DOI:
10.1039/C6RA21192E
(Paper)
RSC Adv., 2016,
6, 112148-112157
Synthesis and characterization of g-C3N4 nanosheet modified polyamide nanofiltration membranes with good permeation and antifouling properties
Received
23rd August 2016
, Accepted 22nd November 2016
First published on 22nd November 2016
Abstract
The incorporation of nano materials into a polyamide (PA) active layer of nanofiltration membranes has become a main choice for the improvement of membrane performance. In this paper, g-C3N4 nanosheets (CNs) were introduced to aqueous solution for the modification of a PA active layer via an interfacial polymerization reaction between piperazine and trimesoyl chloride monomers. FT-IR and XRD analysis confirmed the presence of CNs on the surface of nanofiltration membranes. SEM images indicated the formation of an active layer on the top of the polyethersulfone (PES) substrate and a good dispersion of CNs. AFM images proved that the surface roughness was increased after the incorporation of CNs. The contact angle measurement demonstrated that the surface hydrophilicity of the CN modified PA (PA/CNs) membrane was promoted. Thermal stability and surface charge of the membranes were investigated by TGA and zeta potential analysis, respectively. Compared with a pristine PA membrane, for the membrane containing CNs (0.0025 wt% in aqueous solution) a distinct increase of water flux from 20.9 L m−2 h−1 to 37.6 L m−2 h−1 at 2 bar was observed, while salt rejection of Na2SO4 was maintained above 84.0%. What's more, the anti-fouling properties of the PA/CNs membrane were investigated by using bovine serum albumin and humic acid aqueous solutions, and the results confirmed that the incorporation of CNs into PA membranes could improve antifouling performance.
1. Introduction
The shortage of fresh water has been a great challenge faced by humans, and demands researchers to find new methods and new business models to solve the issue.1 Nanofiltration (NF) is a kind of pressure driven membrane process between reverse osmosis and the ultrafiltration process, drawing people's attention increasingly in recent years.2 There are numerous advantages of NF membranes such as high retention for organic solutions and salts with molecular weights in the range of 100–1000 Da,3 low initial investment compared with reverse osmosis, etc.4 Since the method of interfacial polymerization was firstly utilized in reverse osmosis by Cadotte et al.,5 it has been widely used and becomes a research hotspot in membrane field.6 The thin selective layer is formed by a reaction between two monomers of acyl chloride and an amine on the top of porous support substrate,7–9 and the common monomers for interfacial polymerization are piperazine (PIP) and trimesoyl chloride (TMC).10 The main advantage of NF membranes compared with ultrafiltration membranes is their surface charge, which means solutes even smaller than pore size of membrane can be rejected according to Donnan exclusion.11,12 However, NF membranes have the drawbacks of low water flux and poor anti-fouling property, which have become the major restriction for application.13 Many efforts have been taken by researchers from academic and industrial sectors, they found the properties of PA membranes can be promoted by surface modification with hydrophilic and anti-fouling materials.14,15
Recently, the incorporation of nanoparticles in the top of layer polymer matrix has become a focal point, which can improve not only anti-fouling property but also water permeability.16 For example, zeolite incorporated into the active layer of a PA membrane has been reported by researchers, water permeability was improved without significantly affecting on salt retention as a result.17,18 Also, SiO2 nanoparticle, an inorganic additive, having the characteristics of small particle size and a mass of hydrophilic groups on its surface, were incorporated into the active layer of NF membrane.19 Membrane fouling has become a main problem of PA membranes, therefore, many attempts have been made to change the situation.14,20 The fabrication of antibacterial nanocomposite membrane has been reported, to be specific, silver nanoparticles were immobilized onto the active layer of nanofiltration membrane.21 Graphene oxide (GO), a two-dimensional carbon material with impressive properties such as compatibility, high hydrophilicity and satisfying mechanical property, has attracted considerable attention.22 However, in most of these studies, the stability of membrane has become a problem, impeding further advance of membrane properties.
Graphitic carbon nitride (g-C3N4) is a kind of two-dimensional, graphene-analogue material with series of unique properties such as thermal and chemical stability, outstanding uniformity, as well as facile synthesis.23 g-C3N4 nanosheets (CNs) have porous structure, like graphene, implying water molecules are allowed to freely pass through.24 Therefore, researchers realized that CNs have application potential for preparation of membranes as additives.25 Additionally, the existence of uncondensed NH and NH2 groups in structure of CNs provides a chance to make nanofiltration membranes more stable,26 because a reaction between acyl chloride and an amine can occur easily. So, CNs with unique features are satisfying materials to prepare high-performance membranes, especially nanofiltration membrane. However, rare reports have involved the utilization of CNs in nanofiltration membranes so far.
In the present study, CNs were first incorporated into the active layer of nanofiltration membranes utilizing the method of interfacial polymerization. PIP and TMC were key components in aqueous phase and organic phase respectively for the reaction of interfacial polymerization. The properties of the PA/CNs membranes such as water flux, salt retention and anti-fouling were systematically studied and made comparisons with margin membrane. The novel nanofiltration membranes were characterized by FT-IR, XRD, SEM, AFM, TGA and water contact angle measurements.
