DOI:
10.1039/C6RA20385J
(Paper)
RSC Adv., 2016,
6, 94942-94948
Copper-free click chemistry for microdroplet's W/O interface engineering†
Received
12th August 2016
, Accepted 25th September 2016
First published on 26th September 2016
Abstract
In droplet-based microfluidic, fluorosurfactants are essential to ensure the stability of the emulsion. Beyond this primary role, fluorosurfactants can be engineered to provide droplet inner surface specific interaction characteristics with analyte present in droplet content. Despite the high potency of such capture system in terms of micro-compartmentalisation and surface/analyte ratio, only few studies have reported the use of the water/fluorinated oil interphase to immobilize target molecules. The difficult synthesis of the required functionalized fluorosurfactants needed for each application may account for this relative desertion. To make microdroplet capture approaches more straightforward, we have investigated a ready-to-use click chemistry-based approach that enables intra-droplet chemical modification. This strategy, which avoids tedious synthesis of complex fluorosurfactant, opens access to a wide variety of functional heads via copper-free click chemistry using a pre-functionalized fluorosurfactant which can be easily obtained in large scale. To demonstrate the efficiency of the click chemistry-based microdroplet surface functionalization, we have synthesized an azide fluorosurfactant capable of stabilizing microdroplets and performed a series of intra-droplet surface functionalizations by introducing fluorescent-labeled cycloalkyne derivatives in the aqueous phase. By doing so, we were able to demonstrate via polarization fluorescence that molecules from the aqueous phase could be efficiently captured at the inner droplet surface. Furthermore, we also showed that the density of azide functions at the inner surface could be adjusted by diluting the functionalized surfactant with a non-functionalized one. Fluorescence polarization analysis revealed that these dilutions result in the production of microdroplets with controlled azide surface density.
Introduction
Droplet-based microfluidics has emerged as a powerful miniaturized platform allowing the reliable production of compartmentalized micro-meter sized reactors at high throughput rates.1–3 This last decade, water-in-oil (W/O) emulsions have been extensively described as compartmentalized incubators in which complex systems can be isolated and manipulated. This technology has largely contributed to the recent developments in single-cell analysis notably for genomic studies at the single-cell level.4,5 To develop such reliable biological analysis processes, the production of monodisperse, stable and biocompatible microdroplets is required, while droplet cross-contamination should be avoided. To comply with these constrains the choice of an appropriate continuous phase with a compatible surfactant is of particular importance. The use of fluorinated oils as continuous phase is preferred as they are chemically inert; they exhibit an excellent biocompatibility and a high solubility of respiratory gases6 making them an ideal compartment to encapsulate and grow cells.7 In addition, biochemical compounds have low solubility in fluorinated oil minimizing exchange between droplets. To ensure the stability of the aqueous emulsion in this continuous phase the use of fluorosurfactants is essential. These surfactants not only prevent droplet coalescence by decreasing interfacial tension between the two phases, but are also involved in the biocompatibility of the system and in the process of molecular exchange between droplets.8 To date, most of the fluorosurfactants reported in the literature have been designed in order to avoid interaction with the droplet content and ensure the reliability of biological assays. While interactions between perfluorosurfactants and small organic molecules have been discussed in the literature,9–11 only few studies exploited the water/fluorinated oil interphase to capture molecules from the aqueous phase via specific interactions with a functionalized surfactant. Indeed, despite the favorable surface/volume ratio of microdroplets, only few example of functionalized fluorosurfactant were reported. First, Kreutz J. E. et al. synthesized a surfactant functionalized with a nitrilotriacetate nickel complex able to specifically concentrate His-tagged protein at the microdroplet inner surface. The authors used this capture system to facilitate the crystallization of His-tagged membrane protein in droplets.12 Platzman I. et al. synthesized gold nanoparticle grafted with surfactant and generated nanostructured and biofunctionalized water-in-oil droplets as tools for homing T cells.13 Finally, a fluorosurfactant tagged with a palladium catalyst has been used in Suzuki–Miyaura cross-coupling reactions in continuous flow.14 These pioneer researches illustrate the high potency of using tailored droplet inner surface to address biological and chemical issues. However, using classical synthetic approach, it is necessary to synthesize specific fluorosurfactants for each of these applications.
