DOI:
10.1039/C6RA20302G
(Paper)
RSC Adv., 2016,
6, 94643-94650
Mutagenesis of precursor peptide for the generation of nosiheptide analogues†
Received
11th August 2016
, Accepted 27th September 2016
First published on 29th September 2016
Abstract
Nosiheptide, produced by Streptomyces actuosus ATCC 25421, is a typical thiopeptide biosynthesized from a precursor peptide NosM by post-ribosomal peptide synthesis. To assess which amino acid residue in the core peptide sequence of NosM is tolerated or restricted by the post-translational machinery for nosiheptide towards the change of amino acid side chains, a gene-replacement approach was implemented in the nosM-deletion strain. Site-directed mutagenesis of five cysteine residues involved in the formation of thiazoles and the third threonine residue without modification were carried out to generate ten NosM mutants with single residue replacement in the chromosome. The cysteine to serine mutations did not produce any predicted mature scaffolds, indicating that these replacements are not acceptable by the tailoring enzymes. Three out from five mutants of the third threonine residue in the core peptide of NosM led to the maturation of nosiheptide analogues retaining antibacterial activities. The present study reveals the limitation and opportunity for nosiheptide-producing strain to generate nosiheptide analogues, and provides an insight into appropriate position and amino acid side chain for precursor peptide engineering of nosiheptide biosynthesis.
Introduction
Thiopeptides are a family of highly modified sulfur-rich macrocyclic peptides which result from extensive modification of a ribosomally synthesized precursor peptide.1 While many thiopeptides exhibit impressive in vitro activities against Gram-positive bacteria, especially various drug-resistant bacterial pathogens, their use as an antibiotic treatment option has been hampered by poor aqueous solubility, which makes them to be attractive targets for engineering.2,3 Since the identification of the biosynthetic pathways for a handful of thiopeptide antibiotics in 2009, significant achievements have been made in engineering of the biosynthetic pathways of thiopeptides.4–7 The research groups of Walsh and Kelly have reported various analogues of thiocillin and thiostrepton that are generated by single residue replacement of the precursor peptides,8–16 suggesting that the biosynthetic machinery of thiopeptides is tolerant to various mutations in their linear peptides to result in new variants.
Nosiheptide from Streptomyces actuosus ATCC 25421 is a nature product from the e series of thiopeptides which belongs to the large class of ribosomally-synthesized and post-translationally-modified peptides (RiPPs).1,2 The structure of nosiheptide contains a central 2,3,5,6-tetrasubstituted pyridine core, multiple thiazole rings and an indole side ring to exhibit strong antibacterial activities through an unusual mode of action on ribosome (Fig. 1).17 However, the present utility of nosiheptide has only limited to the treatment of animal infections due to low aqueous solubility and poor bioavailability, which is a common feature of thiopeptides.6,7 To overcome the physicochemical drawbacks, generation of novel nosiheptide analogues appears to be a promising approach and has attracted significant interests. Thus far, nosiheptide intermediates have been generated from inactivating tailoring genes, such as nosA–C, nosN and nosO.18–21 Nosiheptide analogues could also be obtained in S. actuosus by supplementing the fermentation media with tryptophan analogues based on the catalytic promiscuity of NosL, a radical S-adenosyl-L-methionine-dependent L-tryptophan lyase.22–25 In addition, if the lengthy and costly routes were not considered, any designed nosiheptide analogues could possibly be prepared by chemical synthesis, in principle, since the total synthesis of nosiheptide was recently achieved through the assembly of a fully functionalized linear precursor followed by consecutive macrocyclizations.26 Given that nosiheptide arises from the modifications of a ribosomally synthesized linear precursor peptide NosM by a series of post-translational modification enzymes (Fig. 1),18 nosiheptide analogues could potentially be generated in vivo by precursor peptide mutagenesis more feasibly than chemical synthesis.27 However, the accessibility of generating nosiheptide analogues remains unknown compared to other thiopeptides, such as thiocillin and thiostrepton.8–16 Especially, the knowledge on the structural variability of the nosiheptide scaffold is not understood.