2. Experimental
2.1. Materials
Polyethersulfone (PES) was supplied by BASF Co. (German). Poly(ethylene glycol) (PEG, MW = 2000 g mol−1) was purchased from Kermel Chemical Reagent Co. (Tianjin, China). N,N-Dimethyl formamide (DMF) and n-heptane were obtained from Benchmark Chemical Reagent Co. (Tianjin, China). Trimesoyl chloride (TMC) was supplied by Heowns Biochemical Technology Co. (Tianjin, China). Sodium sulfate (Na2SO4), humic acid (HA), piperazine (PIP) and melamine were purchased from Guangfu Fine Chemical Research Institute (Tianjin, China). Bovine serum albumin (BSA) was provided by Lanji Science & Technology Co. (Shanghai, China).
2.2. Preparation of CNs
CNs were prepared according to the thermal oxidation “etching” process.27 Melamine was heated at 550 °C for 4 h under air condition, and the processes, cooling and heating, were both well-organized with a ramp rate of 3 °C min−1. The obtained product was processed into powder in a mortar. The processed product was transferred into an open ceramic boat and left in air condition of 500 °C for 2 h with a ramp of 5 °C min−1. The resulted powder was dispersed in deionized water with a concentration of 1 mg mL−1. Then the solution was treated by ultrasound for 6 h. Finally, the product was obtained by centrifugation.
2.3. Synthesis of membrane substrate
PES ultrafiltration membranes were synthesized via phase inversion method. 16 wt% PES, 16 wt% PEG and 68 wt% DMF were mixed and heated at 60 °C for 8 h with continuous stirring until the solution became completely homogeneous. Then, the solution was put aside for removing air bubbles for overnight. The prepared solution was cast on a glass plate with a casting knife and then moved to a deionized water bath at room temperature. The prepared membranes were moved to another water bath for 24 h to leach out the residual solvents. Finally, the membranes were placed between two sheets of filter paper at room temperature for drying.
2.4. Fabrication of PA and PA/CNs membranes
The composite membranes were fabricated by interfacial polymerization on the top of PES ultrafiltration membranes. PIP and TMC were the monomers respectively in aqueous phase and organic phase. Firstly, a tailored, prepared PES substrate was installed on the bottom of a filtration cell (8200 model ultrafilter) and an aqueous solution (80 mL) of PIP (0.1 wt% in aqueous solution) was poured into the cell. Then, the aqueous solution was squeezed out from the cell for 25 minutes at 2 bar, and the solution, in the cell, was drained at the same time. After that, the excess aqueous solution on the surface of membrane was removed by an electric fan at room temperature until no visible aqueous solution remained. The dried membranes were drenched into n-heptane solution containing 0.15 wt% TMC monomer for 100 s. The next step was to maintain the membranes at room temperature for 25 minutes for further polymerization. At last, the resulted fresh membranes were immersed in deionized water for stopping the reaction. Also, the membranes were stored in deionized water.
The PA/CNs membranes were fabricated by the same method as above, except that CNs (0.0025 wt% in aqueous solution) were dispersed in PIP solution. In order to reach a good dispersion of CNs on the surface of membranes, the solution, containing PIP and CNs, was treated by a probe sonicator for 10 minutes. The schematic of separation process of PA/CNs was shown in Fig. 1.
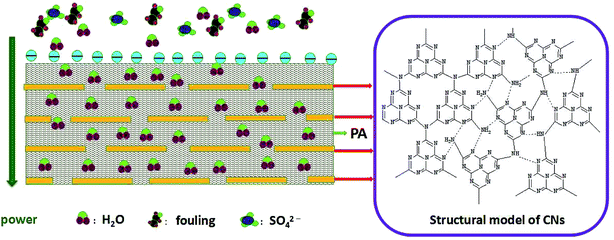 |
| Fig. 1 The schematic of water–salt separation process and antifouling process of PA/CNs membrane. | |
2.5. Characterization
The Fourier transform infrared spectroscopy (FT-IR, Bruker Vertex 80 V) was employed to characterize the chemical structure of the CNs, reacted CNs with TMC and membranes. The morphologies containing surface and cross-section of membranes and the dispersion of CNs were investigated with SEM (FE-SEM, Nanosem 430). The surface roughness of the prepared membranes was studied by using atomic force microscopy (AFM, CSPM5000). X-ray diffraction (XRD, D8, Bruker) was utilized to analyze the crystalline structure of the CNs and membranes. The contact angle measuring instrument was used to measure the surface hydrophilicity of the membranes, and to minimize experimental errors, the average value was obtained by measuring five random locations. Thermal gravimetric analysis (TGA, Netzsch 204 F1) was investigated under a nitrogen atmosphere at a heating rate of 10 °C min−1 to testify the thermal stability of membranes. Surface charge of membranes was examined by zeta potential analysis. 1.0 mmol L−1 KCl aqueous solution was utilized for the determination of the zeta potential, which was measured at pH = 7.
