DOI:
10.1039/C6RA19565B
(Paper)
RSC Adv., 2016,
6, 112370-112380
Anaerobic vs. aerobic preparation of silicon nanoparticles by stirred media milling. The effects of dioxygen, milling solvent, and milling time on particle size, surface area, crystallinity, surface/near-surface composition, and reactivity†
Received
2nd August 2016
, Accepted 14th November 2016
First published on 17th November 2016
Abstract
A stirred media mill was hermetically sealed in a high-purity dinitrogen atmosphere glovebox and used to prepare silicon nanoparticles (SiNPs) by milling a slurry of 45–90 μm pieces of metallurgical-grade silicon (MGS) suspended in anhydrous heptane (HEP), heptane containing a small amount of mesitylene (HEP/MES), or mesitylene containing 22 mM pyrene (MES/PYR) under anaerobic and anhydrous conditions (≤5 ppm dioxygen (O2) and water (H2O) vapor). Excess solvent and other volatiles were removed using a rotary evaporator also housed in the glovebox. The SiNPs were further dried at 22 ± 1 °C under vacuum (≤1 × 10−5 Torr) for at least 15 h. The dark-brown or black pyrophoric nanoparticle powders were stored in a high-purity argon atmosphere glovebox. Samples were removed from the glovebox and analyzed by BET surface area (BET-SA) determination, tensimetric titrations with various pressures of O2, PXRD, SEM, TEM, ATR-FTIR, and/or XPS. The effects of (i) anaerobic vs. aerobic milling, (ii) milling fluid, and (iii) milling time on BET-SA, degree of crystallinity, reactivity with O2, and FTIR- or XPS-determined surface/near-surface composition of the SiNPs are discussed. The BET-SA of SiNPs milled anaerobically for 5 h in MES/PYR (258 m2 g−1) was ca. twice as large as the BET-SA for SiNPs milled aerobically in the same milling fluid. In contrast, the BET-SA of SiNPs milled anaerobically in HEP (40 m2 g−1) was four times smaller than for SiNPs milled aerobically in HEP. The PXRD-determined degree of crystallinity steadily decreased and the BET-SA steadily increased over time (1, 2, or 5 h) for SiNPs milled anaerobically in MES/PYR. The degree of crystallinity of SiNPs milled anaerobically in HEP also steadily decreased over time, but the BET-SAs for 1, 2, 3, 4, and 5 h milled samples were essentially the same. MES/PYR milled SiNPs reacted more slowly with O2 and irreversibly absorbed less O2 than HEP milled SiNPs.
Introduction
The preparation of nanoparticles (NPs) by top-down methods including stirred media milling (attritor milling; e.g., Al, Si, Fe, Cu, BCS Class II drugs),1–4 wet grinding (e.g., Si),5 ball milling (including reactive high-energy ball milling; e.g., Sm(Co0.8Fe0.2)17, SmCo5, Bi2(Te/Se)3, Eu-doped Y2O3, graphite),6–10 sonication (e.g., Bi0.9Gd0.1(Ti/Fe)O3, Si),11–13 and laser ablation (e.g., Si)14 is of current interest. In general, the surface chemistry, morphology, size distribution, physicochemical properties, and crystallinity of NPs are more difficult to control using top-down methods (e.g., Al2O3, SnO2, coconut shell)15–24 than with typical bottom-up methods (e.g., Si, Cu2ZnSn(S/Se)4, Bi0.5Sb1.5Te3, CdTe, Fe-doped MoC2, glipiside).25–31 However, depending on the application the advantages of cost, reproducibility (i.e., fewer chemical or process variables), and the simplicity of scale-up of top-down methods can outweigh the disadvantages. In fact, in some cases relatively narrow particle size distributions have been observed (e.g., Fe3O4)32 and NPs with chemically-tailored surfaces have been prepared using top-down methods (e.g., Si),33,34 and in other cases crystallinity was restored or partially restored after top-down milling (e.g., ZSM-5 zeolite).35
The goal of this project was to determine the differences and similarities of several properties of NPs of a typical semiconductor material prepared by stirred media milling under both aerobic and strictly anaerobic/anhydrous conditions. Anaerobic ball milling has been reported and is relatively straightforward.9,10,33,34,36 However, to our knowledge there is only one report of anaerobic stirred-media milling.37 A typical attritor mill such as the Netzsch MiniCer used in this work operates by continuously cycling a slurry through a water cooled and pressurized milling chamber by means of a closed loop that includes a peristaltic pump, an inline sonicator, and an efficient stirrer. Housing all of this equipment, plus their power supplies, switches, sensors, and pressurized mill-fluid and cooling-water lines in an inert-atmosphere glovebox so that anaerobic/anhydrous milling could be efficiently and reproducibly carried out by a single operator was a significant challenge (see ESI†).
