. Paris, L. H. C. Mattoso and D. S. Correa, RSC Adv., 2015, 5, 73875–73881 RSC.
- J. Liang, S. Su, X. Fang, D. Wang and S. Xu, Mater. Sci. Eng., B, 2016, 211, 61–66 CrossRef CAS.
- S. Y. Min, T. S. Kim, Y. Lee, H. Cho, W. Xu and T. W. Lee, Small, 2015, 11, 45–62 CrossRef CAS PubMed.
- J. Huang, S. Virji, B. H. Weiller and R. B. Kaner, Chem.–Eur. J., 2004, 10, 1314–1319 CrossRef CAS PubMed.
- R. K. Paul and C. K. S. Pillai, Polym. Int., 2001, 50, 381–386 CrossRef CAS.
- L. A. Mercante, A. Pavinatto, L. E. O. Iwaki, V. P. Scagion, V. Zucolotto, O. N. Oliveira, L. H. C. Mattoso and D. S. Correa, ACS Appl. Mater. Interfaces, 2015, 7, 4784–4790 CAS.
- H. Hotelling, J. Educ. Psychol., 1933, 24, 417–441 CrossRef.
- M. Li, Y. Guo, Y. Wei, A. G. MacDiarmid and P. I. Lelkes, Biomaterials, 2006, 27, 2705–2715 CrossRef CAS PubMed.
- Y. Furukawa, F. Ueda, Y. Hyodo, I. Harada, T. Nakajima and T. Kawagoe, Macromolecules, 1988, 21, 1297–1305 CrossRef CAS.
- K. Fatyeyeva, A. A. Pud, J. F. Bardeau and M. Tabellout, Mater. Chem. Phys., 2011, 130, 760–768 CrossRef CAS.
- A. B. Da Silva and R. E. S. Bretas, Synth. Met., 2012, 162, 1537–1545 CrossRef.
- D. M. Taylor and A. G. Macdonald, J. Phys. D: Appl. Phys., 1987, 20, 1277–1283 CrossRef CAS.
- M. Ringner, Nat. Biotechnol., 2008, 26, 303–304 CrossRef CAS PubMed.
- P. H. B. Aoki, P. Alessio, L. N. Furini, C. J. L. Constantino, T. T. A. T. Neves, F. V. Paulovich, M. C. F. De Oliveira and O. N. Oliveira, Langmuir, 2013, 29, 7542–7550 CrossRef CAS PubMed.
- X. Q. Yang, C. X. Yang and X. P. Yan, J. Chromatogr. A, 2013, 1304, 28–33 CrossRef CAS PubMed.
- A. L. Cinquina, F. Longo, G. Anastasi, L. Giannetti and R. Cozzani, J. Chromatogr. A, 2003, 987, 227–233 CrossRef CAS PubMed.
- H. A. Martins-júnior, T. a. Kussumi, A. Y. Wang and D. T. Lebre, J. Braz. Chem. Soc., 2007, 18, 397–405 CrossRef.
|
This journal is © The Royal Society of Chemistry 2016 |