DOI:
10.1039/C6RA19901A
(Paper)
RSC Adv., 2016,
6, 99514-99523
Fabrication of a multifunctional nanocomposite containing INP coated with chitosan coupled with folic acid and loaded with quercetin in diagnosis and its possible treatment of cancer
Received
6th August 2016
, Accepted 7th October 2016
First published on 13th October 2016
Abstract
Quercetin is one of the most prevalent as well as thoroughly studied dietary flavonoids with several biological and anticancer properties. However, its low bioavailability and poor solubility in aqueous medium (∼2%) are major concerns associated with the therapeutic application of quercetin. Therefore, the present work is aimed at designing a nanocomposite that will be nontoxic, cost effective and enhance the increased solubility of the drug quercetin that possess anticancer properties. The multifunctional nanocomposite was developed using iron oxide nanoparticles (INP) extracted and synthesized from magnetite sand and further fabricated using chitosan, folic acid and finally loaded with aqueous poorly soluble quercetin. The developed nanocomposite was characterized for its physicochemical properties. To confirm the anticancer properties, the nanocomposite was treated with two different cell lines and assessed by flow cytometry and by ethidium bromide acridine orange staining to detect the apoptotic and necrotic cells respectively. The nanoparticles were crystalline in nature with average size distribution values of 17.58 d nm and 42.7 d nm for INPs and Q-INP-CF respectively. Moreover, vibrating sample magnetometer (VSM) and Magnetic Resonance Imaging (MRI) results revealed the magnetic properties of Q-INP-CF. Q-INP-CF induced apoptosis in both PC-3 and MCF-7 as observed using flow cytometry and by ethidium bromide acridine orange staining. The prepared Q-INP-CF can be effectively used as a novel and cheaper MRI contrast agent with MRI sensitivity which will be helpful in both diagnosis and eradicating cancer cells.
1. Introduction
Quercetin (Q) is known to have modulatory effects on cell apoptosis, migration and growth via various signaling pathways. Although quercetin possesses great medicinal value, its applications as a therapeutic drug are limited. Problems like low oral bioavailability and poor aqueous solubility make quercetin an unreliable candidate for therapeutic purposes. Additionally, the rapid gastrointestinal digestion of quercetin is also a major barrier for its clinical translation.1 Hence, to overcome these disadvantages quercetin-based nanoformulations have been considered recently. Nanoformulations of quercetin have shown promising results in its uptake by the epithelial system as well as enhanced delivery to the target site. In the present work iron oxide nanoparticles (INP) will be used for the preparation of the nanocomposite. INP will be coated with chitosan natural polycationic biopolymer which is a biodegradable, biocompatible and non toxic polymeric material2,3 that will act as a potential carrier for quercetin. Folic acid (F), an essential water soluble vitamin, is used as a target ligand for the folate receptor which is usually over expressed in prostate and other cancers.4,5
Biomedical uses of magnetic nanoparticles are mostly focused on tumor cells investigation and treatment. Iron oxides, such as magnetite (Fe3O4) are rather stable against oxidation and display significant magnetization, being preferred in various applications and also due to their lack of toxicity.6–8 Synthesis of iron oxides in the nano range for various applications has been an active and challenging area of research during the last two decades. The processes include careful choice of pH, concentration of the reactants, temperature, method of mixing, and rate of oxidation.9 The nano iron oxides have been synthesized by almost all the known wet chemical methods which include precipitation at ambient/elevated temperatures, surfactant mediation, emulsion/micro-emulsion, electro-deposition etc.
Iron is one of the major components of earth's crust accounting about 180 billion tons of iron ore reserves respectively and this excessive occurrence leads to the extensive processing of iron ores into valuable metallic products useful for household applications.10 But still there is no evidence in biomedical application. Hence in the present study, magnetite sand obtained from sand river beds is used as the starting material for the synthesis of INP. The earth abundant magnetite sand comprises of about 58–60% iron which can be procured using physical treatments such as magnetic and gravity methods.11 To be better used for in the present study, INPs are coated with chitosan, conjugated with folic acid, and finally loaded with quercetin. The nanocomposite is characterized and evaluated for its bioactivity.
2. Materials and methods
Annexin V-FITC Apoptosis Detection Kit, N-hydroxysuccinimide (NHS), N,N′-dicyclohexylcarbodiimide (DCC), folic acid and quercetin were purchased from Sigma Aldrich Chemicals Pvt Ltd (USA). fetal bovine serum, penicillin/streptomycin antibiotic and trypsin EDTA were purchased from Gibco. All other chemicals were obtained from Sisco Research Laboratories (SRL), Pvt Ltd, India. All the chemicals used were extra pure and were of molecular biology grade.
Extraction and synthesis of INPs from magnetite sand
River sand was collected and separated using a magnet to obtain magnetite sand. Synthesis of INPs from magnetite sand was done as described by Prabu et al., (2014).12 Briefly, acid leaching was used for the rapid synthesis of INP (magnetite-Fe3O4) with high yield. Magnetite sand weighing 25 g was stirred in 37.5 wt% hydrochloric acid (HCl) at 80 °C for 1 h to obtain iron leachate. The iron leachate was centrifuged at 6000 rpm for 10 min to remove silica and other impurities. The supernatant was collected and treated with 5% H2O2, which serves as an oxidative agent. The solution was filtered using Whatman filter paper and the filtrate containing Fe3+ and Fe2+ ions was stirred vigorously at 70 °C for 45 min. To this, dilute ammonium hydroxide solution (1
:
1, v/v) was added and the pH was increased to 11, which resulted in the formation of a dark black colored precipitate. The precipitate obtained was stirred vigorously at 60 °C for 30 min under nitrogen atmosphere. The resulting INP were washed several times with ethanol/water (1
:
1) and dried at 100 °C.