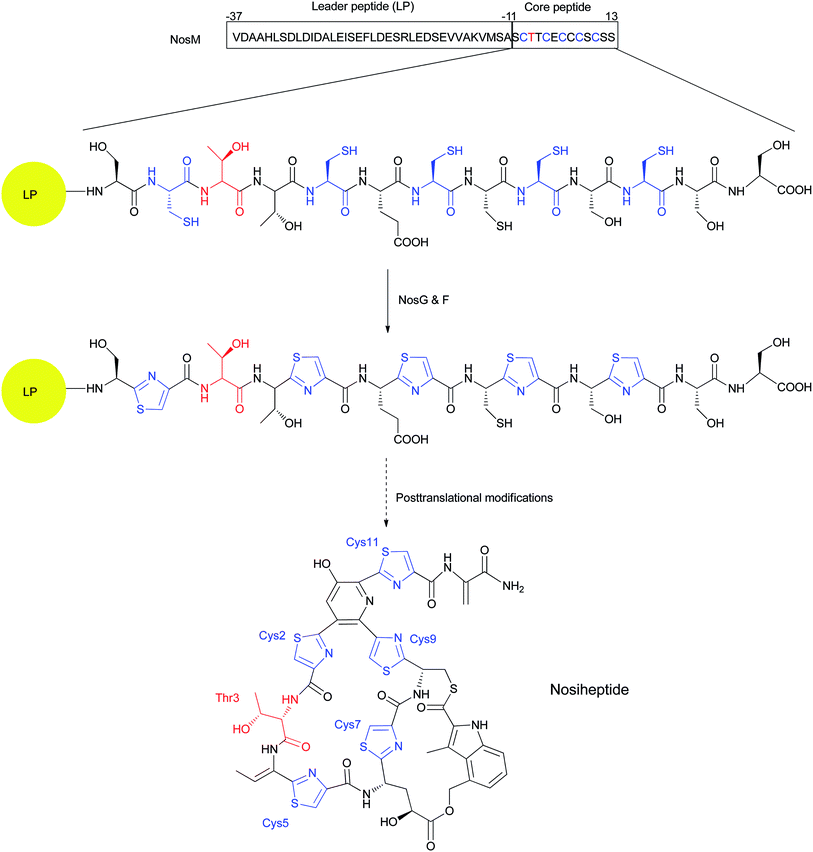 |
| Fig. 1 Chemical structure and illustrative biosynthetic pathway of nosiheptide. The Cys residues participating in the formations of thiazole rings and Thr3 are indicated in blue and red respectively. | |
The precursor peptide NosM is composed of an N-terminal leader peptide (37-aa) and a C-terminal core peptide (13-aa, SCTTCECCCSCSS) as shown in Fig. 1.18 Similar to other thiopeptides, the core peptide is processed to mature nosiheptide and the leader is cleaved at a late stage.28,29 To gain an in-depth and comprehensive understanding of the biosynthetic pathway of nosiheptide, our laboratory previously reported several key features involved in the complicated modifications and the biosynthetic regulation of nosiheptide.20,27,30–32 Our studies demonstrated that the C-terminal extended Ser13 residue of the core peptide is absolutely required in nosiheptide maturation, although only the first 12 amino acids of the core peptide are found in mature nosiheptide.27 Site-directed mutagenesis of Ser13 by a gene-replacement in the nosM-deletion strain (S. actuosus L1000, ΔnosM strain) could produce analogues with different modifications in the dehydroalanine tail.27 To compare and identify the amino acid residues to influence the post-translational modification machinery, the positions involved in multiple enzymatic transformations, such as the amino acid residues forming thiazole rings, were chosen to compare a particular residue without modification in nosiheptide scaffold by site-directed mutagenesis in this study. The present results not only revealed the tolerance or restriction of the post-translational machinery of nosiheptide biosynthesis towards precursor peptide engineering, but also provided new insights into the biosynthetic process of nosiheptide.