2.6. Membrane performance testing
All the membrane experiments were carried out in the same filtration cell mentioned in Subsection 2.4. The effective area of the cell was 28.7 cm2. The flux and retention of all membrane tests were conducted at room temperature and 2 bar. To obtain a steady flux, each membrane was compacted at 2.5 bar with a feed solution for 30 minutes before testing. The flux was calculated according to following equation: |
 | (1) |
where J is the flux (L m−2 h−1), ΔV is the permeation volume (L) in a time scale Δt (h). A is the effective area of membrane (m2). The rejection ratio of salt solution was calculated by the following equation: |
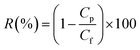 | (2) |
where, Cp and Cf are the concentrations of the feed and permeate solutions, respectively.
2.7. Antifouling property testing
The antifouling property of the membranes was investigated with BSA and HA solutions respectively. The concentration of BSA and HA solutions were both 1 g L−1. The fouling experiments were all carried out for 30 h at room temperature and the permeate flux was recorded every 30 minutes. After the fouling experiments, all the fouled membranes were washed for 1 h using pure water. Then, the filtration experiments were conducted with pure water for 2 h and the water flux was recorded every 30 minutes. Finally, the antifouling property of PA membrane and PA/CNs membrane was made comparison.
3. Results and discussion
3.1. Characterization of CNs
XRD was used to investigate the crystalline structure of CNs and the result was shown in Fig. 2a. Two characterized peaks of CNs were observed at 2θ = 27.1° and 2θ = 12.5° respectively and these diffraction peaks confirmed the synthesis of CNs exactly.25
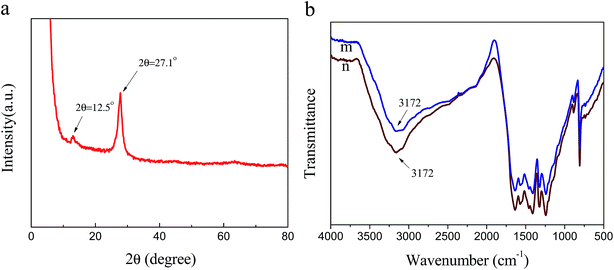 |
| Fig. 2 (a) XRD spectra of CNs and (b) FT-IR spectra of (n) CNs and (m) reaction products of CNs and TMC. | |
The chemical structure of CNs was investigated by FT-IR and the results were shown in Fig. 2b (n). Due to the out-of-plane bending vibration of tri-s-triazine ring system,27 a sharp peak at 805 cm−1 was appeared. Trigonal N–(C)3 (full condensation) stretching and bridging C–NH–C (partial condensation) stretching vibrations could be characterized by the absorption bands in the scope of 1100–1700 cm−1.26 A distinct band was presented at 3172 cm−1, which was the characterized band of terminal NH and NH2 groups.28 Additionally, the chemical structure of reaction products of CNs and TMC was shown in Fig. 2b (m). Compared with pure CNs, the characteristic peak intensity at 3172 cm−1 of reacted CNs declined confirming occurrence of chemical reaction between CNs and TMC.
3.2. XRD analysis of NF membranes
XRD patterns of CNs, PA membrane and PA/CNs membrane were depicted in Fig. 3a. Obviously, the main characteristic peaks of PA membrane and PA/CNs membrane were same except that PA/CNs membrane emerged a new peak at 2θ = 27.1°. The appearance of characteristic peak at 2θ = 27.1° confirmed the existence of CNs on the surface of PA/CNs membrane.
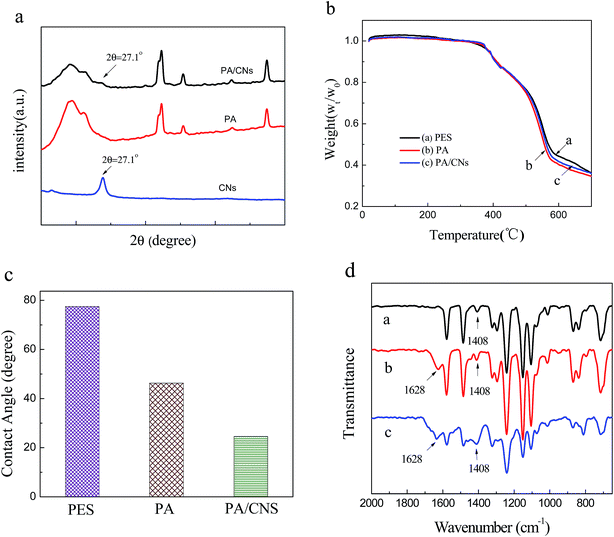 |
| Fig. 3 (a) XRD spectras of CNs, PA membrane and PA/CNs membrane; (b–d) TGA curves, water contact angle and FT-IR spectras of PES substrate, PA and PA/CNs membranes. | |
3.3. Thermal property of membranes
TGA analysis was adopted to characterize the thermal stability of the PES substrate, PA membrane and PA/CNs membrane by measuring the weight loss. The experiment was under a nitrogen atmosphere at a heating rate of 10 °C min−1. As shown in Fig. 3b, the weight loss of all membranes was stable up to 360 °C, manifesting good thermal stability and complete elimination of water. The backbone of all membranes was degraded in the range from 360 °C to 800 °C, and the degradation trend was similar, indicating the incorporation of CNs did not change thermal stability of membranes.