This difficult lengthy and tedious synthetic work somehow hampers the development of approaches relying on custom-made interphase. Interestingly, Dejournette et al. showed that the synthesis of a surfactant might not be required as the surfactant can self-assemble at the interface,15 but this strategy has not been applied to produce functional interphase. Hence, because of the synthetic feasibility all functionalized surfactants so far reported are made from short PFPE or perfluoroalkyl chain as fluoro tail16 even though such short chains are known to be less efficient for long-term droplets stabilization.
To overcome wearisome, one by one, synthesis of functional surfactants, we developed an alternative approach based on the intra-droplet chemical modification of a pre-functionalized fluorosurfactant prone to react with a variety of functional heads.
To guarantee a robust, versatile and biocompatible chemical modification method, we turned our attention towards Strain-Promoted Azide-Alkyne Cycloaddition (SPAAC) also known as copper-free click chemistry.17–21 Accordingly, we have synthesized a universal surfactant bearing an azide moiety at the end of a short hydrophilic PEG chain 1 and demonstrated the intra-droplet functionalization process by introducing into the aqueous phase fluorescent-labeled cycloalkyne derivatives 6 or 7 (sulfoCy5-BCN or TAMRA-PEG6-BCN, BCN = bicyclo[6.1.0]nonyne) (Fig. 1). By co-encapsulating both fluorescent BCN probes 6 and 7, we showed that this intra droplet modification method enables straightforward access to multi-functionalized water/oil interphase. We further demonstrated that the azide-surfactant 1 could be diluted in a non-functional one without preventing the click reaction to proceed at the droplet inner surface. This dilution enabled to fine-tune the density of azide groups at the interface, which has been successfully characterized after reaction with a fluorescent strained alkyne derivative by fluorescence polarization.
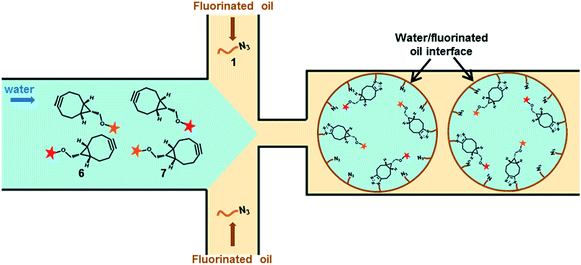 |
| Fig. 1 Microdroplets surface engineering by Cu free click chemistry. Microdroplets are produced using azide fluorosurfactant 1 as stabilizer. The co-encapsulation of strained alkyne derivatives 6 and 7, prone to react specifically with azide moiety via Strain-Promoted Alkyne Azide Cycloaddition, enables the direct chemical modification of the microdroplets inner surface. | |
Results and discussion
Design and synthesis of the azide fluorosurfactant (1)
To design the azide fluorosurfactant we referred to the optimization study described in the literature for neutral fluorosurfactant reliable for biological applications. Large perfluoropolyether (PFPE) tails have been proven efficient for a long-term stabilization and amongst them Krytox 157FSH (Dupont), a large PFPE–carboxylate derivative (MW = 6000–6500 g mol−1) is commercially available. With its charged head groups Krytox can be used as a surfactant to stabilize W/O emulsion, but lead to the non-specific adsorption of positively charged biomolecules. These non-specific interactions affect the folding of biomolecules resulting in the loss of their related activities.22 Initial efforts have been made in order to mask Krytox carboxylate