Extraction of chitosan from crab shells
The exoskeleton of Gecarcoidea natalis (red crab) was collected from the local market near the coastal area of Muttukaadu, Chennai, Tamil Nadu, India. Chitosan was extracted from the exoskeleton of crab waste by the modified method described by Kim (1999),13 and by the Sonat Corporation (1991).14 The shells were washed and dried under the sun light. The viscera and tissues were carefully removed and placed in hot air oven at 60 °C for 24 h. The shells were crushed into small pieces and were packed into the airtight containers. Extraction of chitosan involves a three step process, deproteinization, demineralization and deacetylation. The sample was deproteinized with 200 mL of 1 N NaOH at 80 °C for 24 h with constant stirring in order to decompose the albumen into water soluble. The NaOH was exchanged intermittently and the sample was washed with deionised water every time before adding fresh NaOH. After 24 h the sample was filtered. The sample filtrate was washed and dried. Followed by deproteinization demineralization was done. This was performed in order to remove the minerals mainly calcium carbonate. About 20 g of sample powder was demineralised with 200 mL of 2 N HCl for 24 h with constant stirring and thus filtered. The filtrate was washed with distilled water and filtered till the liquid showed neutral pH. The filtrate was then dried in a vacuum dryer and weighed. Finally the deacetylation process was carried out by adding 100 mL of 50% NaOH and then boiled at 180 °C for 2 h on a hot plate. The samples were then placed under the hood and cooled for 30 min at room temperature. Afterwards the samples were washed continuously with the 50% NaOH and filtered in order to retain the solid matter. The samples were over dried at 120 °C for 24 h. The solid matter formed was chitosan and was creamy white in colour.
Surface modification of iron oxide nanoparticle with chitosan (INP-C)
The iron oxide nanoparticle obtained from magnetite sand was coated with chitosan extracted from crab shells. Chitosan will interact with negatively charged molecules such as the hydroxyl (Fe–OH) groups on the surface of magnetite nanoparticles. The hydrophilic Fe3O4 cores rapidly adsorb well-dissolved chitosan polymers. The addition of sodium tripolyphosphate (TPP) a polyvalent anion cross-links the adsorbed chitosan molecules to each other through the ionic interactions between the positively charged amino groups of chitosan and negatively charged TPP. Initially, chitosan was dissolved in 1% (w/v) acetic acid solution using sonication until the solution becomes transparent. The iron nanoparticles were dispersed in chitosan solution. Later, TPP dissolved in deionized water was also added to the mixture and allowed for constant stirring for 12 h maintaining a neutral pH. Finally the INP coated with chitosan was separated using a magnet and air dried.
Synthesis of folic acid conjugated, quercetin loaded iron oxide nanocomposite (Q-INP-CF)
Folic acid (1 g) was dissolved in 10 mL DMSO, subjected to ultrasound for 10 min, mixed with 12 mg N-hydroxysuccinimide (NHS), 62 mg N,N′-dicyclohexylcarbodiimide (DCC) and the mixture was stirred in the dark for 12 h. Later, 10 mL of chitosan coated iron oxide nanoparticles (containing 15 mg Fe3O4 nanoparticles), was added subjected to ultrasound for 20 min, and stirred overnight in dark cold room. The folic acid possesses two carboxyl groups, termed α- and γ- and the latter exhibits a much higher reactivity in a carbodiimide-mediated coupling to the reactive amino group of chitosan. Folic acid is conjugated to chitosan directly by activating the γ-COOH group of folic acid by coupling agents, N,N′-dicyclohexylcarbodiimide (DCC) and with N-hydroxysuccinimide (NHS).15,16 The mixed solution was separated with an external magnetic field, alternately rinsed with ultra-pure water and ethanol 4–5 times, then put through dialysis and dispersed in ultrapure water, to obtain folate-conjugated Fe3O4 nanoparticles. Further, the INP-CF was added to the 10 mg of quercetin dissolved in ethanol with constant stirring at high speed for 6 h. Finally, the fabricated INP-C coupled with folic acid and loaded with quercetin is separated using a magnet, air dried and lyophilized to obtain Q-INP-CF in the powdered form.
Characterization of Q-INP-CF nanocomposite
UV-vis spectroscopy. Schimadzu 1700 UV-vis spectrophotometer was used to record the absorbance of INP, chitosan, INP-C, folic acid and Q-INP-CF. Each sample was diluted in deionised water (0.1 mg mL−1) and the spectra were recorded at the wavelength of 200–800 nm.
XRD. Crystal structure of Q-INP-CF were detected using Rigaku Miniflex II desktop X-ray Diffractometer (Rigaku, Tokyo, Japan) with Cu Kα (wavelength 1.5406 Å) radiation over range of 10–80° angle, step scan 0.02, scan rate 4° min−1 at 40 kV and 30 mA.
Transmission electron microscopy (TEM). The shape and size of the prepared nanoparticles was determined using TECNAI FE12 TEM instrument, operated at 120 kV using SIS imaging software. Samples were prepared by spin coating of the precursor solution on formvar/carbon coated copper grids (mesh size 200) at 37 °C.
Scanning electron microscopy (SEM). SEM images were recorded on Hitachi S-4800 to visualize the surface morphology of Q-INP-CF. Samples were coated with gold ions using an ion coater (Fisons sputter coater) 0.1 torr pressure, 20 mA current and 70 s coating time.
Infrared spectroscopy. The infrared spectra were recorded in the range 400–4000 cm−1 on a Fourier transform infrared spectrometer (FTIR-Shimadzu-8000). Nanoparticle powder (1 mg) was milled with KBr and the mixture was pressed to form a transparent pellet for FTIR analysis.