3.4. Contact angle measurement
The hydrophilicity of membranes was evaluated by water contact angle analysis and the results were shown in Fig. 3c. The water contact angle of neat PES substrate was 77.5°. However, it was found that the water contact angle of PA membrane was 46.3°. The explanation was as follows: unreacted amine groups in structure of PIP could decrease the water contact angle, improving the hydrophilicity of membranes.12 With the addition of CNs (0.0025 wt% in aqueous solution) into active layer of PA membrane, the water contact angle was decreased from 46.3° to 24.6°. The phenomenon could be explained that CNs were more hydrophilic materials than PA. Furthermore, during the formation of PA/CNs membrane, hydrophilic CNs might migrate towards the top layer to reduce interface energy, resulting in water was easily adsorbed by PA/CNs membrane. Also, the presence of amine groups in structure of CNs could reduce the water contact angle of membrane.12 Therefore, the incorporation of CNs into PA membrane increased surface hydrophilicity.
3.5. FT-IR analysis of the membrane surface
The chemical structure of PES substrate, PA membrane and PA/CNs membrane was characterized using FT-IR and the results were shown in Fig. 3d. The reaction of interfacial polymerization between PIP and TMC was occurred on the surface of PES substrates, because the characteristic peak of –CONH– was appeared at 1628 cm−1.29 Compared with PA membrane, the intensity of characteristic peak of PA/CNs membrane at 1628 cm−1 and 1408 cm−1 was enhanced distinctly. The appearance of the phenomenon was ascribed to the incorporation of CNs into PA membrane, and a reaction between amine groups in structure of CNs and TMC.
3.6. SEM and AFM analysis
The surface and cross-section of membranes were examined by SEM and the images were shown in Fig. 4. Fig. 4a depicted the surface morphology of pristine PA membrane. Like the common morphology for traditional PA membrane, the surface of the PA membrane was compact and nodular,30 which was the result of cross-linking reaction between PIP and TMC. Fig. 4b depicted the surface morphology of PA/CNs membrane. With the addition of CNs into PA membrane, the surface structure was changed significantly. Due to the existence of CNs in cross-linking process, CNs were immerged in PA active layer, resulting in the change of surface formation. Fig. 4c and d depicted the contaminated membranes of PA and PA/CNs with BSA. Compared with uncontaminated PA membrane, the surface of contaminated PA membrane was denser and had huge lumps on surface. The phenomenon demonstrated that a mass of BSA attached on surface of membrane. Compared with uncontaminated PA/CNs membrane, the surface structure of contaminated PA/CNs membrane changed slightly. Fig. 4e and f showed the contaminated membranes of PA and PA/CNs with HA. Compared with uncontaminated PA membrane, the surface structure of contaminated PA membrane changed significantly. The surface of contaminated PA membrane adsorbed many large lumps and was not as smooth as before resulting in aggravation of fouling. However, the surface of contaminated PA/CNs membrane had only a small mount of contaminant. The results confirmed that the incorporation of CNs into PA layer improved the antifouling property. From Fig. 4g and h, the finger-like porous support of PES and the thin selective layer were both observed in images of pristine PA and PA/CNs membranes. The images of cross-section of the PA and PA/CNs membranes proved the membrane structure was stable without appearing collapsion and active layer on the top of PES substrate was fabricated. In addition, compared with PA active layer, the active layer thickness of PA/CNs had no significant change and was about 600–700 nm.