charges. Clausell-Tormos et al. studied the influence of different hydrophilic heads for single-cell encapsulation and found that the use of non-ionic hydrophilic heads allowed cell culture proliferation.7 Amongst them, PFPE–PEG block copolymers have proven to be very efficient to stabilize emulsion while avoiding non-specific adsorption of biomolecules at the interface and maintaining an excellent biocompatibility.23 Since these studies, few biocompatible surfactants suitable for biological assays have been described, such as PFPE–Tris24 and PFPE–polyglycerol25 based surfactants. PFPE–PEG block copolymers remain today the reference fluorosurfactants for W/O emulsion stabilization in fluorinated systems. In accordance, we chose to synthesize a PFPE–PEG copolymer bearing an azide moiety which will be prone to react with strained alkyne derivatives. The PFPE–PEG block copolymers are usually obtained by coupling reaction between a PEG–amine derivative and the Krytox acyl chloride. Thus, to afford our azide fluorosurfactant, we first synthesized a bifunctional PEG12 linker 3 bearing both an azide and a free amine moieties (Scheme 1). The hydrophilic chain 3 was prepared by coupling two bifunctional oligoethylene glycol derivatives 4 and 5 obtained from hexaethylene glycol in 5 and 4 steps respectively (see ESI†). N-(17-Amino-3,6,9,12,15-pentaoxaheptadecan-1-yl)carbamate 5 was first activated using EDC and HOBt, and then reacted with tert-butyl N-(17-amino-3,6,9,12,15-pentaoxaheptadecan-1-yl)carbamate 4 (Scheme 1). After N-Boc deprotection, the bifunctional PEG12 derivative 3 was isolated in 81% yield over two steps. Krytox 157FSH was activated by treatment with oxalyl chloride and reacted with 3 in a mixture of CH2Cl2/Novec 7100 to afford Krytox-PEG12-azide 1. The conversion of the Krytox carboxylic acid group to the non-ionic amide derivative was investigated by 19F NMR spectroscopy as reported by Holtze et al.23 Using this analysis process, several azide fluorosurfactant batches were synthesized with a purity ranging from 75 to 85%. The NMR characterization of the azide fluorosurfactant 1 was described (see ESI, Fig. S1–S3†).
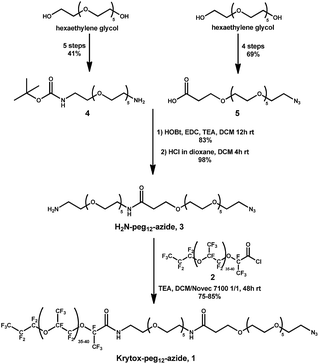 |
| Scheme 1 Synthesis of azide fluorosurfactant, Krytox-PEG12-azide (1). | |
Design and synthesis of sulfoCy5-BCN (6) and TAMRA-PEG6-BCN (7)
To evaluate SPAAC reaction at the microdroplets inner surface, two fluorescent strained cycloalkyne derivatives have been synthesized. Fluorescent BCN ((1R,8S,9S)-bicyclo[6.1.0]non-4-yn-9-ylmethyl) probes have been widely used to reveal azide functions in complex biological media and have notably been applied in the labelling of proteins and glycans, as well as in the three-dimensional visualization of living melanoma cells.26
Accordingly, we used a similar strategy to reveal the availability of azide moieties at the water/fluorinated oil interphase reflected by a concentration of fluorescence at the microdroplet inner surface. BCN was thus conjugated with sulfo-Cy5 and TAMRA fluorophores to afford two fluorescent probes 6 and 7 with different fluorescence excitation/emission properties (Fig. 2). The synthesis of the fluorescent strained alkyne derivatives is described (see ESI†).