We chose metallurgical grade silicon (MGS) as an inexpensive test material with which to prepare silicon nanoparticles (SiNPs), in part because of the current interest in, and the wealth of information on the properties of, SiNPs prepared by top-down as well as bottom-up methods.38 Heptane and mesitylene were chosen as test organic solvents. Their densities, viscosities, and surface tensions are substantially different: 0.680 g cm−3, 0.39 mPa s,39 and 19.6 nN m−1,40 respectively, for heptane, and 0.861 g cm−3, 0.66 mPa s,41 and 28.1 nN m−1,42 respectively, for mesitylene. In addition, recent literature has shown that relatively inert aliphatic hydrocarbons such as heptane would not be expected to undergo strong, irreversible chemisorption to surfaces,33,34 in contrast to the well-established high reactivity of aromatic compounds with silicon surfaces.43 Finally, pyrene was chosen as a potentially relevant surface modifier because pyrene derivatives have been attached to the surfaces of Si nanowires (albeit through Si–O–C linkages and not through Si–C(sp2) bonds).44
Experimental
I. Chemicals
Metallurgical grade silicon (MGS) was obtained from Kratos LLC (98.9 ± 0.2% Si, 0.35 ± 0.30% Fe, 0.2 ± 0.15% Al, 0.12 ± 0.06% Ca). Pyrene (Alfa Aesar, 98%), ethanol (Pharmco-AAPER, 200 proof, ACS/USP grade), 2-propanol (Fisher, 99.999%), Apiezon Type N grease, Dow-Corning High-Vacuum Silicone Grease, dinitrogen (N2; Airgas UHP, 99.999%), and O2 gas (AirGas, 99.5%) were used as received. Anhydrous O2-free heptane (Fisher, HPLC grade) and mesitylene (Alfa Aesar, 98+%) were prepared by refluxing over sodium metal for 12 h followed by distillation under an atmosphere of purified N2. Boil-off liquid nitrogen (AirGas, 99.999%) and liquid argon (AirGas, 99.999%), both certified to be <1 ppm O2, <1 ppm H2O vapor, and <0.5 ppm total hydrocarbon, were used to purge separate gloveboxes.
II. Preparation of silicon nanoparticles (SiNPs) by stirred media milling
Slurries of 45–90 μm pieces of MGS (20 g) that had been hand ground in a mortar and pestle and sieved and 460 mL of milling fluid (either heptane (HEP), heptane containing a small amount of mesitylene (HEP/MES), or mesitylene containing 22 mM pyrene (MES/PYR)) were circulated through the attritor mill with a peristaltic pump. The attritor mill was a continuous-flow Netzsch MiniCer Laboratory stirred media mill with a 160 mL yttrium-stabilized zirconium oxide-lined milling chamber, a cylindrical yttrium-stabilized zirconium oxide slotted mixer blade operating at 4104 rpm, a 150 μm stainless-steel separator screen, and double mechanical seals separating the milling chamber from the motor bearings by means of 80 psi seal fluid, which was either heptane or mesitylene (see schematic drawing in Fig. S-1†). The milling chamber was filled with yttrium-stabilized zirconium oxide beads (Netzsch, 0.3–0.4 mm) and was connected to an inline QSonica sonicator (700 W, 20 kHz) equipped with an 85 mL low-flow cell and a 1.27 cm diameter probe. The sonicator was programmed to operate for 3 minutes at 30% of peak amplitude every 10 minutes during milling. (Sonication has been shown to improve de-agglomeration and dispersion of NPs during wet milling, shortening the time needed to reach “limiting fineness”.45) The milling loop assembly also included a 200 mL stainless steel tank, in which the slurry was vigorously mixed using an overhead stirrer before it re-entered the milling chamber. The four components of the milling loop assembly (the mill, sonicator cell, peristaltic pump, and mixing tank) were connected with Viton tubing. The slurry was added to the loop assembly via the mixing tank after removing its lid and was circulated through the milling chamber at 20–30 mL min−1 for 1–6 h (the flow rate varied as the viscosity of the slurries increased during the milling process). The slurry was removed from the loop through a valve at the bottom of the mixing tank. The temperature of the slurry during milling was typically 25–30 °C and was not allowed to exceed 32 °C (the temperature was controlled by adjusting the rate at which cooling water flowed through the mill chamber housing).