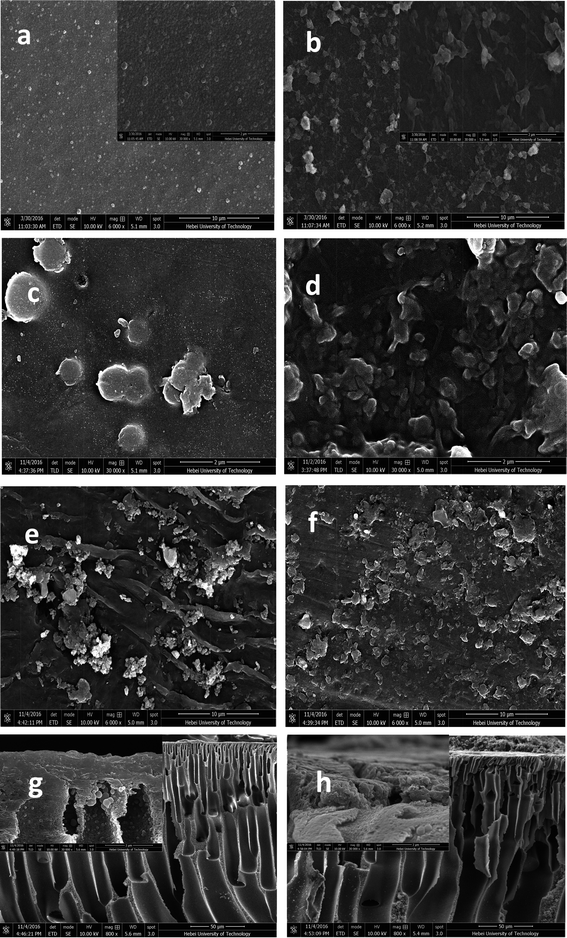 |
| Fig. 4 SEM images of (a and b) the uncontaminated surface, (c and d) the contaminated surface with BSA, (e and f) the contaminated surface with HA, (g and h) the cross-section of PA and PA/CNs membranes. | |
Fig. 5 showed the three-dimension AFM images of membranes. Root mean square (RMS) values were calculated for a scan area of 5 μm × 5 μm and the high RMS values represented high surface roughness. Compared with unmodified PA membrane, the roughness of PA/CNs increased significantly. The increase of roughness of PA/CNs membrane was attributed to the incorporation of CNs into active layer. Although, the anti-fouling property was weak for rougher surface of membrane, the incorporation of CNs into PA membrane could improve the hydrophilicity, enhancing the membrane fouling resistance.12 The results of experiment proved the antifouling property of membrane was improved by incorporating CNs.
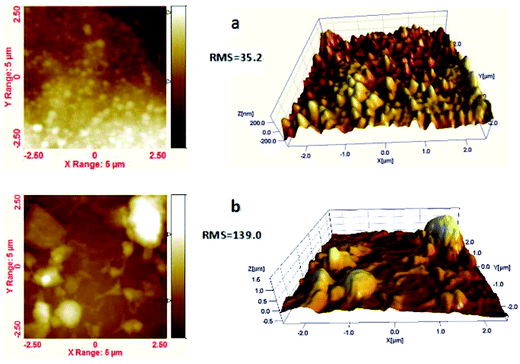 |
| Fig. 5 AFM images of (a) PA membrane and (b) PA/CNs membrane. | |
3.7. Surface charge property of membranes
The surface zeta potential was used to measure the surface charge of membranes at pH = 7 as shown in Fig. 6. The surface charge of membranes was a crucial parameter to separation and antifouling properties, especially for salt and charged foulings. From Fig. 6, the membranes of PA and PA/CNs were both negatively charged and the intensity of the PA/CNs membrane was high than PA membrane. A possible explanation was that CNs was negatively charged. The incorporation of CNs into PA active layer improved the surface negative charge density.
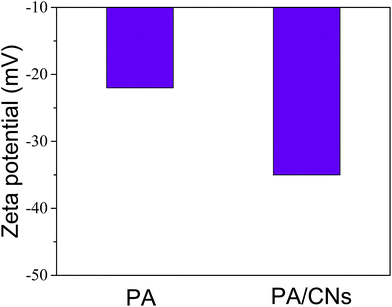 |
| Fig. 6 Zeta potential at pH = 7 of PA and PA/CNs membranes. | |
3.8. Effects of preparation conditions on performances
3.8.1. The concentration of CNs. Fig. 7 described the effect of concentration of CNs incorporated into the membranes on pure water flux and salt rejection. The conditions of interfacial polymerization for the prepared membranes were same except the concentration of CNs in aqueous solution, and the same conditions were as follows: 0.1 wt% PIP in the aqueous phase; the immersion time of membranes in PIP solution for 25 minutes; 0.15 wt% TMC in the organic phase; reaction time for 100 s and curing at room temperature for 25 minutes. In this study, different contents of CNs in aqueous solution from 0 to 0.00375 wt% were incorporated into PA layer. The results demonstrated that water flux was increased sharply while the salt rejection was decreased with the concentration of CNs increasing gradually. The high permeability of the membranes might be attributed to the superior hydrophilicity. CNs were hydrophilic materials, which contributed to water molecules enter, promoting the water permeability. Also, the incorporation of CNs into the composite membranes provided a large number of hydrophilic sites at the membranes surface, accelerating the sorption of water into the membranes. Additionally, the incorporation of CNs decreased the interaction of polymer chains, resulting in loose of membrane surface.