Hydrodynamic diameter (Dh) measurements and zeta potential (z). The hydrodynamic diameter and zeta potential of coated and uncoated nanoparticles was assessed using Malvern Zetasizer. Dispersion of INP, INP-C, INP-CF and Q-INP-CF were prepared at a concentration of 0.1 mg mL−1 in deionised water, ultrasonicated for 60 min and then subjected to analysis.
Magnetic measurements
Vibrating sample magnetometer (VSM). The saturation magnetization (Ms) and coercive force (Hc) of Q-INP-CF were measured using a vibrating sample magnetometer (VSM, Dexing, Model: 250) with a sensitivity of 10−3 emu. The magnetic field was changed uniformly with a time rate of 66 Oe s−1.
MRI phantom experiment. The MRI images for Q-INP-CF were captured using clinical 1.5 T MRI scanner (Avanto, Siemens, Germany) to confirm the super paramagnetic behavior of Q-INP-CF. Nanoparticles at two different concentrations (40 and 80 μg mL−1) were suspended in a tube containing 2% agarose (low melting) solidified at room temperature and kept at 4 °C. T2 weighted imaging parameters were set as follows: TR = 800 m s−1; TE = 26 m s−1; flip angle, 20°; FOV = 6 × 6 cm; matrix = 230 × 230; slice thickness = 5 mm and slice 0.5 mm. MRI data were analysed using the measurement of the T2-weighted signal intensity for region of interest.
In vitro studies
Cell culture. Human prostate (PC-3) and breast (MCF-7) cancer cell lines were procured from National Centre for Cell Science (NCCS), Pune, India and were cultured in DMEM culture medium with 10% FBS, 5% CO2 at 37 °C with the addition of penicillin/streptomycin according to manufactures protocol. Cells were passaged at 70 to 80% confluence using trypsin EDTA and were used for further studies.
MTT assay. MTT reduction assay was performed to assess % inhibition rate and to evaluate cytotoxicity in PC-3 and MCF-7 cells. Briefly, cells were seeded (5 × 103 cells per well) in a 96-well culture plate and incubated for 24 h. Cells were treated with INP, quercetin and Q-INP-CF (20, 40, 60, 80 and 100 μM) separately for 24 h. Thereafter, MTT at a concentration of 5 mg mL−1 in PBS (pH 7.4) was added to each well (10% v/v), and the cells were further incubated for 4 h at 37 °C in dark. Later DMSO was added to dissolve the blue crystals and the optical density was measured at 570 nm. The inhibition rate (%) related to control wells containing cell culture medium without treatment was calculated by [A] test/[A] control × 100, where [A] test is the absorbance of the test sample and [A] control is the absorbance of the control sample. Six replicates were prepared for each sample.
Apoptosis detection assay. Annexin V-FITC Apoptosis Detection Kit procured from Sigma USA, was employed to detect apoptotic and necrotic cells both in PC-3 and MCF-7 cell lines. The manual of the kit was strictly followed. Briefly, both PC-3 and MCF-7 cells were plated in the 6-well plates (1 × 105 cells per well) and incubated for 12 h. The nanoparticle with and without the drug were introduced to the cells and incubated for another 24 h. A control of non-induced cancer cells at 1 × 106 cells per mL was considered for a zero time data point. Then the cells were collected, washed twice with cold Dulbecco's phosphate buffered saline and re-suspended in binding buffer (1 × 106 cells per mL). After resuspension with binding buffer about 500 μL of PC-3 and MCF-7 cells was transferred to a tube and the non-induced cells were also transferred to another tube. 5 μL of Annexin V FITC conjugate and 10 μL of propidium iodide solution were added to each cell suspension followed by incubation for 15 min at room temperature in the dark. The stained prostate and breast cancer cells were diluted by the binding buffer and directly analyzed by the fluorescence-activated cell sorter (FACS, FACS Calibur, BD Biosciences, USA). The cells were set as positive depending on the fluorescence intensity of Annexin V-FITC or PI. Cells, which are early in the apoptotic process, will stain with the Annexin V FITC conjugate alone. Live cells will show no staining by either the propidium iodide solution or Annexin V FITC conjugate. Necrotic cells will be stained by both the propidium iodide solution and Annexin V FITC conjugate.
Acridine orange and ethidium bromide (AO/EB) assay. AO/EB assay is one of the methods for the quantification of apoptosis.17 The PC-3 and MCF-7 cells were seeded in a T-25 mm culture dish and the cells were treated with INP, Q and Q-INP-CF nanocomposite was treated for 24 h. Medium was removed, and the cells were washed with PBS. The cells were then stained with equal volume of combined staining of AO (5 mg mL−1 in PBS) and EB (3 mg mL−1 in PBS) for 5 minutes at room temperature and examined by an fluorescence microscope (DMI 4000B; Leica Microsystems) at a 10× magnification.
3. Results and discussion
An effort was made to extract chitosan from crab shells that will be cost effective with excellent biodegradability and less toxic in nature. The extracted chitosan was in a powdered creamy white form and the percentage of yield was found to be 38.11%. The physicochemical and functional properties of the prepared chitosan was also carried out to determine parameters such as ash content, moisture content and degree of deacetylation using FTIR. The results of physicochemical and functional properties of the prepared chitosan are given in the (Table 1). iron oxide nanoparticles (INP) was extracted and synthesized from magnetite sand and the magnetic measurements were assessed by vibrating sample magnetometer (VSM) and Magnetic Resonance Imaging (MRI) (Fig. 1D).