Results and discussion
Construction, screening and verification of NosM mutants
To explore potential tolerance of the core peptide to amino acid substitution, two types of amino acid residues in the core peptide sequence of NosM, including the cysteines involved in multiple post-translational modifications and the third threonine (Thr3) without modification, were selected for mutagenesis and ten mutants with single-site replacement of NosM were designed (Fig. 2A). The genes encoding the precursor peptide NosM with desired mutations were constructed by overlap extension and cloned into E. coli–Streptomyces shuttle vector pKC1139. After conjugation into the nosM-gene-deletion strain S. actuosus L1000, the strains were incubated with non-antibiotic ISP1 medium for 5 rounds (two days per round). Insertion mutants were selected by replicating on MS plate containing apramycin, and double-crossover took place only in those colonies sensitive to apramycin. Mutants exhibiting the double-crossover phenotype were further confirmed by colony PCR. As described in our previous report, the 539 bp PCR product demonstrated that mutated nosM genes were successfully integrated into S. actuosus L1000 chromosome (Fig. 2B).27,31
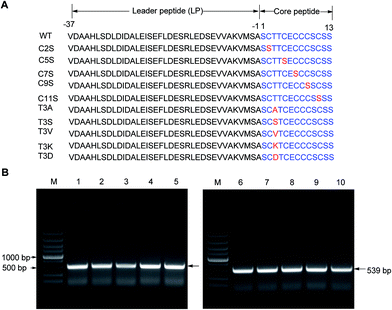 |
| Fig. 2 Construction of NosM variants. (A) Sequence comparison of wild-type NosM and its mutants. The mutated amino acid residues are indicated in red bold. (B) Confirmation of nosM mutants by PCR. Lanes (1) C2S, (2) C5S, (3) C7S, (4) C9S, (5) C11S, (6) T3A, (7) T3S, (8) T3V, (9) T3K, (10) T3D, M: DL5000 DNA marker. | |
Substitution of cysteine to serine in the core peptide of NosM
Cysteine to serine mutations have been employed in thiopeptides engineering to probe whether particular thiazole ring could be converted to an oxazole ring.9 For nosiheptide, all cysteine residues except Cys8 of NosM are expected to convert to thiazolines by cyclodehydration and then oxidized to thiazoles in mature nosiheptide by the cyclodehydratase/dehydrogenase complex Nos G & F according to proposed biosynthetic pathway (Fig. 1).18 Thus, mutations of these cysteine residues to serine could potentially produce nosiheptide analogues with oxazole rings (Fig. 3A). In this study, we first investigated whether cysteines in the core peptide of nosiheptide could be replaced by serine through a cysteine to serine scanning. Site-directed mutagenesis of nosM fragments were amplified by overlap PCR and then transferred into the chromosome of the ΔnosM strain to obtain five mutants named C2S, C5S, C7S, C9S and C11S.
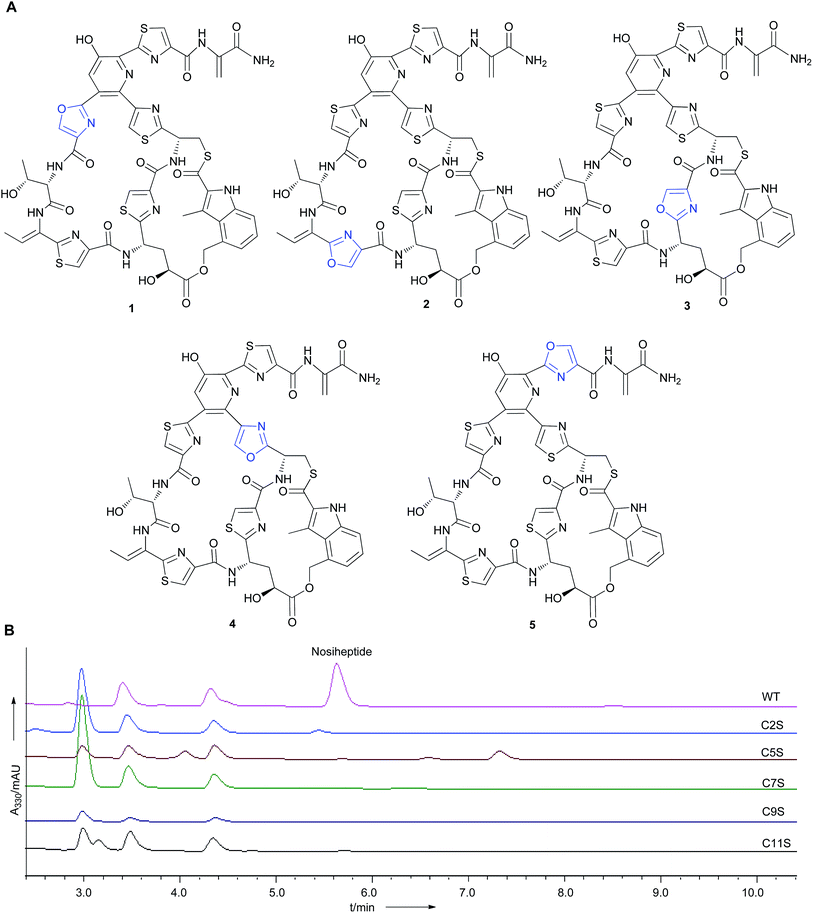 |
| Fig. 3 Predicted structures and HPLC analyses of nosiheptide analogues generated by cysteine to serine mutation. (A) Predicted structures of nosiheptide analogues generated by C2S, C5S, C7S, C9S and C11S. (B) HPLC chromatograms of the extracts from wild-type and NosM mutant strains. Unmarked peaks are unknown or unidentified components from the cultures. | |
After fermentation of the mutants, HPLC analyses indicated that only the fermentation extracts from wild type (WT) strain produced nosiheptide with a retention time of 5.8 min, and all serine substituted mutants failed to result in any mature scaffolds, demonstrating that these positions are reluctant to the alterations (Fig. 3B). Although visually detectable peaks could be observed at 2.8 min for all mutants, no nosiheptide-related compounds could be isolated due to their instability. After scaling the fermentation volumes up to 10 L, no relevant products could be obtained either. Additionally, LC-MS scanning of the extracts could not detect the molecular weights of the predicted analogues. The present results indicated that mutations of these cysteine residues are not tolerated, which could be caused by following reasons: (1) the core peptide of NosM after mutations could not be recognized by Nos G & F; (2) although the substituted serine residue could still be processed to form oxazole by Nos G & F, the linear precursor bearing an oxazole ring could not be further modified into a mature scaffold. However, at which step this residue vital for recognition remains for a thorough investigation.