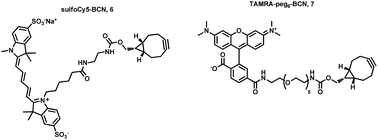 |
| Fig. 2 Structure of fluorescent probes, sulfoCy5-BCN (6) and TAMRA-PEG6-BCN (7). | |
SPAAC reaction at the microdroplet inner surface
The SPAAC reaction at the droplets inner surface was evaluated by analysing droplets of 40–50 pL prepared using Krytox-PEG12-azide 1 as surfactant in the oil phase while the fluorescent BCN derivatives 6 and 7 were introduced via the aqueous phase. After collection and incubation, droplets were reinjected and spaced by fluorinated oil in a second chip to record fluorescence signals obtained from photomultiplier tubes (PMTs). W/O emulsions were also analysed by confocal microscopy to observe fluorescence distribution across the droplets. A first emulsion prepared using 2.5% azide surfactant 1 in Novec 7500 and sulfoCy5-BCN 6 at 300 nM in Pluronic F-127 (0.01% in PBS) for aqueous phase was analysed (Fig. 3a). Signals obtained during emulsion reinjection showed a concave meniscus signal and confocal microscopy clearly indicated that the probe was located preferably at the inner surface of the water droplet. Two control experiments were performed. First, non-functionalized commercially available fluorosurfactant (008-F, Ran Biotechnologies) and fluorescent BCN derivative 6 were used to prepare the emulsion (Fig. 3b). Finally, microdroplets were generated with azide diblock surfactant 1 in the presence of sulfoCy5-alkyne fluorophore (Fig. 3c). This control fluorophore was not functionalized with a strained alkyne, and the click reaction could, therefore, not occur without copper catalyst. In both cases signals from PMT and confocal microscopy showed that fluorescence was uniform within the droplets volume confirming that the click reaction did not occur and that signal observed in experiment was the result of specific SPAAC reaction at the droplet surface. Accordingly, this first set of experiments showed that SPAAC proceeded efficiently and specifically at the azide-pre-functionalized microdroplets surface. Similar results were obtained with TAMRA-PEG6-BCN 7. SPAAC reaction only occurred when Krytox-PEG12-azide 1 was used in the presence of TAMRA-PEG6-BCN 7 as shown by the fluorescence concentration at the inner surface droplet (Fig. 4a). Using of a non-functionalized surfactant (008-F, Fig. 4b) or a non-functionalized fluorophore (TAMRA-6-CO2H, Fig. 4c) led to microdroplets with uniform fluorescence concentration confirming that the SPAAC reaction did not occur.
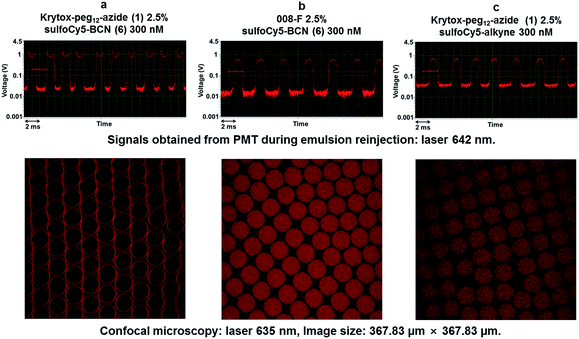 |
| Fig. 3 SPAAC reaction at the inner surface using sulfoCy5 derivatives. Conditions for droplets production: oil phase – Novec 7500; aqueous phase – Pluronic F-127 (0.01% in PBS 1×): (a) Krytox-PEG12-azide 1 2.5%/sulfoCy5-BCN 6 300 nM. (b) 008-F 2.5%/sulfoCy5-BCN 6 300 nM. (c) Krytox-PEG12-azide 1 2.5%/sulfoCy5-alkyne 300 nM. | |
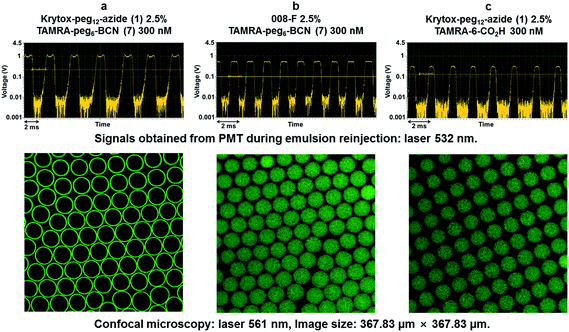 |
| Fig. 4 SPAAC reaction at the inner surface using Krytox-PEG12-azide (1) and TAMRA-PEG6-BCN (7). Conditions for droplets production: oil phase – Novec 7500; aqueous phase – Pluronic F-127 (0.01% in PBS 1×): (a) Krytox-PEG12-azide 1 2.5%/TAMRA-PEG6-BCN 7 300 nM. (b) 008-F 2.5%/TAMRA-PEG6-BCN 7 300 nM. (c) Krytox-PEG12-azide 1 2.5%/TAMRA-6-CO2H 300 nM. | |
Bi-functionalization of microdroplets inner surface
Results described above showed that the intra-droplet SPAAC reaction is efficient to prepare mono-functionalized microdroplets interphase. We next investigated the possibility offered by this approach to generate multi-functionalized interphase without prior synthesis of diverse complex fluorosurfactants. Toward this end, fluorescent probes 6 and 7 were co-encapsulated in microdroplets stabilized with Krytox-PEG12-azide 1. SulfoCy5-BCN 6 and TAMRA-PEG6-BCN 7 were diluted at 200 nM and Krytox-PEG12-azide 1 was used at 2.5% in Novec 7500 for the oil phase. Signals from PMT during emulsion reinjection and confocal microscopy showed that the fluorescence signals of both probes were co-located at the microdroplets inner surface (Fig. 5).