Table 1 Physiochemical properties of chitosan extracted from crab shells
S. No |
Characteristics |
Chitosan |
1 |
% yield |
38.11% |
2 |
Moisture content |
5% |
3 |
Residue on ignition |
3.3% |
4 |
pH |
6.5 |
5 |
Solubility |
Soluble in acetic acid, conc. HCl, H2SO4 |
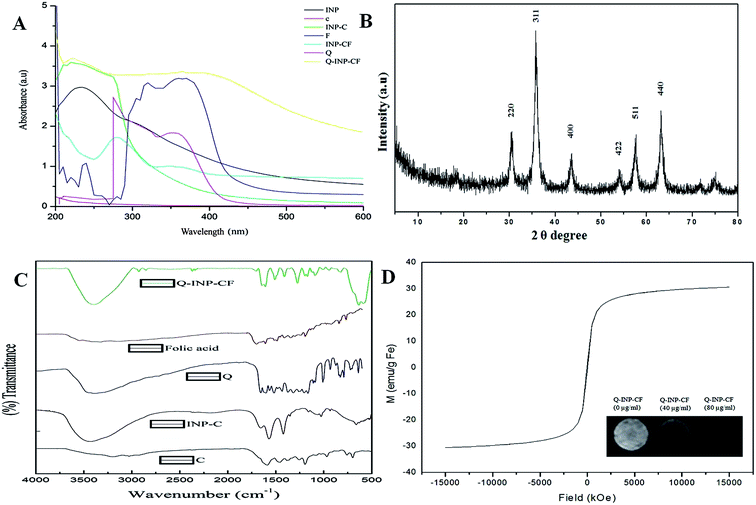 |
| Fig. 1 (A) shows the UV spectra for chitosan, quercetin, folic acid, chitosan coated INP and Q-INP-CF. (B) Represents the powder XRD patterns of Q-INP-CF. (C) Shows the FTIR spectroscopy for chitosan, quercetin, folic acid, chitosan coated INP and Q-INP-CF. Remarkable absorption peaks were obtained by Q-INP-CF. Distinct peak at 582 cm−1 for Fe–O bond. The specific band at 1406 cm−1 for benzene ring of folic acid. Absorption peaks at 839 cm−1 and 1506 cm−1 was observed for the aromatic groups present in quercetin. (D) Gives the magnetization curves of Q-INP-CF measured by VSM at room temperature and T2-weight phantoms of Q-INP-CF at two different concentrations in 1.5% agarose using 1.5 T MRI. Q-INP-CF at 80 μg mL−1 concentration shows high drop in signal and darkening of pixel due to the increased concentration of Fe. | |
UV-vis spectroscopy
UV-vis spectroscopy is more often applied for the study of covalent and non-covalent interactions. Certain functional groups present in organic molecules absorb light at characteristic wavelengths in the UV-vis region, this technique is applied qualitatively to identify the presence of these groups in the samples and the interaction between them.18
In Fig. 1A iron oxide nanoparticle shows a characteristic peak at 232 nm and chitosan at 278 nm where as chitosan modified iron oxide nanoparticle shows peak at 274 nm. The shifting of peaks confirms the coating of chitosan on the surface of iron oxide nanoparticles. Folic acid exhibits a strong absorbance at 238 nm, a shoulder peak at about 303 nm, and a further strong peak at 373 nm. The peaks obtained are very much similar to that obtained by19 The spectrum for folic acid conjugated, chitosan coated iron oxide nanoparticle (INP-CF) shows two characteristic bands one at 279 nm and the other at 358 nm. The peak at 358 nm clearly suggests the bonding of folic acid to chitosan coated INP, there is slight shift in the peak from 373 nm to 358 nm which the characteristic peak for folic acid whereas the peaks obtained at 238 nm and shoulder peak at 303 nm have disappeared in the UV spectrum of INP-CF. Similarly the peak at 279 nm confirms the presence and bonding of chitosan to INP in the UV spectrum of INP-CF.
It is reported that UV-vis spectrum of quercetin has two bands that are attributed to different parts of the conjugated aromatic rings present in it. The first band (band I) at the 300–380 nm corresponds to the cinnamyl and the second band (band II) at 240–280 nm corresponds to the benzoyl.20 In the current study two different bands are observed one at 277 nm and the other at 352 nm which correspond to benzoyl and cinnamyl rings respectively. In the UV spectrum of Q-INP-CF nano composite two distinct peaks are observed one at 222 nm which attributes to the presence of iron oxide nanoparticle and the other at 363 nm which attributes to the cinnamyl ring present in the quercetin and confirms the loading of quercetin to the INP-CF.
XRD
The phase structure of the prepared nanocomposite QT-INP-CF was identified by powder XRD. From a typical XRD pattern (Fig. 1B) six characteristics peaks at 2θ values 30.08, 35.42, 43.05, 53.41, 56.93, 62.5 marked by the indices, (220), (311), (400), (422), (511) and (440) correspond to the characteristic peaks of INP were obtained indicating the cubic spinal structure of Fe3O4. The results obtained coincides with the results of Prabu et al., (2014).12 The peaks of (2θ theta) are the characteristic peaks of organic substance, suggesting that the coating layer indeed was of chitosan, folic acid and quercetin.
TEM
TEM was applied to measure the shape and size of the synthesized nanoparticles. In Fig. 2A the image shows well-separated nanoparticles with a small aggregates. The core sizes of a single Q-INP-CF nanoparticle were found to be very small with an average diameter being in the range of 15–20 nm. The small aggregates of nanoparticles forming nanoclusters is probably due to the magnetism and surface tension of the droplet on the carbon coated TEM grid as it dries during preparation for imaging.