Mutagenesis of Thr3 in the core peptide of NosM
As evidenced for thiocillin, amino acid substitutions at positions of the precursor peptide not involved in post-translational modifications might not disrupt the mature scaffold.8,9 Based on the amino acid sequence of NosM and the structure of nosiheptide, Thr3 in NosM is not modified by any modification enzymes in nosiheptide biosynthesis (Fig. 1). Therefore, mutagenesis of this position might not interfere with the biosynthetic machinery for nosiheptide biosynthesis. Thr3 was subsequently targeted for substitutions. Five Thr3 mutants consisting of T3A, T3S, T3V, T3D and T3K were successfully generated. The chemical structures of predicted nosiheptide analogues are presented in Fig. 4A.
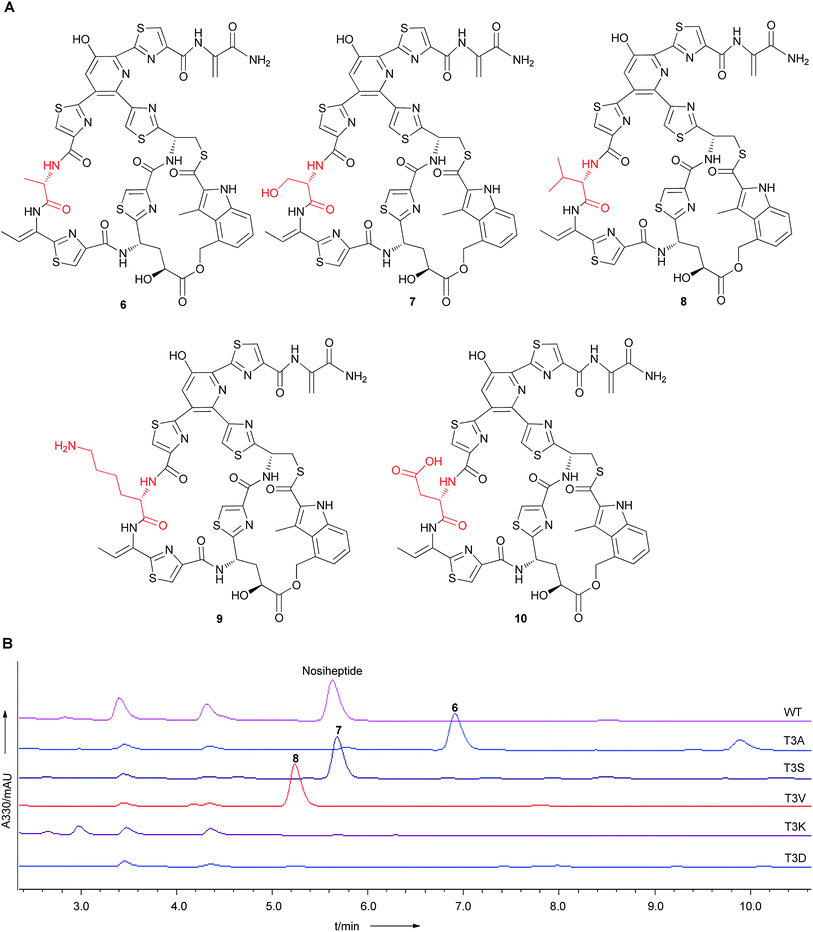 |
| Fig. 4 Predicted structures and HPLC analyses of nosiheptide analogues generated by site-directed mutagenesis of Thr3 in the core peptide of NosM. (A) Predicted structures of nosiheptide analogues generated by T3A, T3S, T3V, T3K and T3D. (B) HPLC chromatograms of the extracts from wild-type and NosM mutant strains. Unmarked peaks are unknown or unidentified components from the cultures. | |
The culture extracts of these mutant strains were evaluated for the presence of nosiheptide-related metabolites by HPLC. T3A mutant could produce a new metabolite 6 that was readily observed by HPLC with a retention time of 7.0 min (Fig. 4B). Meanwhile, both T3S and T3V mutants could generate observable metabolite respectively. Metabolite 7 from T3S mutant appeared around 5.8 min, which is very close to nosiheptide, while metabolite 8 from T3V mutant showed a retention time at 5.3 min (Fig. 4B), indicating that Thr3 variants with neutral side chains could produce predicted nosiheptide analogues. To improve the solubility of nosiheptide scaffold, T3K and T3D variants with basic or acidic side chain of the amino acids were generated, but these mutants failed to produce predicted nosiheptide analogues (Fig. 4B), presumably due to the presence of a charged group to negatively impact subsequent post-translational process.