Initially, when the milling fluid was changed from MES/PYR to HEP, the seal-fluid tank still contained MES, and a small amount of MES leaked into the HEP milling fluid during the operation of the mill. The exact amount is not known, but is estimated to be ≤5% v/v MES in HEP for 5 h of milling.46 Only two samples were prepared in this way. All subsequent HEP milled samples were prepared with HEP in the seal-fluid tank.
Rotary evaporation at ca. 1 × 10−3 Torr and ≤40 °C was used to remove HEP or MES from the milled slurries (control experiments demonstrated that PYR is not sufficiently volatile to be removed from the MES/PYR milled SiNPs under these conditions). The resulting dark-brown-to-black free-flowing SiNP powders were further dried in a sealed glass flask under vacuum (≤1 × 10−5 Torr) at 22 ± 1 °C for at least 15 h to remove any remaining traces of HEP, MES, or other volatiles that may have been present as free or weakly physisorbed hydrocarbon molecules. Vacuum drying was continued until no further condensation of any volatile substance was observed in an empty −196 °C cold trap connected to the sealed glass flask containing the SiNP powder.
For anaerobic/anhydrous milling all operations except (i) grinding MGS into 45–90 μm pieces and (ii) vaccum drying the SiNP powders at ≤1 × 10−5 Torr were carried out in a purified N2 atmosphere glovebox. The strategies and procedures used to hermetically seal the Netzsch mill (with its associated cooling-water lines, power supply, various electronic interlock safeguards, and 80 psi seal-fluid tank), sonicator, peristaltic pump, stainless steel mixing tank, overhead stirrer, and Buchi RII rotary evaporator in the glovebox are described in the Ph.D. dissertation of one of us (see also Fig. S-2†).47 The glovebox was continuously purged with the boil-off vapor from liquid N2 certified to contain less than 1 ppm O2 and less than 1 ppm H2O vapor. Anhydrous, O2-free heptane and mesitylene were used. To avoid cross-contamination of HEP-milled SiNPs with MES vapor or MES/PYR-milled SiNPs with HEP vapors, (i) the milling glovebox was purged for 3 days whenever a different milling fluid was to be used and (ii) a separate purified-argon atmosphere glovebox was used to store the SiNP samples after vacuum drying.
III. Surface area determinations
Specific surface areas were determined by N2 gas PVT measurements using a glass vacuum line and BET analysis (−196 °C sample temperature; N2 monolayer surface-area equivalent = 16.2 Å2 per molecule).48,49 Anaerobically-milled samples (typically ca. 1 g) were weighed in the argon atmosphere glovebox into narrow-neck glass flasks and evacuated at 1 × 10−5 Torr for at least 16 h. The narrow necks minimized the temperature-gradient volume of N2 gas (i.e., the gradient from −196 °C (sample temperature)) to 22 ± 1 °C (i.e., the gas temperature at an MKS Instruments Baratron capacitance manometer (0–1000 Torr range, ±0.1 Torr sensitivity). Measured amounts of N2 were introduced into the apparatus and the pressure of gaseous N2, and hence the amount of adsorbed N2, was determined by PVT measurements after equilibrium was reached (usually ≤15 min). Typical graphical analyses of the data are shown in Fig. S-3.†
IV. Reactions with O2
Anaerobic samples of SiNPs that had been stored in the argon atmosphere glovebox were transferred to glass flasks equipped with Teflon-in-glass high-vacuum valves, were evacuated at ≤1 × 10−5 Torr for 12 h, and were exposed to several small measured doses of O2 gas at 22 ± 1 °C. The change in the pressure of O2 over time was recorded, and the amount of O2 irreversibly absorbed by the sample was determined by PVT measurements (the pressure was measured using the electronic manometer described above).