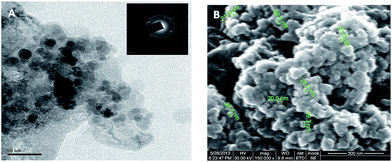 |
| Fig. 2 (A) Shows transmission electron microscopy (TEM) images of Q-INP-CF. Nanoparticles are well-separated with a small aggregates. The inset image is the enlarged image of a single nanoparticle. (B) Gives the scanning electron microscopy (SEM) of Q-INP-CF that appears to be spherical and agglomerated, with a uniform size distribution of around 20–22 nm. | |
Scanning Electronic Microscopy
The Fig. 2B represents the SEM images of Q-INP-CF. Q-INP-CF appears to be spherical and agglomerated, a phenomenon which occurs due to the drying and/or the conductive layer of gold sputtered onto the surface of the nanoparticles. It is reported that particles with small size tend to aggregate due to their high specific surface energy and thus form unclear images under SEM.21 Q-INP-CF is with a uniform size distribution of around 20–22 nm.
FTIR
FTIR spectroscopy is an appropriate technique that provides information on molecular interaction of nanoparticles due to their functional groups and hence monitor changes in the FT-IR spectra of their component parts. The FTIR spectra of chitosan (Fig. 1C) showed a strong absorption band at 3465 cm−1 due to OH and amine N–H symmetrical stretching vibrations. A peak at 3051 cm−1 was due to symmetric –CH2 stretching vibration attributed to pyranose ring.22 The sharp peak at 1365 cm−1 was assigned to CH3 in amide group.23 The broad peak at 1083 cm−1 and 1181 cm−1 indicated the C–O stretching vibration in chitosan and peaks at 1645 cm−1 and 1585 cm−1 were due to –C
O stretching (amide I) and NH stretching (amide II). FT-IR spectrum of INP-C, a peak at 3454 cm−1 is due to the stretching vibrations of water molecules and hydroxyl groups present in the INP and there is shift in the band from 590 cm−1 to 659 cm−1 which attributes to the Fe–O bond vibration of INP. There is a shift in the band for the amide groups of chitosan to 1657 cm−1 and 1597 cm−1 for –C
O stretching (amide I) and NH stretching (amide II) respectively in the chitosan coated INP nanoparticle.
When analyzing the FTIR spectra of free folic acid (FA) a peak at 3542 cm−1 is observed which is due to the hydroxyl stretching bands of glutamic acid moiety and N–H group of the pteridine ring of FA. A band at 1608 cm−1 and 1699 cm−1 represents the bending of N–H vibration and stretching vibration of C
O for free folic acid respectively. The bands at 1488 cm−1 is due to the benzene ring of folic acid. Similarly, for quercetin the major peaks were as follows a strong-broad band at 3388 cm−1 (O–H stretching), a medium-sharp peak at 1655 cm−1 and a strong-sharp 1604 cm−1 (C
O stretching), a strong-sharp peak at 1517 cm−1 (aromatic group), a medium-sharp peak at 1316 cm−1 and 1165 cm−1 (C–O–C stretching), and a weak-sharp peak at 931 cm−1 (aromatic group C–H stretching). The peaks obtained for quercetin is much similar to that obtained by Qi et al., (2015).24
Thus when comparing the above peaks with FTIR spectra of QT-INP-CF a distinct peak at 582 cm−1 was observed which confirms the Fe–O bond vibration of INP. A band at 1507 cm−1 and 1642 cm−1 represents the bending of N–H vibration and stretching vibration of C
O for the presence of folic acid respectively. The band at 1406 cm−1 is specifically due to the presence of benzene ring of folic acid. Thus the peaks obtained for folic acid confirms its conjugation with INP-C.
A slight shift in the peak value from 3388 cm−1 to 3402 cm−1 is observed for OH stretching of quercetin. A shift in the peak at 839 cm−1 and 1506 cm−1 was observed in the QT-INP-CF FTIR spectra which are the characteristic aromatic groups present in the quercetin. Similarly peaks at 1092 cm−1 and 1197 cm−1 indicates the C–O stretching vibration present in chitosan. This clearly suggests that quercetin is loaded on to the INP-CF.
Hydrodynamic diameter (Dh) measurements and zeta potential (z)
The charge on the surface of the coated and uncoated nanoparticles was analyzed using Malvern Zetasizer. The zeta potential values of INP, INP-C and Q-INP-CF are given in Fig. 3. The zeta potential values of INP are found to be increased after coating with chitosan and a slight decrease was observed after conjugating them with folic acid. Average hydrodynamic diameter of Q-INP-CF was found to be 42.7 d nm in size.
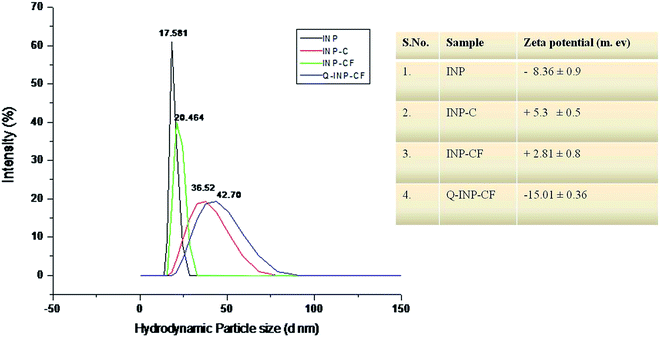 |
| Fig. 3 Hydrodynamic particle size distribution and zeta potential for INP, INP-C, INP-CF and Q-INP-CF. Average hydrodynamic diameter of Q-INP-CF was found to be 42.7 d nm in size. | |
Vibrating sample magnetometry (VSM)
Hysteresis loops of QT-INP-CF were characterized by a vibrating sample magnetometer (VSM) as a function of the magnetic field at room temperature (Fig. 1D). The saturation magnetization of final iron oxide nanocomposite (QT-INP-CF) was about 31.4 emu g−1. According to the VSM results, it was proved that the sample showed super paramagnetic behavior, i.e., after removal of the external magnetic field they did not retain any magnetism.25 Therefore, due to the high magnetization and super paramagnetic properties of QT-INP-CF, it thus becomes suitable for biomedical applications and for hyperthermia process in in vivo to eradicate the cancer cells.