Structural elucidation and activity analysis of nosiheptide analogues
HPLC-TOF/MS analyses of the fermentation extracts showed that metabolite 6, 7, and 8 have a molecular mass of m/z 1192.1814 [M + H]+, 1208.1530 [M + H]+ and 1220.1735 [M + H]+ (ESI Fig. S1–S3†), respectively, consistent with what expected for T3A, T3S and T3V. After scaling up the fermentation, these nosiheptide analogues were purified to greater than 95% (Fig. 5), but their production levels were substantially reduced relative to nosiheptide (Table 1). Their structures were further elucidated by 1H- and 13C-NMR (ESI Tables S1–S3 and Fig. S4–S9†). Similar to nosiheptide, the antibacterial activity of these metabolites was evaluated to be active against both methicillin-susceptible and methicillin-resistant Staphylococcus aureus (Table 1), suggesting that these variations did not disrupt the binding of these compounds to 50S ribosomal subunit adjacent to the GTPase-associated center.1 Meanwhile, it was interesting to note that, although the clog
P value of metabolite 7 is lower than others, no apparent difference on its in vitro antibiotic activity is observed compared to other compounds. Given the complex structures of nosiheptide and its analogues, the improved physiochemical property, particularly for metabolite 7, will be further evaluated in animal models to reflect true improvement of antibacterial activity in vivo. Nevertheless, these results indicated that Thr3 in the core peptide of NosM could be replaced by neutral amino acids and the mutations could produce relevant analogues retaining antimicrobial activities.
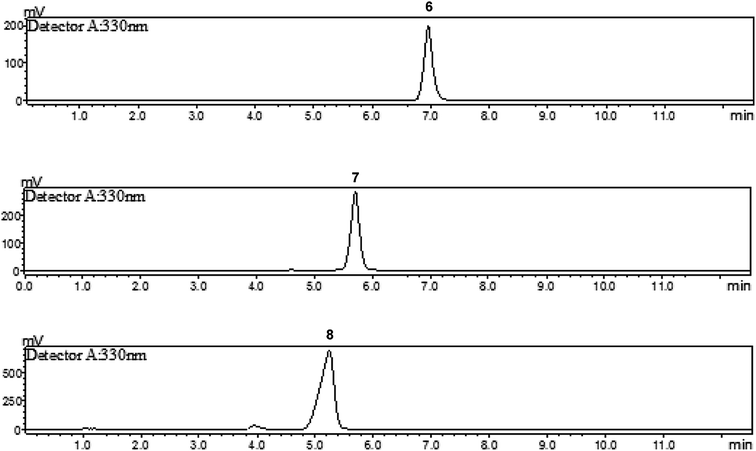 |
| Fig. 5 HPLC analyses of purified nosiheptide analogue 6, 7, and 8 from T3A, T3S and T3V mutants. | |
Table 1 Summary of production and antibacterial activity of nosiheptide and its analogues
Compound |
Production (mg L−1) |
MIC (μg mL−1) |
clog Pb |
ATCC 29213a |
ATCC 43300a |
Staphylococcus aureus subsp. aureus. clog P, values calculated with ACD/Chemsketch 2016.1. |
Nosiheptide |
12.4 |
0.05 |
0.02 |
0.72 |
6 |
3.1 |
0.02 |
0.02 |
1.43 |
7 |
5.3 |
0.05 |
0.02 |
0.38 |
8 |
2.1 |
0.05 |
0.02 |
2.31 |
Conclusions
In summary, we have probed the substrate specificity of nosiheptide post-translational machinery towards single-site mutation in the core peptide of NosM. The sites for mutagenesis are five cysteines that become thiazoles and the third threonine that undergoes no further post-translational modifications. The cysteine to serine mutagenesis did not produce any predicted mature scaffolds, demonstrating that these replacements are not accepted by the tailoring enzymes. The mutagenesis of Thr3 with five different amino acids led to the maturation of three nosiheptide analogues with neutral side chains retaining antimicrobial activities and no analogue production from two mutants with charged side chains, suggesting that Thr3 in NosM is partially mutational residue for nosiheptide biosynthesis. This study reveals the limitation and feasibility for core peptide engineering to produce nosiheptide analogues and provides new insight into appropriate positions and amino acid side chains to influence nosiheptide maturation.