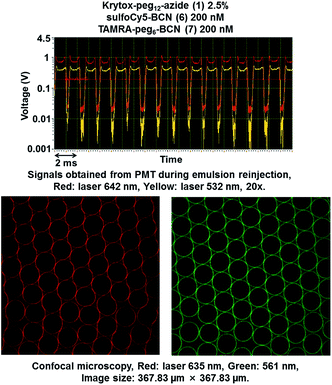 |
| Fig. 5 Double functionalization of microdroplet surface. Conditions for droplets production: oil phase – 2.5% Krytox-PEG12-azide 1 in Novec 7500; aqueous phase – sulfoCy5-BCN 6 200 nM, TAMRA-PEG6-BCN 7 200 nM in Pluronic (0.01% in PBS 1×). | |
Fine-tuning of azide surface density
To modulate the azide group surface density we decided to dilute the azide fluorosurfactant 1 in the non-functionalized fluorosurfactant 008-F. We first checked that dilution of the azide fluorosurfactant did not prohibit the click reaction at the inner surface. Three emulsions were prepared with decreasing concentrations of 1 (Fig. 6, a: 20%, b: 10% and c: 5%) in the commercial fluorosurfactant 008-F. Aqueous phase was charged with sulfoCy5-BCN 6 (300 nM) and a tracer fluorophore ACMS (1 μM) in Pluronic 0.01%. Characteristic PMT signals for surface located fluorescence were obtained for concentrations (a) (1, 20%) and (b) (1, 10%) but not for concentration (c) (1, 5%) (Fig. 6).
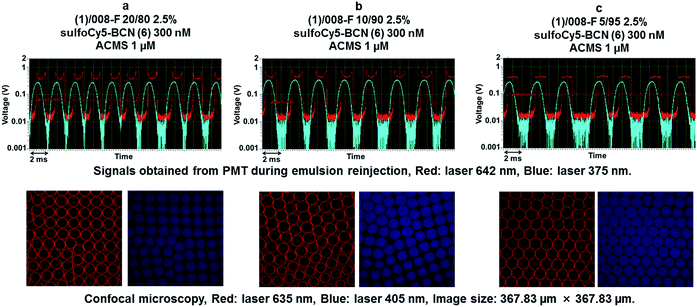 |
| Fig. 6 SPAAC reaction after dilution of azide fluorosurfactant 1 in the non-functionalized commercial fluorosurfactant 008-F. Conditions for droplets production: oil phase – 2.5% 1/008-F (20/80 to 05/95) in Novec 7500, a = 1/008-F 80/20, b = 1/008-F 10/90, c = 1/008-F 05/95; aqueous phase – a, b, c = sulfoCy5-BCN 6 300 nM, ACMS 1 μM in Pluronic (0.01% in PBS 1×). | |
However, confocal microscopy revealed that SPAAC reaction proceeded well for all conditions. In all conditions control fluorophore (ACMS) was uniformly distributed in the droplets volume as confirmed by both PMT signals and confocal microscopy.