MRI
MRI is a potent technique used for the diagnosis of various diseases like cancer and other impairments. A high performance MR imaging can be produced by a target specific MRI contrast agent with unique superparamagnetism. In the current study, the folic acid in the Q-INP-CF, is used to target the folate receptor and γ-Fe2O3 with unique paramagnetism will help in achieving an enhanced T2*W image. Fig. 1D shows the phantoms of agarose with increasing concentrations of Q-INP-CF. There is no drop in signal and darkening of pixel due to the absence of Fe in the agarose but Q-INP-CF at two different concentrations shows high drop in signal and darkening of pixel due to the increased concentration of Fe. As reported in literature appearance of darker pixels and a substantial MRI drop in signal in general have generated a darkening contrast in T2*W images.26,3 Thus, the prepared Q-INP-CF can be effectively used as a novel and cheaper MRI contrast agent with MRI sensitivity which will be useful in both diagnosis and to remove cancer cells by the process of apoptosis.
MTT assay
The cytotoxicity and % inhibition INP, Q and Q-INP-CF were evaluated in the cancer cells. These results (Fig. 4) clearly suggest that INP does not induce any toxicity to the PC-3 and MCF-7 cells. The percentage inhibitory rate of INP in both the cells is shown to be less than 10%. And quercetin loaded to the INP nanoparticles could inhibit cancer cell proliferation more efficiently than quercetin alone. The IC50 value for Q-INP-CF is observed to be 40 μM and is considered for further studies. Thus, it is expected that Q-INP-CF nanocomposite could efficiently improve the penetration of the drug into the cell membrane without any toxicity, more over the folic acid conjugated to the nanoparticle will effectively facilitate the permeation and uptake of quercetin and increase the accumulation of quercetin within the cancer cell and destroy them completely.
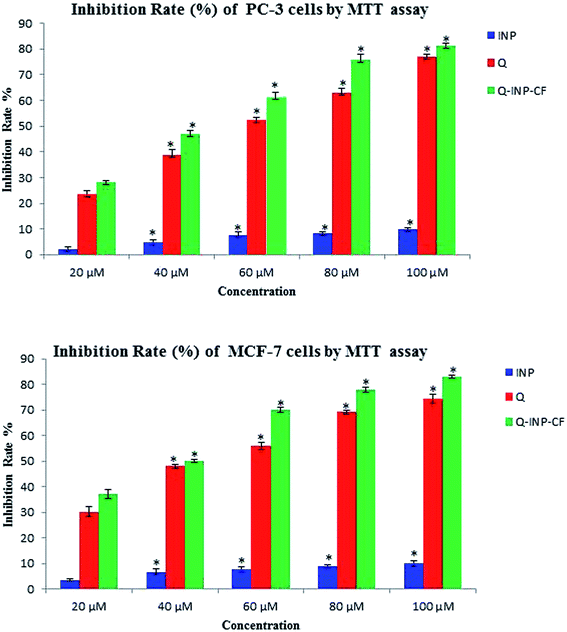 |
| Fig. 4 Represents MTT assay on the inhibition rate of PC-3 and MCF-7 cells after treatment with INP, Q and Q-INP-CF. Q-INP-CF indicates significant inhibition effect on PC-3 and MCF-7 cells in comparison with either INP or quercetin alone treatment. The IC50 value for Q-INP-CF is observed to be 40 μM. Where * represents p < 0.05. Each bar represents the mean ± SEM of six independent observations. | |
Apoptosis assay by Annexin V-FITC and Propidium Iodide (PI) staining
In this staining Annexin V-FITC binds to phosphatidylserine (PS) exposed on the outer leaflet of the plasma membrane without membrane damage, which is the feature of an early stage of apoptosis while remaining propidium iodide (PI) is a DNA-binding dye that can enter the cells when their membranes are ruptured a characteristic of both necrosis and late apoptosis. Presently, the PC-3 and MCF-7 cells stained with PI and Annexin V-FITC were subjected to flow cytometry, and the proportion of live, apoptotic, and necrotic cells was determined. Four different groups were considered and compared control, INP alone, Q alone, Q-INP-CF for 36 h. As shown in Fig. 5, a significant increase in the apoptosis rate (from 1.75% to 21.0% and 1.28% to 22.82% in PC-3 and MCF-7 cells respectively) was observed in Q-INP-CF when compared to INP treated cells. This is probably due to the conjugation of folic acid to the nanocomposite. The folic acid will bind to the folate receptors that are usually over expressed in cancer cells compared to normal epithelial cells and enhances the uptake of the nanocomposite into the cell and thus increases the bioavailability and concentration of the drug quercetin within the cells to trigger the process of apoptosis. Whereas, in the control and INP treated cells there is no significant induction of apoptosis.