V. ATR-FTIR spectroscopy
A Thermo Scientific-Nicolet iS50-FTIR with an iATR attenuated total reflectance attachment (ZnSe single-bounce ATR crystal) was used to record FTIR spectra (4 cm−1 resolution, 256 scans). The powdered samples were pressed against the ATR crystal. The anaerobically-milled SiNP samples were exposed to air very slowly in screw-capped scintillation vials (weeks) before recording their FTIR spectra.
VI. Powder X-ray diffraction (PXRD)
Diffraction data were collected on a Scintag X-2 powder X-ray diffractometer, with Cu Kα radiation (λ = 0.154 nm) at 40 mA and 45 kV, a tube divergent slit width of 2 mm, and a tube scatter slit width of 4 mm. The instrument was equipped with a Peltier detector having a scatter slit width of 0.5 mm and a reference slit width of 0.2 mm. The experimental setup was standard Bragg–Brentano geometry with a stationary sample stage. Each sample was scanned from 5° to 90° or from 10° to 80° in 0.02° step increments with 1 s collection time per step. Aerobically-milled SiNP samples were sprinkled onto a thin film of high-vacuum silicone grease applied to a glass microscope slide or were mixed with Apiezon Type N hydrocarbon grease in a mortar and pestle and spread as a paste on a glass slide. Anaerobically-milled samples were prepared by mixing the SiNPs (0.106 ± 0.008 g) and Apiezon Type N grease (0.260 ± 0.007 g) in a mortar and pestle in the argon atmosphere glovebox. The dark brown paste was spread on a glass slide. Diffraction data were recorded as soon as possible after the anaerobically-milled samples were removed from the glovebox. A PXRD pattern for a Si standard sample is shown in Fig. S-4.†
VII. Transmission and scanning electron microscopy
A JEOL JEM-2100F field emission transmission electron microscope was used to record TEM images. Selected SiNP samples were dispersed in ethanol or 2-propanol with a bath sonicator for 10 min. After allowing large aggregates to settle for ca. 1 min, the dispersion was added dropwise to TEM grids (Ted Pella #01840, pure carbon on 200 mesh copper) and allowed to air dry for 10 min. The grids were attached to a double tilt holder and evacuated to 1 × 10−3 Torr to remove the solvent. The samples were imaged at 200 keV and were collected in darkfield view at two angles 90° apart to enhance the contrast between amorphous and crystalline regions and to observe crystalline regions with different orientations. The images were processed using Gatan Microscopy software (v. 2.31.734.0).
A JEOL JSM-6500F field emission scanning electron microscope was used to record SEM images. Selected SiNP samples were prepared as described above.
VIII. X-ray photoelectron spectroscopy
X-ray photoelectron spectroscopy (XPS) experiments were performed on a PHI-5800 system using a monochromatic Al Kα X-ray source. A takeoff angle of 45° was used for all analyses and an electron neutralizer set to 5 μA for anaerobic samples and 35 μA for aerobic samples was used to partially offset sample charging. High resolution spectra were collected using 0.1 eV per step, 100 ms per step, 23.50 eV pass energy, and a spot size of 0.8 × 2 mm. CasaXPS Version 2.3.16 was used to analyze all data. Silicon and carbon spectra were fit using literature values (see Table S-1†)50–61 with a Tougaard background and oxygen spectra were fit with a Shirley background, using an average width of 5 and 70% Gaussian and 30% Lorentzian peak shapes. After fitting the C 1s spectrum, all peaks were shifted by setting the C–C/C–H peak to 285.0 eV to charge-correct all binding energies. The metallic silicon environment (i.e., % Si(Si)) was fit with both 2p1/2 and 2p3/2 peaks. The other Si environments (SiC, SiOx (x ≤ 2)) were fit with single peaks. No trace of Zr, Y, or other contaminants from the milling equipment with the exception of a trace of fluorine from the fluoropolymer tubing, were observed in the XPS spectra.