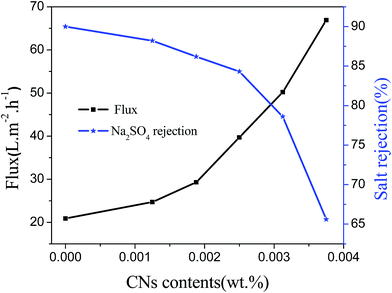 |
| Fig. 7 Pure water flux and salt rejection for the series of PA/CNs membranes at different concentrations of CNs in aqueous solution and 2 bar. | |
From Fig. 7, it was seen that the salt rejection of the PA/CNs membranes was reduced compared with pristine PA membranes. Obviously, when the incorporated amount of CNs was less 0.0025 wt%, the pure water flux was improved and the salt rejection maintained high level with the increase of CNs. However, when the incorporated amount of CNs was more than 0.0025 wt%, the pure water flux was improved and the rate of salt rejection was declined dramatically. The phenomenon could be explained by three reasons. Firstly, the hydrophilicity of the composite membranes was improved, which promoted the mass transfer. Secondly, with the incorporation of CNs into PA membranes, the process of interfacial polymerization was changed, appearing different surface structure compared with PA membrane. The change made the PA membranes loosen and caused defects of membrane formation because of the problem of compatibility between organic and inorganic materials. Thirdly, zeta potential analysis proved that the incorporation of CNs in PA active layer could improve the density of negatively charge. The improvement of negatively charge intensity was helpful for rejection of Na2SO4 due to Donnan exclusion. When the CNs concentration in aqueous solution was less 0.0025 wt%, the effect of CNs incorporated into PA membranes was insignificant on membrane formation. Therefore, the salt rejection of the membranes changed negligibly. On the contrary, when the CNs concentration was over 0.0025 wt%, the salt rejection of the membranes was decreased distinctly.
3.8.2. The immersion time of membranes in PIP solution. Fig. 8 showed the effect of immersion time of membranes in PIP solution on salt rejection and water flux. The conditions of the reaction were as follows: 0.1 wt% PIP and 0.0025 wt% CNs in the aqueous phase; 0.15 wt% TMC in the organic phase; the reaction time for 100 s and curing at room temperature for 25 minutes. The immersion time of membranes in PIP solution respectively was 10 minutes, 15 minutes, 20 minutes, 25 minutes and 30 minutes. When the immersion time of membranes in PIP solution was increased gradually from 10 to 20 minutes, the water flux was decreased while the salt rejection was opposite. When the immersion time of membranes in PIP solution was from 20 to 30 minutes, the changes of water flux and salt rejection were slight. The phenomenon could be explained that the adsorption of PIP into membranes needed a process, which meant the amount of PIP in membranes was increased gradually as the extension of time and reached saturation eventually. At low PIP content, the crosslinking rate of the active layer was inferior, resulting in loose of PA active layer. According to size exclusion mechanism, the loose surface of membrane had low reject rate for Na2SO4. Additionally, the low crosslinking rate of active layer made the compact degree of PA and CNs low, thus instability of membrane surface. As the results, numerous defects occurred due to the unstable membrane surface. With the extension of time, the crosslinking density was increased, and membrane structure became more compact and stable. Naturally, the water flux was reduced and the salt rejection was improved. When the filtration time was from 20 to 30 minutes, the adsorption and desorption of PIP reached a equilibrium, which indicated that the amount of PIP in membrane was saturated. Therefore, to prepare a membrane with good performance, the filtration time must be more than 20 minutes.
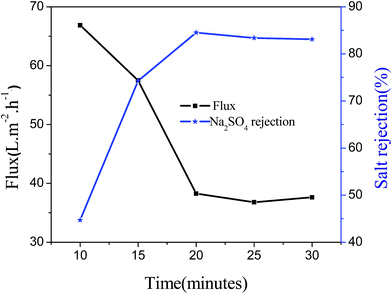 |
| Fig. 8 Pure water flux and salt rejection for the series of PA/CNs membranes at different immersion time of membranes in PIP solution and 2 bar. | |
3.8.3. Reaction time. Fig. 9 described the effect of reaction time on water flux and salt rejection. The conditions of the reaction were as follows: 0.1 wt% PIP and 0.0025 wt% CNs in the aqueous phase; 0.15 wt% TMC in the organic phase; the membranes in PIP solution for 25 minutes and curing at room temperature for 25 minutes. The time of reaction was 60–120 s. When the reaction time was from 60–100 s, the water flux was decreased while the salt rejection was increased. When the reaction time was from 100–120 s, the water flux and the salt rejection were both decreased. The phenomenon was because when the reaction time was short, with increasing of reaction time gradually, the degree of crosslinking was improved, which meant the active layer became more compact. Besides, with the reaction time improved, the thickness of active layer was increased prolonging the time through membrane surface of water. So the water flux was decreased while the salt rejection was increased. When the reaction time was overlong, the density of active layer was too large to jam water channels. Although, the incorporation of CNs into membranes could promote the water flux, the method produced a great many defects due to the bad compatibility between organic materials and inorganic materials. When the permeability was small, the defects of membranes could not be ignored. Because when the membranes were too dense, the defects became a kind of main water channel, however, which had no the capacity to salt rejection. Therefore, when the reaction time was overlong, the water flux and salt rejection were both decreased.