Experimental
Strains, plasmids and reagents
Bacterial strains and plasmids used in this study are listed in Table 2. Primer Star Max DNA polymerase, rTaq DNA polymerase and DNA marker were purchased from TaKaRa Biotechnology Co., Ltd. (Dalian, China). Restriction enzymes and other enzymes were purchased from Fermentas (Thermo Fisher, USA). Chemicals, biochemicals and media were purchased from Sinopharm Chemical Reagent Co., Ltd. (Shanghai, China) and Oxoid Ltd. (Basingstoke, United Kingdom) unless otherwise stated.
Table 2 Strains and plasmids used and constructed in this study
Strains/plasmids |
Characteristic(s) |
Source/reference |
E. coli strain |
ET12567/pUZ8002 |
Donor strain for conjugation between E. coli and Streptomyces |
Our laboratory |
DH5α |
Host for general cloning |
TIANGEN |
![[thin space (1/6-em)]](https://www.rsc.org/images/entities/char_2009.gif) |
Streptomyces actuosus |
ATCC 25421 |
Wild type strain, nosiheptide producing |
ATCC |
L1000 |
ΔnosM strain |
Our laboratory |
C2S |
nosM variant encoding the C2S mutation |
This study |
C5S |
nosM variant encoding the C5S mutation |
This study |
C7S |
nosM variant encoding the C7S mutation |
This study |
C9S |
nosM variant encoding the C9S mutation |
This study |
C11S |
nosM variant encoding the C11S mutation |
This study |
T3A |
nosM variant encoding the T3A mutation |
This study |
T3S |
nosM variant encoding the T3S mutation |
This study |
T3V |
nosM variant encoding the T3V mutation |
This study |
T3D |
nosM variant encoding the T3D mutation |
This study |
T3K |
nosM variant encoding the T3K mutation |
This study |
![[thin space (1/6-em)]](https://www.rsc.org/images/entities/char_2009.gif) |
Staphylococcus aureus subsp. aureus |
ATCC 29213 |
Wound, sensitive to oxacillin |
ATCC |
ATCC 43300 |
Clinical isolate, resistant to methicillin |
ATCC |
![[thin space (1/6-em)]](https://www.rsc.org/images/entities/char_2009.gif) |
Plasmids |
pMD18-T |
E. coli subcloning vector |
TaKaRa |
pKC1139 |
E. coli–Streptomyces shuttle vector |
Our laboratory |
Mutagenesis of nosM
DNA manipulations in E. coli and S. actuosus were performed based on the procedures described previously.20,27,30–32 Ten mutants were generated by homologous double-crossover between the plasmid containing mutated nosM genes and the chromosome of nosM-deletion strain S. actuosus L1000. Ten pairs of overlapping complementary primers in total were designed for the mutagenesis of nosM gene (ESI Table S4†). All PCR products containing desired nucleotide changes were cloned into pKC1139 to obtain recombinant plasmids, which were then introduced into S. actuosus L1000 by E. coli–Streptomyces conjugation. The genotype of strains with apramycin-sensitive characteristics were confirmed by PCR amplification and 1.5% agarose gel electrophoresis.
Fermentation, isolation, and analysis of nosiheptide analogues
Fermentation of S. actuosus wild-type and recombinant strains and isolations of the metabolites from 10 L culture broth of each recombinant strain were carried out according to the method described previously.20,27 HPLC analyses of nosiheptide and its analogues were performed using an Agilent Eclipse Plus C18 column (4.6 × 100 mm, 3.5 μm; Palo Alto, CA, USA). The mobile phases consisted of solvent A, 0.1% TFA in water and solvent B, 0.1% TFA in acetonitrile. The sample injection volume was 20 μL. Elution was carried out with a linear gradient in the following program: 0 to 10 min, 40% B to 55% B; 10.1 to 15 min, 55% B; 15.1 to 18 min, 55% B to 60% B; 20–23 min, 40% B. The flow rate of 1 mL min−1, column temperature of 40 °C and UV detection at 330 nm were used with an Shimadzu SCL-2010A HPLC system (Shimadzu Co., Tokyo, Japan).