Results
Table 1 lists the milling parameters, the BET-determined surface areas (BET-SAs), and the surface/near-surface XPS-determined atom% compositions of the SiNP samples studied in this work. The SiNPs milled anaerobically or aerobically in mesitylene containing 22 mM pyrene (MES/PYR) for y h are designated MP-y or MP(air)-y, respectively (y = 1, 2, 5, or 6). The SiNPs milled anaerobically or aerobically in heptane (HEP) for y h are designated H-y or H(air)-y, respectively (y = 1–5). The SiNPs milled anaerobically or aerobically in heptane containing a small amount of mesitylene (HEP/MES) for 5.5 h are designated HM-5 or HM(air)-5, respectively. Each of the five pairs of SiNPs abbreviated MP-1/MP-2, MP-5/MP-6, MP(air)-5/MP(air)-6, H-1/H-2, and H-3/H-4 were prepared in a single milling operation; 50% of the slurry was harvested after the shorter of the two times and the remainder harvested after the longer of the two times. The three batches of SiNPs abbreviated H(air)-5, HM-5, and HM(air)-5 were harvested at a single time (5, 5.5, and 5.5 h, respectively) from separate milling operations.
Table 1 Silicon nanoparticle (SiNP) preparation, BET surface area, and XPS-determined surface compositiona
SiNP sample |
Anaerobic or aerobic milling |
Milling mediumb |
Milling time, h |
BET surface area, m2 g−1 |
Surface and near-surface composition (by weighted XPS peak integration) |
% Si |
% C |
% O |
% F |
% SiC |
% SiOxc |
% SiO2 |
% Si(Si) |
% Si(total)d |
A Netzsch Minicer with 0.3–0.4 mm yttrium-stabilized ZrO2 beads was used to mill 45–90 μm pieces of metallurgical grade silicon. MES/PYR = 22 mM pyrene dissolved in mesitylene. HEP = heptane. HEP/MES = heptane with ≤5% v/v mesitylene. x < 2. The % Si(total) is the sum of % SiC, % SiOx, % SiO2, and % SiSi. |
20–45 μm |
Hand ground |
— |
— |
0.7 |
— |
— |
— |
— |
— |
— |
— |
— |
45–90 μm |
Hand ground |
— |
— |
0.2 |
0 |
9 |
35 |
56 |
45 |
18 |
37 |
0 |
MP-1 |
Anaerobic |
MES/PYR |
1 |
75 |
— |
— |
— |
— |
— |
— |
— |
— |
MP-2 |
Anaerobic |
MES/PYR |
2 |
162 |
— |
— |
— |
— |
— |
— |
— |
— |
MP-5 |
Anaerobic |
MES/PYR |
5 |
258 |
10 |
3 |
0 |
18 |
31 |
62 |
7 |
0 |
MP-6 |
Anaerobic |
MES/PYR |
6 |
206 |
— |
— |
— |
— |
— |
— |
— |
— |
MP(air)-5 |
Aerobic |
MES/PYR |
5 |
130 |
8 |
5 |
3 |
8 |
24 |
56 |
20 |
0 |
MP(air)-6 |
Aerobic |
MES/PYR |
6 |
140 |
— |
— |
— |
— |
— |
— |
— |
— |
H-1 |
Anaerobic |
HEP |
1 |
44 |
8 |
5 |
0 |
54 |
67 |
22 |
11 |
0 |
H-2 |
Anaerobic |
HEP |
2 |
45 |
— |
— |
— |
— |
— |
— |
— |
— |
H-3 |
Anaerobic |
HEP |
3 |
33 |
11 |
4 |
0 |
47 |
62 |
23 |
11 |
3 |
H-4 |
Anaerobic |
HEP |
4 |
36 |
— |
— |
— |
— |
— |
— |
— |
— |
H-5 |
Anaerobic |
HEP |
5 |
40 |
11 |
4 |
0 |
44 |
59 |
26 |
12 |
4 |
H(air)-5 |
Aerobic |
HEP |
5 |
169 |
7 |
7 |
12 |
10 |
36 |
32 |
33 |
0 |
HM(air)-5 |
Aerobic |
HEP/MES |
5.5 |
243 |
4 |
9 |
5 |
15 |
33 |
35 |
32 |
0 |
HM-5 |
Anaerobic |
HEP/MES |
5.5 |
295 |
14 |
3 |
0 |
21 |
38 |
55 |
6 |
1 |
Representative BET-SA plots are shown in Fig. S-3.† Duplicate analyses on selected samples demonstrated that the reproducibility of the BET-SA determinations is ±5% or better. Plots of BET-SA vs. milling time for the three types of anaerobically-milled SiNPs are shown in Fig. 1.