While the above results clearly showed that the azide fluorosurfactant could be diluted in a non-functional one without preventing the click reaction, they did not enable us to determine whether this dilution led to a lower azide surface density. To investigate this particular point, we hypothesized that fluorescence polarization would increase when the fluorescent probe are captured at the microdroplet inner surface. According to this assumption, we produced microdroplets containing different surface azide densities and measured the fluorescence polarization after ensuring that the SPAAC reaction took place. Seven emulsions were prepared with increasing concentrations of 1 (0, 1, 5, 10, 20, 50 and 100%) in the non-functionalized surfactant (008-F) while maintaining the concentration of fluorescent sulfoCy5-BCN 6 probe in aqueous phase constant (250 nM in Pluronic F-127). Results of fluorescence polarization analysis are depicted in Fig. 7. Increasing concentration of diblock-azide 1 led to an enhancement of polarization fluorescence indicating that higher concentration of fluorescent probe was indeed captured at the inner surface droplet. Moreover fluorescence polarization reached a plateau at around 20% of azide surfactant 1. From this concentration, the total amount of fluorescent probe 6 encapsulated in the microdroplets has reacted with the azide moieties at the inner surface. A rough calculation considering a microdroplets volume of 44 pL and the concentration of 6 of 250 nM indicate that one droplet contains about 11 attomol of probe 6. Thus, microdroplets stabilized with a mixture of 1/008-F 20/80 contains 11 attomol of azide at their inner surface corresponding to a surface density of 1.8 nmol m−2.
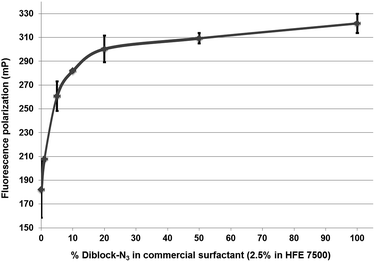 |
| Fig. 7 Fluorescence polarization for a concentration range of diblock-azide (1) in commercial fluorosurfactant (008-F). Conditions for droplets production: oil phase – 2.5% 1/008-F (0/100 to 100/0) in Novec 7500; aqueous phase – sulfoCy5-BCN 6 250 nM in Pluronic F-127 (0.01% in PBS 1×). | |
Reaction at the inner droplet surface was validated for each collected emulsions by confocal microscopy (see ESI, Fig. S4†).
A second fluorescence polarization experiment was performed by varying the concentration of sulfoCy5-BCN probe 6 while keeping a 5% ratio of Krytox-PEG12-azide 1 in the non-functionalized surfactant (008-F). The aqueous phase was charged with increasing concentrations of sulfoCy5-BCN 6 (0.01, 0.1, 1 and 10 μM in Pluronic 0.01%). Negative controls using only non-functionalized surfactant 008-F as stabilizer were also performed and fluorescence polarization remained constant (186 ± 15 mP) for each probe concentration (Fig. 8, light blue). Using the mixture 1/008-F 5/95 as surfactant, no modification of fluorescence polarization was observed between 10 and 100 nM indicating that microdroplets surface was not saturated at this concentration range (Fig. 8, dark blue). Increasing the fluorescent probe concentration to 1 μM led to a decrease of fluorescence polarization. Finally, at 10 μM negative and positive controls exhibited the same fluorescence polarization values showing that the quantity of fluorophore probe located at the inner microdroplet surface is negligible compared to the population of free fluorophore probes inside the droplet. Microdroplets interface thus appear to be saturated between 100 nM and 1 μM of free probe. This corresponds to a quantity of azide groups per droplet between 4.4 to 44 attomol.