For preparation, the nosiheptide analogues were purified to greater than 95% purity using a 10 × 250 mm S-5 μM C18 YMC-Pack ODS-A column (YMC Co. Ltd., Kyoto, Japan) on a Waters LC150 Preparative HPLC System run at 4 mL min−1 with UV detection at 330 nm. The target metabolites eluted by 80% methanol were evaporated in vacuum and freeze-dried. Analyses of nosiheptide analogues by HPLC-MS, HR-ESI-MS and NMR were carried out according to the method described previously.20,27
Assay of antibacterial activity
The minimum inhibitory concentrations (MICs) for nosiheptide and analogues were measured by broth dilution method described previously.20 The compounds were dissolved in DMSO to produce a stock solution (1000 μg mL−1), and then serially diluted into Mueller–Hinton broth (50 μL) in a 96-well microtiter plate. Two representative Staphylococcus aureus strains ATCC 43300 (methicillin resistant) and ATCC 29213 (methicillin susceptible) were used to evaluate the antibacterial activity of nosiheptide and analogues. After the compounds were diluted, 50 μL aliquots of the test strains (about 5 × 10−5 CFU mL−1) were added to corresponding wells of the microtiter plates. After incubation at 37 °C and 150 rpm for 18–24 h, the MIC values were determined as the lowest concentration of compound that inhibits visible bacterial growth. All assays were repeated for 3 times.
Acknowledgements
This work was supported by the grants from the “111” Project from the Ministry of Education of China and State Administration of Foreign Export Affairs of China (No. 111-2-07), the National Natural Science Foundation of China (No. 81172967), the Science & Technology Pillar Program of Jiangsu Province (No. SBE201371217), and the Priority Academic Program Development of Jiangsu Higher Education Institutions (PAPD).
References
- P. G. Arnison, M. J. Bibb, G. Bierbaum, A. A. Bowers, T. S. Bugni, G. Bulaj, J. A. Camarero, D. J. Campopiano, G. L. Challis, J. Clardy, P. D. Cotter, D. J. Craik, M. Dawson, E. Dittmann, S. Donadio, P. C. Dorrestein, K. D. Entian, M. A. Fischbach, J. S. Garavelli, U. Göransson, C. W. Gruber, D. H. Haft, T. K. Hemscheidt, C. Hertweck, C. Hill, A. R. Horswill, M. Jaspars, W. L. Kelly, J. P. Klinman, O. P. Kuipers, A. J. Link, W. Liu, M. A. Marahiel, D. A. Mitchell, G. N. Moll, B. S. Moore, R. Müller, S. K. Nair, I. F. Nes, G. E. Norris, B. M. Olivera, H. Onaka, M. L. Patchett, J. Piel, M. J. Reaney, S. Rebuffat, R. P. Ross, H. G. Sahl, E. W. Schmidt, M. E. Selsted, K. Severinov, B. Shen, K. Sivonen, L. Smith, T. Stein, R. D. Süssmuth, J. R. Tagg, G. L. Tang, A. W. Truman, J. C. Vederas, C. T. Walsh, J. D. Walton, S. C. Wenzel, J. M. Willey and W. A. van der Donk, Nat Prod Rep, 2013, 30, 108–160 RSC.
- M. C. Bagley, J. W. Dale, E. A. Merritt and X. Xiong, Chem. Rev., 2005, 105, 685–714 CrossRef CAS PubMed.
- M. F. Chellat, L. Raguž and R. Riedl, Angew. Chem., Int. Ed., 2016, 55, 6600–6626 CrossRef CAS PubMed.
- F. Zhang and W. L. Kelly, Methods Enzymol., 2012, 516, 3–24 CAS.
- Q. Zhang and W. Liu, Nat Prod Rep, 2013, 30, 218–226 RSC.
- X. Just-Baringo, F. Albericio and M. Álvarez, Angew. Chem., Int. Ed., 2014, 53, 6602–6616 CrossRef CAS PubMed.
- X. Just-Baringo, F. Albericio and M. Álvarez, Mar. Drugs, 2014, 12, 317–351 CrossRef CAS PubMed.
- M. G. Acker, A. A. Bowers and C. T. Walsh, J Am Chem Soc, 2009, 131, 17563–17565 CrossRef CAS PubMed.