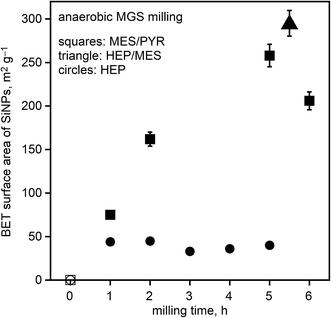 |
| Fig. 1 BET surface areas vs. milling time for SiNPs milled in heptane (HEP), heptane containing a few percent mesitylene (HEP/MES), and mesitylene containing 22 mM pyrene (MES/PYR). The error bar shown are ±5% and in some cases are smaller than the width or diameter of the data points. | |
Representative XPS spectra for MP-5, MP(air)-5, H-5, and H(air)-5 and their deconvolutions with 2, 3, or 4 Gaussian curves based on the literature binding energies listed in Table S-1† are shown in Fig. 2. A comparison of XPS spectra for H-1, H-3, and H-5 is shown in Fig. S-5.†
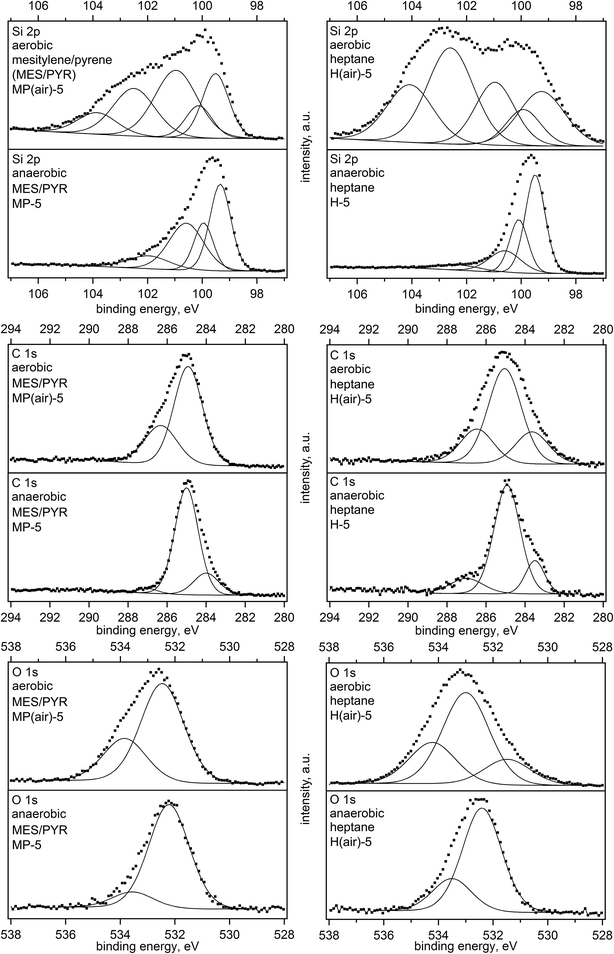 |
| Fig. 2 X-ray photoelectron spectra of selected SiNPs milled aerobically and anaerobically in this work. | |
SEM images of HM(air)-5 at two magnifications are shown in Fig. 3. TEM images of H-1 and H-5 are shown in Fig. 4. TEM images of HM(air)-5 showing both crystalline and amorphous regions and of H-1 at two orthogonal beam orientations are shown in Fig. S-6 and S-7,† respectively.
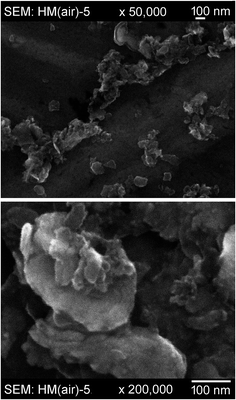 |
| Fig. 3 Scanning electron micrographs at two magnifications of SiNPs milled aerobically in heptane containing a few percent mesitylene. | |
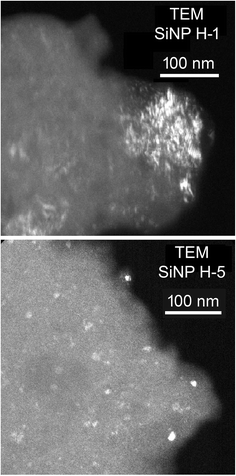 |
| Fig. 4 TEM images of a single SiNP milled anaerobically in heptane for 1 h (top) and for 5 h (bottom). | |
PXRD patterns for hand-ground 20–45 μm pieces of MGS, for anaerobically-milled SiNPs MP-y (y = 1, 2, 5, 6) embedded in high-vacuum silicone and H-y (y = 1–5) embedded in Apiezon Type N grease are shown in Fig. 5. To make the comparisons easier to understand, Fig. 5 also lists the milling time and BET-SA for each sample. Table 2 compares BET-SA results and PXRD estimated average crystallite sizes for MP-y and H-y.