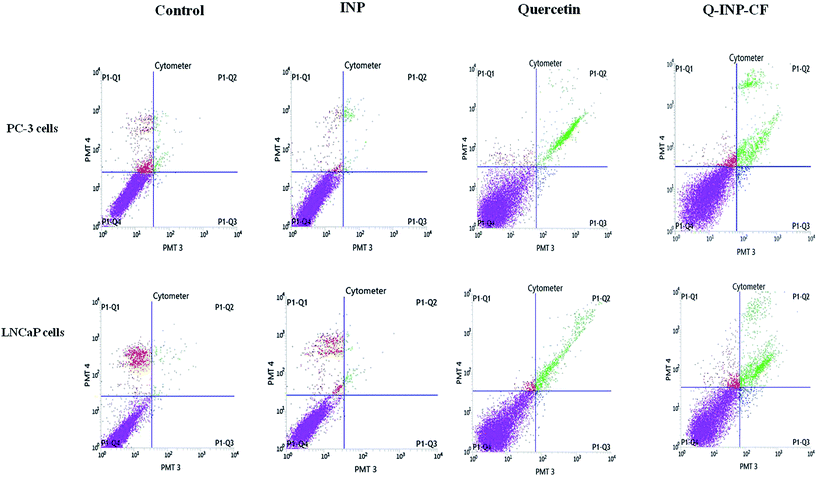 |
| Fig. 5 Effect of Q-INP-CF on apoptosis/necrosis of PC-3 and MCF-7 cells by flow cytometry. After incubation with INP, Q and Q-INP-CF for 36 h, cells were harvested and labeled with a combination of annexin V-FITC and propidium iodide. Significant increase in the apoptosis rate (from 1.75% to 21.0% and 1.28% to 22.82% in PC-3 and MCF-7 cells respectively) was observed upon Q-INP-CF treatment in comparison with INP and quercetin treatment. | |
Apoptosis and necrosis evaluation by fluorescent double staining
To further confirm apoptosis, dual AO/EB staining (Fig. 6A and B) was used in a human prostate (PC-3) and breast cancer (MCF-7) cell lines to examine the morphological changes taking place in the cells. These dyes emit different shades of fluorescence and possess a different ability to penetrate cells. AO penetrates into living cells, emitting green fluorescence after intercalation into DNA. The second dye, EB emits red fluorescence in the cells with an altered cell membrane. Morphological features of apoptosis such as chromatin condensation, nuclear fragmentation, alterations in the size and the shape of cells, as revealed by fluorescence microscopic analysis, were observed predominantly after Q-INP-CF treatment compared to quercetin alone treated cells after 24 h. The control and INP induced cells did not show significant apoptotic or necrotic cells. There are various mechanistic pathway reported from literature by which quercetin induces apoptosis to tumor cells. Several researchers have reported the activation of the mitochondrial pathway, and therefore caspase-9.27,28 Bax translation leads to the leakage of cytochrome c from mitochondria29 cytochrome c binds to Apaf-1, which then permits recruitment of procaspase-9; caspase-9 cleaves and activates executioner caspases including procaspase-3. The activated caspases thus induces apoptosis to cancer cells.
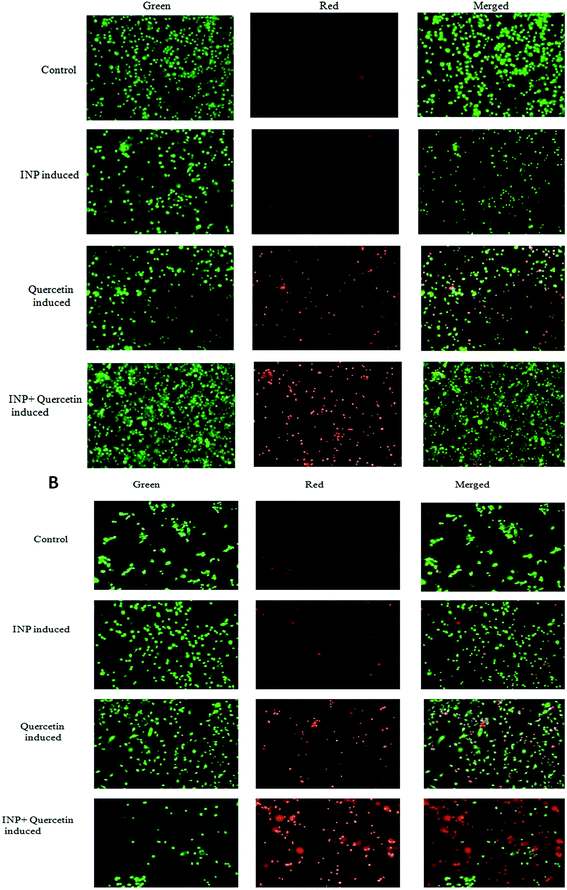 |
| Fig. 6 (A) & (B) Apoptosis detection by acridine orange ethidium bromide (AO/EtBr) staining in PC-3 (A) and MCF-7 (B) cells. Induction of apoptosis was observed predominantly after Q-INP-CF treatment compared to quercetin alone treated cells after 24 h. The control and INP induced cells did not show significant apoptotic or necrotic cells. | |
To summarize a cost effective and eco-friendly Q-INP-CF is synthesized utilizing the indigenous materials such as iron oxide nanoparticle from magnetite sand and chitosan from crab shells. Quercetin loaded iron oxide nanoparticle was prepared in order to increase the bioavailability at the target site of cancer tissue, this was assessed by flow cytometry and AO/EtBr staining. There is a significant increase in the induction of apoptosis in prostate and breast cancer cells when treated with Q-INP-CF. In MRI application Q-INP-CF at two different concentrations shows high drop in signal and darkening of pixel due to the increased concentration of Fe. Thus, the prepared Q-INP-CF can be effectively used as a novel and cheaper MRI contrast agent with MRI sensitivity which will be helpful in both diagnosis and killing of cancer cells.