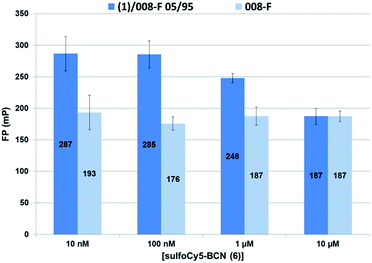 |
| Fig. 8 Fluorescence polarization for a concentration range of sulfoCy5-BCN (6) probe using a mixture of (1)/008-F 05/95 as surfactant. Conditions for droplets production: oil phase – dark blue: 2.5% 1/008-F (5/95) in Novec 7500, light blue: 2.5% 008-F in Novec 7500; aqueous phase – sulfoCy5-BCN 6 (10 nM, 100 nM, 1 μM or 10 μM), ACMS 1 μM in Pluronic (0.01% in PBS 1×). | |
The value of 4.4–44 attomol azide/droplet (0.72–7.2 nmol m−2) for 5% of azide surfactant appears coherent with the result obtained above for 20% azide surfactant 1 (11 attomol of azide/droplet). However, the density at the droplet interface for the PEG–diPFPE surfactant (similar to the one used as co-surfactant in this study) was assessed to a much higher value of 3.4 μmol m−2 by using the pendant drop tensiometry method.27 The pendant drop experiment was performed with surfactant concentrations from 1.3 mmol m−3 to 1.04 mol m−3 which represent surfactant to oil ratio (w/w) from 0.001% to 0.78%. In our microfluidic experiments 2.5% (w/w) surfactant concentration in HFE 7500 was used, representing a large excess of both 008-F and 1. The lower amount of azide groups available for click reaction could thus be explained by the competition between the two surfactants for droplet surface occupancy. Available azide groups at the surface could thus be displaced from the surface by the excess of non-functional surfactant. This could indicate that the surface coverage of the azide surfactant 1 is much smaller than that of the 008-F surfactant.
Conclusions
We have reported a microdroplets surface engineering strategy using Strain Promoted Alkyne-Azide Cycloaddition. We designed and synthesized an azide PFPE–PEG based fluorosurfactant prone to react with strained alkyne derivatives via a copper-free click reaction. By using strained alkyne conjugated beforehand with fluorophores, we demonstrated that click reaction at the microdroplets inner surface is an efficient and reliable method to generate functionalized interphase. We showed that the surface density of azide group can be adjusted by diluting the functionalized surfactant with a non-functionalized one. Fluorescence polarization analysis revealed that these dilutions result in the production of microdroplets with controlled azide surface density. This result shed light on the need of future functional fluorosurfactants development for high droplet surface occupancy. This intra-droplet surface engineering strategy circumvent the wearisome complex synthesis of functionalized fluorosurfactants and should thus open interesting prospects for the development of droplet microfluidic approaches relying on specific capture at the W/O interface.
Acknowledgements
The Medalis Labex, the icFRC (International Center for Frontier Research in Chemistry) and the SATT Connectus Alsace are gratefully acknowledged for their financial support. We thank Pascal Villa (PCBIS, Strasbourg University) for microfluidic station access and helpful discussions. Andrew Griffiths and Héloïse Cockenpot (Biochemistry Laboratory, ESPCI Paris) are also gratefully acknowledged for providing photolithography mask and for fruitful discussions. Nanofabrication of silicon wafers was performed by STnano platform (Hicham Majjad, IPCMS, Strasbourg University). Confocal microscopy analyses were performed at PIQ platform (Pharmacy Faculty, Strasbourg University) and we thank Romain Vauchelles for his help.
Notes and references
- A. B. Theberge, F. Courtois, Y. Schaerli, M. Fischlechner, C. Abell, F. Hollfelder and W. T. S. Huck, Angew. Chem., Int. Ed., 2010, 49, 5846–5868 CrossRef CAS PubMed.
- A. D. Griffiths and D. S. Tawfik, Trends Biotechnol., 2006, 24, 395–402 CrossRef CAS PubMed.
- H. Song, D. L. Chen and R. F. Ismagilov, Angew. Chem., Int. Ed., 2006, 45, 7336–7356 CrossRef CAS PubMed.
- H. N. Joensson and H. Svahn, Angew. Chem., Int. Ed., 2012, 51, 12176–12192 CrossRef CAS PubMed.
- A. Rakszewska, J. Tel, V. Chokkalingam and W. T. S. Huck, NPG Asia Mater., 2014, 6, e133 CrossRef CAS.
- J. G. Riess and M. P. Krafft, Biomaterials, 1998, 19, 1529–1539 CrossRef CAS PubMed.