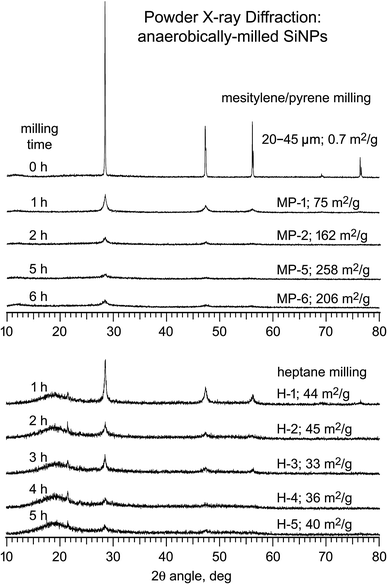 |
| Fig. 5 PXRD patterns for 20–45 μm hand-ground MGS particles (top, milling time = 0) and for SiNPs milled in mesitylene/pyrene (top) and heptane (bottom) at various milling times. The BET-determined surface area for each sample is also shown. See Table 1 for sample abbreviations. | |
Table 2 Silicon nanoparticle (SiNP) BET surface area and PXRD-estimated crystallite size
SiNP samplea |
BET surface area, m2 g−1 |
Estimated crystallite size,b nm |
See Table 1 for a key to sample abbreviations. Estimated by PXRD peak-width analysis using the Scherrer equation (see ESI for details). The signal/noise ratio for this PXRD pattern was too low for a meaningful estimation of the peak full-width at half height (see Fig. 5). |
MP-1 |
75 |
14 |
MP-2 |
162 |
11 |
MP-5 |
258 |
9 |
MP-6 |
206 |
8 |
H-1 |
44 |
43 |
H-2 |
45 |
22 |
H-3 |
33 |
13 |
H-4 |
36 |
—c |
H-5 |
40 |
—c |
ATR-FTIR spectra of several samples are shown in Fig. 6. Significantly, SiNP samples that had been milled in HEP/MES or MES/PYR, either anaerobically or aerobically, and evacuated at 1 × 10−5 Torr for ≥15 h at room temperature, exhibited aromatic ν(CH) bands at ca. 2730 and 3015 cm−1 indicating the presence of strongly physisorbed or chemisorbed mesitylene. Samples milled in MES/PYR also exhibited an aromatic ν(CH) band at ca. 3045 cm−1 indicative of PYR. For comparison, spectra of liquid HEP, liquid MES, and solid PYR are shown in Fig. S-8.†
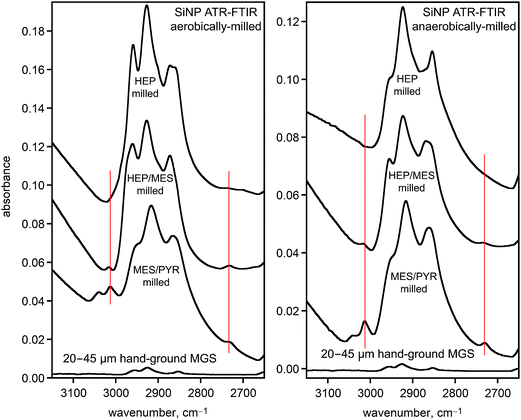 |
| Fig. 6 ATR-FTIR spectra of SiNPs milled either aerobically (left) or anaerobically (right) in heptane (HEP), heptane containing a small percentage of mesitylene (HEP/MES), and mesitylene containing 22 mM pyrene (MES/PYR). | |
Tensimetric plots of P(O2) vs. time are shown in Fig. S-9† for anaerobically-milled SiNP samples of HM-5 and MP-5 exposed to several small measured doses of O2 gas (the gas volumes used for the two samples were similar). For MP-5 the initial pressures of the five sequential doses of O2 were 2.4, 3.1, 2.9, 84.4, and 73.6 Torr. For HM-5 the initial pressures of the eight sequential doses of O2 were 2.0, 4.6, 3.0, 9.6, 73.6, 83.1, 141.6, and 142.7 Torr. A video showing the pyrophoric nature of a SiNP sample milled for 5.5 h in HEP is available from the authors.