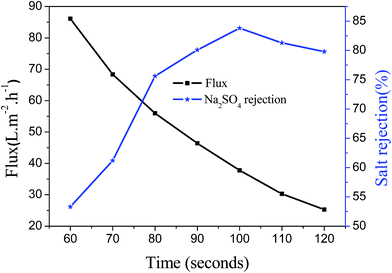 |
| Fig. 9 Pure water flux and salt rejection for the series of PA/CNs membranes at different reaction time and 2 bar. | |
3.9. Antifouling performance of membranes
It is widely accepted that membrane fouling has become a crucial problem in practical application, causing great losses. To testify the improvement of fouling resistance, aqueous solutions of a BSA and a humic acid were used to permeation tests.
Fig. 10 showed the comparision of flux between PA membrane and PA/CNs membrane with the increase of time. When membranes surface were exposed to a BSA solution, PA/CNs membrane flux had a sharp decline at first and then kept stable, while the PA membrane decreased continuously in flux with time. When membranes surface were exposed to a humic acid solution, PA/CNs membrane flux and PA membrane flux both had a sharp decline at first and then kept stable. However, the flux decline ratio of PA/CNs membrane was lower than PA membrane. Obviously, the flux decline ratio of PA/CNs membrane always kept lower than PA membrane and recovery ratio of PA/CNs membrane was high than PA membrane, which demonstrated the antifouling property of membranes were improved by the incorporation of CNs. Obviously, every membrane exposed in BSA and HA aqueous solution exhibited a sharp decline of flux due to the membrane fouling and concentration polarization. After several hours, the flux of membranes kept stable, because the adsorption and desorption of membranes to foulings reached an equilibrium. The antifouling property of the PA/CNs membrane might be attributed to the hydrophilicity of membrane surface.31 According to the solution-diffusion theory,32 the increase of membrane hydrophilicity could improve water molecular movement, enhancing water flux and fouling resistance.33 PA/CNs membrane had a more hydrophilic surface, as confirmed by contact angle analysis (Fig. 3c), so the antifouling property of PA/CNs membrane was better than PA membrane. In addition, zeta potential analysis confirmed that the negatively charge density of PA/CNs membrane was high than PA membrane. The BSA and HA were both negatively charged at pH = 7. Negatively charged BSA and HA were difficult attached on negatively charged surface of membrane due to the electrostatic repulsive interaction. Therefore, the antifouling property of PA/CNs was better than PA membrane.
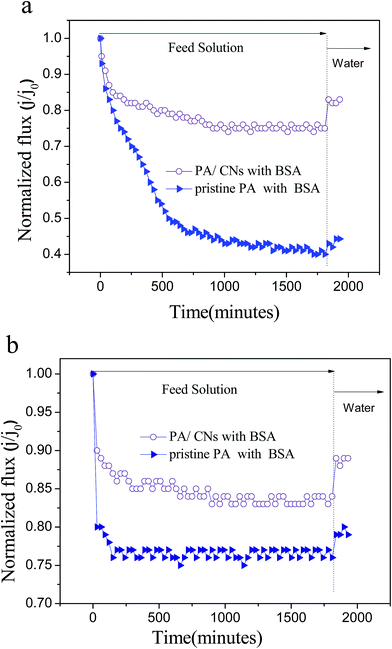 |
| Fig. 10 Characterization of PA and PA/CNs membranes with (a) BSA solution and (b) HA solution. | |
4. Conclusion
A novel composite NF membrane with the incorporation of synthesized CNs were prepared by interfacial polymerization. Different concentrations of CNs in aqueous solution, the immersion time of membranes in PIP aqueous solution and reaction time were investigated respectively. The optimum PA/CNs membrane was prepared under the conditions of 0.0025 wt% CNs in aqueous solution, the membrane in PIP solution for 25 minutes and the reaction time of interfacial polymerization for 100 s. The results of experiment indicated that performance of the optimum PA/CNs membrane was improved significantly compared to margin membrane. For example, water flux of membranes were increased from 20.9 L m−2 h−1 to 37.6 L m−2 h−1 at 2 bar while salt rejection of Na2SO4 was changed slightly. Additionally, under same conditions, the antifouling property of PA/CNs membrane to bovine serum albumin (BSA) and humic acid (HA) was better than PA membrane. The results of characterization indicated that CNs were uniformly dispersed on the surface of membrane. Additionally, with the incorporation of CNs, hydrophilicity, density of negatively charge, roughness and thermal stability of membrane were all improved. The results of characterization and experiments proved the performance of NF membrane was improved with the introduction of CNs, and the materials of CNs are potential to be used further in membrane industry.
Acknowledgements
Thanks the supports by National Natural Science Fund of China (21276063, 21476059, and 21576189), Hebei Science and Technology Support Program (16273101D).
References
- M. Safarpour, A. Khataee and V. Vatanpour, J. Membr. Sci., 2015, 489, 43–54 CrossRef CAS.
- M. Mulder, Basic Principles of Membrane Technology, Kluwer Academic Publishers, Netherlands, 2nd edn, 1998 Search PubMed.
- R. W. Baker, Membrane Technology and Applications, John Wiley & Sons Ltd, Chichester, 2nd edn, 2004 Search PubMed.