Acknowledgements
The financial assistance provided by DST as Women scientist Fellow to Dr Gunadharini D. N. is gratefully acknowledged (order letter no. SR/WOS-A/LS-1156/2014 (G) dt 28.10.2014). Our thanks to Dr J. Arunakaran, Head of the Department, Endocrinology Department, IBMS, University of Madras for providing facility to perform in vitro studies.
References
- J. E. Kipp, Int. J. Pharm., 2004, 284, 109–122 CrossRef CAS PubMed
. - V. R. Sinha, A. K. Singla, S. Wadhawan, R. Kaushik, R. Kumria, K. Bansal and S. Dhawan, Int. J. Pharm., 2004, 274, 1–33 CrossRef CAS PubMed
. - M. Chamundeeswari, S. S. Liji, J. P. Justin, M. Ganesh Kumar, M. Pandima Devi and T. P. Sastry, Biotechnol. Appl. Biochem., 2010, 55, 29–35 CrossRef CAS PubMed
. - F. Sonvico, S. Mornet, S. Vasseur, C. Dubernet, D. Jaillard, J. Degrouard, J. Hoebeke, E. Duguet, P. Colombo and P. Couvreur, Bioconjugate Chem., 2005, 16, 1181–1188 CrossRef CAS PubMed
. - J. Pan and S. S. Feng, Biomaterials, 2009, 30, 1176–1183 CrossRef CAS PubMed
. - F. A. Tourinho, J. Depeyrot, G. J. da Silva and M. C. L. Lara, Braz. J. Phys., 1998, 28, 345–359 CrossRef
. - S. Gustafsson, A. Fornara, K. Petersson, C. Johansson, M. Muhammed and E. Olsson, Cryst. Growth Des., 2010, 10, 2278–2284 CAS
. - E. Cheraghipour, S. Javadpour and A. R. J. Mehdizadeh, J. Biomed. Sci. Eng., 2012, 5, 715–719 CrossRef
. - C. Domingo, R. Rodriguez-Clemente and M. J. Blesa, J. Colloid Interface Sci., 1994, 165, 244–252 CrossRef CAS
. - S. Wu, A. Sun, F. Zhai, J. Wang, W. Xu, Q. Zhang and A. A. Volinsky, Mater. Lett., 2011, 65, 1882–1884 CrossRef CAS
. - W. Widanarto, M. R. Sahar, S. K. Ghoshal, R. Arifin, M. S. Rohani and K. Hamzah, J. Magn. Magn. Mater., 2013, 326, 123–128 CrossRef CAS
. - P. Prabu, S. V. Weslen, B. Sreedhar, B. Santhosh Kumar and T. P. Sastry, Mater. Chem. Phys., 2014, 148, 1212–1220 CrossRef
. - D. Kim, http://www.members.tripod.com/-Dalwoo, 1999.
- C. Sonat, http://www.geocities.com/chitosanus, 1991.
- P. Li, Y. Wang, F. Zeng, L. Chen, Z. Peng and L. X. Kong, Carbohydr. Res., 2011, 346, 801–806 CrossRef CAS PubMed
. - Y. Zu, Q. Zhao, X. Zhao, S. Zu and L. Meng, Int. J. Nanomed., 2011, 6, 3429–3441 CrossRef CAS PubMed
. - D. Baskic, S. Popovic, P. Ristic and N. N. Arsenijevic, Cell Biol. Int., 2006, 30, 924–932 CrossRef CAS PubMed
. - J. Kumirska, M. Czerwicka, Z. Kaczyński, A. Bychowska, K. Brzozowski, J. Thöming and P. Stepnowski, Mar. Drugs, 2010, 8, 1567–1636 CrossRef CAS PubMed
. - G. Ciofani, V. Raffa, A. Menciassi and A. Cuschieri, Nanoscale Res. Lett., 2009, 4, 113–121 CrossRef CAS PubMed
. - D. Yu, J. Chem. Pharm. Res., 2014, 6, 236–240 Search PubMed
. - Y. Zhang, N. Kohler and M. Zhang, Biomaterials, 2002, 23, 1553–1561 CrossRef CAS PubMed
. - A. Pawlak and M. Mucha, Thermochim. Acta, 2003, 396, 153–166 CrossRef CAS
. - H. Zheng, Y. M. Du, J. H. Yu, R. H. Huang and L. N. Zhang, J. Appl. Polym. Sci., 2001, 80, 2558–2565 CrossRef CAS
. - Y. Qi, M. Jiang, Y. Cui, L. Zhao and X. Zhou, Nanoscale Res. Lett., 2015, 10, 1–9 CrossRef CAS PubMed
. - D. Dorniani, M. Z. B. Hussein, A. U. Kura, S. Fakurazi, A. H. Shaari and Z. Ahmad, Int. J. Mol. Sci., 2013, 14, 23639–23653 CrossRef PubMed
. - C. G. Hadjipanayis, M. J. Bonder, S. Balakrishnan, X. Wang, H. Mao and C. George, Small, 2008, 4, 1925–1929 CrossRef CAS PubMed
. - I. Wang, S. Lin-Shiau and J. Lin, Eur. J. Cancer, 1999, 35, 1517–1525 CrossRef CAS PubMed
. - M. van Erk, P. Roepman, T. van der Lende, R. Stierum, J. Aarts, P. van Bladeren and B. van Ommen, Eur. J. Nutr., 2005, 44, 143–156 CrossRef CAS PubMed
. - P. Costantini, E. Jacotot, D. Decaudin and G. Kroemer, J. Natl. Cancer Inst., 2000, 92, 1042–1053 CrossRef CAS PubMed
.
|
This journal is © The Royal Society of Chemistry 2016 |