- J. Clausell-Tormos, D. Lieber, J. C. Baret, A. El-Harrak, O. J. Miller, L. Frenz, J. Blouwolff, K. J. Humphry, S. Koster, H. Duan, C. Holtze, D. A. Weitz, A. D. Griffiths and C. A. Merten, Chem. Biol., 2008, 15, 427–437 CrossRef CAS PubMed.
- J. C. Baret, Lab Chip, 2012, 12, 422–433 RSC.
- P. Gruner, B. Riechers, B. Semin, J. Lim, A. Johnston, K. Short and J.-C. Baret, Nat. Commun., 2016, 7, 10392 CrossRef CAS PubMed.
- J.-M. Vincent, J. Fluorine Chem., 2008, 129, 903 CrossRef CAS.
- R. Corrêa da Costa, T. Buffeteau, A. Del Guerzo, N. D. McClenaghan and J. M. Vincent, Chem. Commun., 2011, 47, 8250 RSC.
- J. E. Kreutz, L. Li, S. Roach, T. Hatakeyama and R. F. Ismagilov, J. Am. Chem. Soc., 2009, 131, 6042 CrossRef CAS PubMed.
- I. Platzman, J. W. Janiesch and J. P. Spatz, J. Am. Chem. Soc., 2013, 135, 3339 CrossRef CAS PubMed.
- A. B. Theberge, G. Whyte, M. Frenzel, L. M. Fidalgo, R. C. R. Wootton and W. T. S. Huck, Chem. Commun., 2009, 6225 RSC.
- C. J. DeJournette, I. Kim, H. Medlen, X. Li, L. J. Vincent and C. Easley, J. Anal. Chem., 2013, 85, 10556 CAS.
- D. J. Holt, R. J. Payne and C. Abell, J. Fluorine Chem., 2010, 131, 398 CrossRef CAS.
- E. M. Sletten and C. R. Bertozzi, Angew. Chem., Int. Ed., 2009, 48, 6974 CrossRef CAS PubMed.
- R. K. V. Lim and Q. Lin, Chem. Commun., 2013, 49, 11007 RSC.
- M. King and A. Wagner, Bioconjugate Chem., 2014, 25, 825 CrossRef CAS PubMed.
- A. Borrmann and J. C. M. van Hest, Bioorthogonal chemistry in living organisms, Chem. Sci., 2014, 5, 2123 RSC.
- F. C. Pigge, Curr. Org. Chem., 2016, 20, 1902 CrossRef CAS.
- L. S. Roach, H. Song and R. F. Ismagilov, Anal. Chem., 2005, 77, 785 CrossRef CAS PubMed.
- C. Holtze, A. C. Rowat, J. J. Agresti, J. B. Hutchison, F. E. Angilè, C. H. J. Schmitz, S. Köster, H. Duan, K. J. Humphry, R. A. Scanga, J. S. Johnson, D. Pisignano and D. A. Weitz, Lab Chip, 2008, 8, 1632 RSC.
- Y.-L. Chiu, H. F. Chan, K. K. L. Phua, Y. Zhang, S. Juul, B. R. Knudsen, Y.-P. Ho and K. W. Leong, ACS Nano, 2014, 8, 3913 CrossRef CAS PubMed.
- O. Wagner, J. Thiele, M. Weinhart, L. Mazutis, D. A. Weitz, W. T. S. Huck and R. Haag, Lab Chip, 2016, 16, 65 RSC.
- J. Dommerholt, S. Schmidt, R. Temming, L. J. A. Hendriks, F. P. J. T. Rutjes, J. C. M. van Hest, D. J. Lefeber, P. Friedl and F. L. van Delft, Angew. Chem., Int. Ed., 2010, 49, 9422 CrossRef CAS PubMed.
- Q. Brosseau, J. Vrignon and J. C. Baret, Soft Matter, 2014, 10, 3066 RSC.
Footnote |
† Electronic supplementary information (ESI) available. See DOI: 10.1039/c6ra20385j |
|
This journal is © The Royal Society of Chemistry 2016 |