- Y. L. Ji, Q. F. An, Q. Zhao, H. L. Chen and C. J. Gao, J. Membr. Sci., 2011, 376, 254–265 CrossRef CAS.
- J. E. Cadotte, K. E. Cobian, R. H. Forester and R. J. Petersen, NTIS Report no. PB-253193, 1976.
- W. J. Lau, A. F. Ismail, N. Misdan and M. A. Kassim, Desalination, 2012, 287, 190–199 CrossRef CAS.
- N. Widjojo, T. S. Chung, M. Weber, C. Maletzko and V. Warzelhan, J. Membr. Sci., 2011, 383, 214–223 CrossRef CAS.
- I. J. Roh, J. Membr. Sci., 2002, 198, 63–74 CrossRef CAS.
- A. P. Korikov, P. B. Kosaraju and K. K. Sirkar, J. Membr. Sci., 2006, 279, 588–600 CrossRef CAS.
- D. H. Wu, Y. F. Huang, S. C. Yu, D. Lawless and X. S. Feng, J. Membr. Sci., 2014, 472, 141–153 CrossRef CAS.
- B. V. Bruggen, A. Koninckx and C. Vandecasteele, Water Res., 2004, 38, 1347–1353 CrossRef PubMed.
- M. R. S. Kebria, M. Jahanshahi and A. Rahimpour, Desalination, 2015, 367, 255–264 CrossRef CAS.
- D. Rana and T. Matsuura, Chem. Rev., 2010, 110, 2448–2471 CrossRef CAS PubMed.
- B. X. Mi and M. Elimelech, J. Membr. Sci., 2010, 348, 337–345 CrossRef CAS.
- S. Bano, A. Mahmood, S. J. Kim and K. H. Lee, J. Mater. Chem. A, 2015, 3, 2065–2071 CAS.
- M. Safarpour, V. Vatanpour, A. Khataee and M. Esmaeili, Sep. Purif. Technol., 2015, 154, 96–107 CrossRef CAS.
- N. Ma, J. Wei, R. Liao and C. Y. Tang, J. Membr. Sci., 2012, 405, 149–157 CrossRef.
- S. J. Xia, L. J. Yao, Y. Zhao, N. N. Li and Y. Zheng, Chem. Eng. J., 2015, 280, 720–727 CrossRef CAS.
- L. M. Jin, S. L. Yu, W. X. Shi, X. S. Yi, N. Sun, Y. L. Ge and C. Ma, Polymer, 2012, 53, 5295–5303 CrossRef CAS.
- D. H. Shin, N. Kim and Y. T. Lee, J. Membr. Sci., 2011, 376, 302–311 CrossRef CAS.
- S. Y. Lee, H. J. Kim, R. Patel, S. J. Im, J. H. Kim and B. R. Min, Polym. Adv. Technol., 2007, 18, 562–568 CrossRef CAS.
- C. Xu, A. Cui, Y. Xu and X. Fu, Carbon, 2013, 62, 465–471 CrossRef CAS.
- Y. Wang, X. C. Wang and M. Antonietti, Angew. Chem., Int. Ed., 2012, 51, 68–89 CrossRef CAS PubMed.
- D. E. Jiang, V. R. Cooper and S. Dai, Nano Lett., 2009, 9, 4019–4024 CrossRef CAS PubMed.
- K. T. Cao, Z. Y. Jiang, X. S. Zhang, Y. M. Zhang, J. Zhao, R. S. Xing, S. Yang, C. Y. Gao and F. S. Pan, J. Membr. Sci., 2015, 490, 72–83 CrossRef CAS.
- B. V. Lotsch, M. Doblinger, J. Sehnert, L. Seyfarth, J. Senker, O. Oeckler and W. Schnick, Chem.–Eur. J., 2007, 13, 4969–4980 CrossRef CAS PubMed.
- P. Niu, L. Zhang, G. Liu and H. M. Cheng, Adv. Funct. Mater., 2012, 22, 4763–4770 CrossRef CAS.
- S. W. Bian, Z. Ma and W. G. Song, J. Phys. Chem. C, 2009, 113, 8668–8672 CAS.
- Q. Wang, G. S. Zhang, Z. S. Li, S. Deng, H. Chen and P. Wang, Desalination, 2014, 352, 38–44 CrossRef CAS.
- M. Ghanbari, D. Emadzadeh, W. J. Lau, S. O. Lai, T. Matsuura and A. F. Ismail, Desalination, 2015, 358, 33–41 CrossRef CAS.
- C. M. Pang, P. Y. Hong, H. L. Guo and W. T. Liu, Environ. Sci. Technol., 2005, 39, 7541–7550 CrossRef CAS PubMed.
- K. P. Lee, T. C. Arnot and D. Mattia, J. Membr. Sci., 2011, 370, 1–22 CrossRef CAS.
- L. He, L. F. Dumee, C. F. Feng, L. Velleman, R. Reis, F. H. She, W. M. Gao and L. X. Kong, Desalination, 2015, 365, 126–135 CrossRef CAS.
|
This journal is © The Royal Society of Chemistry 2016 |