- A. A. Bowers, M. G. Acker, A. Koglin and C. T. Walsh, J Am Chem Soc, 2010, 132, 7519–7527 CrossRef CAS PubMed.
- A. A. Bowers, M. G. Acker, T. S. Young and C. T. Walsh, J Am Chem Soc, 2012, 134, 10313–10316 CrossRef CAS PubMed.
- T. S. Young, P. C. Dorrestein and C. T. Walsh, Chem Biol, 2012, 19, 1600–1610 CrossRef CAS PubMed.
- C. Li, F. Zhang and W. L. Kelly, Mol. Biosyst., 2011, 7, 82–90 RSC.
- C. Li, F. Zhang and W. L. Kelly, Chem. Commun., 2012, 48, 558–560 RSC.
- F. Zhang and W. L. Kelly, ACS Chem Biol, 2015, 10, 998–1009 CrossRef CAS PubMed.
- F. Zhang, C. Li and W. L. Kelly, ACS Chem Biol, 2016, 11, 415–424 CrossRef CAS PubMed.
- S. Wang, Q. Zheng, J. Wang, Z. Zhao, Q. Li, Y. Yu, R. Wang and W. Liu, Org. Chem. Front., 2015, 2, 106–109 RSC.
- S. Wang, S. Zhou and W. Liu, Curr Opin Chem Biol, 2013, 17, 626–634 CrossRef CAS PubMed.
- Y. Yu, L. Duan, Q. Zhang, R. Liao, Y. Ding, H. Pan, E. Wendt-Pienkowski, G. Tang, B. Shen and W. Liu, ACS Chem Biol, 2009, 4, 855–864 CrossRef CAS PubMed.
- Y. Yu, H. Guo, Q. Zhang, L. Duan, Y. Ding, R. Liao, C. Lei, B. Shen and W. Liu, J Am Chem Soc, 2010, 132, 16324–16326 CrossRef CAS PubMed.
- W. Liu, Y. Xue, M. Ma, S. Wang, N. Liu and Y. Chen, ChemBioChem, 2013, 14, 1544–1547 CrossRef CAS PubMed.
- H. Guo, J. Wang, Y. Li, Y. Yu, Q. Zheng, J. Wu and W. Liu, Chem. Sci., 2014, 5, 240–246 RSC.
- Q. Zhang, Y. Li, D. Chen, Y. Yu, L. Duan, B. Shen and W. Liu, Nat Chem Biol, 2011, 7, 154–160 CrossRef CAS PubMed.
- X. Ji, Y. Li, W. Ding and Q. Zhang, Angew. Chem., Int. Ed., 2015, 54, 9021–9024 CrossRef CAS PubMed.
- G. Sicoli, J. M. Mouesca, L. Zeppieri, P. Amara, L. Martin, A. L. Barra, J. C. Fontecilla-Camps, S. Gambarelli and Y. Nicolet, Science, 2016, 351, 1320–1323 CrossRef CAS PubMed.
- W. Ding, X. Ji, Y. Li and Q. Zhang, Front. Chem., 2016, 4, 27 Search PubMed.
- K. P. Wojtas, M. Riedrich, J. Y. Lu, P. Winter, T. Winkler, S. Walter and H. D. Arndt, Angew. Chem., Int. Ed., 2016, 55, 9772–9776 CrossRef CAS PubMed.
- W. Liu, M. Ma, Y. Xue, N. Liu, S. Wang and Y. Chen, ChemBioChem, 2013, 14, 573–576 CrossRef CAS PubMed.
- X. Yang and W. A. van der Donk, Chemistry, 2013, 19, 7662–7677 CrossRef CAS PubMed.
- M. A. Ortega and W. A. van der Donk, Cell Chem. Biol., 2016, 23, 31–44 CrossRef CAS PubMed.
- M. Ma, Y. Xue, W. Liu, H. Zhang, L. Kong, S. Wang and Y. Chen, Chem. Commun., 2014, 50, 10430–10433 RSC.
- L. Jiang, Y. J. Xue, W. Y. Liu, M. Ma, X. R. Wu, S. Z. Wang and Y. J. Chen, Chin. J. Nat. Med., 2015, 13, 854–860 Search PubMed.
- X. R. Wu, P. Y. Huang, Y. J. Xue, W. Y. Liu, M. Ma and Y. J. Chen, RSC Adv., 2016, 6, 72399–72408 RSC.
Footnotes |
† Electronic supplementary information (ESI) available. See DOI: 10.1039/c6ra20302g |
‡ Shuzhen Wang and Xulu Zheng contributed equally to this work. |
|
This journal is © The Royal Society of Chemistry 2016 |