Discussion
I. General comments
As discussed above, the goal of this study was to investigate the effects of (i) aerobic vs. rigorously anaerobic/anhydrous (hereinafter simply “anaerobic”) top-down nanoparticle preparation by attritor (i.e., stirred media) milling, (ii) aliphatic vs. aromatic hydrocarbons as the medium for suspending and transporting the particles to the milling chamber in a continuous loop, and (iii) short vs. long anaerobic milling times on nanoparticle shape, size, surface area, degree of crystallinity, surface/near-surface composition, and reactivity.
Anaerobic milling was performed in an inert-atmosphere glovebox continuously purged with purified N2 certified to contain less than 1 ppm O2 and less than 1 ppm H2O vapor. The anaerobic quality of the atmosphere was tested periodically between milling experiments by exposing the tungsten filament of a new 25 W light bulb to the glovebox atmosphere.62 The bulb burned continuously for at least 24 h, indicating that the combined concentrations of O2 and H2O vapor were 5 ppm or less.63
The concentrations of O2 in the HEP, HEP/MES, and MES/PYR slurries during aerobic milling are not known. The equilibrium concentrations of O2 in similar solvents in contact with dry air (21% O2) at 1 atm are 2.8 mM in n-octane64 and 1.8 mM in toluene65 (the concentration is probably higher than 1.8 mM in mesitylene because the solubility of O2 in aromatic hydrocarbons increases with increasing methyl substitution66). The slurries are mixtures of 460 mL solvent plus 20 g of SiNPs that probably vary in effective volume during milling (8.6 mL at the start of milling). Furthermore, during aerobic milling the circulating slurry is open to the air only briefly as it passes through the mechanically-stirred open slurry bucket, and therefore air/slurry gas–exchange equilibrium may not be established once milling begins. Finally, atmospheric pressure in Fort Collins, CO, is typically 635–640 Torr, significantly less than 1 atm. Therefore, an upper-limit estimate of the O2 concentration in our slurries during aerobic milling is ca. 2 mM.
The micrographs in Fig. 3 are typical of both aerobically- and anaerobically-milled samples in this study. The SiNPs have irregular shapes with dimensions that nominally range from 20 to 200 nm. The larger particles appear to be agglomerations of smaller particles. In general, attritor milling, like most top-down methods of nanoparticle preparation, is not expected to produce nanoparticles with a uniform shape or a narrow distribution of sizes,13,32,35,67 although Fink and co-workers have demonstrated a relatively narrow range of SiNP sizes by reactive high-energy ball milling (90% of the particles were between 1 and 4 nm).9
II. Aerobic milling
Surface/near-surface weighted XPS silicon peak intensities showed 3% SiO2 for MP(air)-5, 5% SiO2 for HM(air)-5, and 12% SiO2 for H(air)-5. These results demonstrate that an inert aliphatic hydrocarbon such as HEP did not “protect” the SiNP surfaces as much as the aromatic hydrocarbon MES as far as reaction with O2 during aerobic milling resulting in the formation of SiO2. This was true even when only a few percent of MES was present in the HEP milling fluid. Therefore, it is unlikely that this is a bulk solvent effect but instead is due to the greater reactivity of MES with the SiNP surfaces relative to HEP. The ATR-FTIR spectra in Fig. 6 show the presence of surface-bound MES even when the SiNPs were milled in HEP containing only a few percent of MES.
It is well known that aliphatic hydrocarbons are much less reactive than unsaturated or aromatic hydrocarbons. In addition, to our knowledge there is no precedent for the activation of an aliphatic C(sp3)–H bond by a Si surface, even under mechanochemical synthetic conditions. For example, Mitchell et al. found that unsaturated hydrocarbons with C(sp2)–H or C(sp)–H bonds such as 1-octene and 1-octyne, respectively, reacted with the surfaces of SiNPs formed by high-energy ball milling, but the fully-saturated aliphatic hydrocarbon octane did not.33,34 Furthermore, aromatic compounds such as benzene,43,68–72 toluene,72,73 p-xylene,74 naphthalene,75,76 and coronene77,78 have been shown to undergo strong chemisorption with certain faces of rigorously-cleaned Si with the formation of Si–C bonds.
III. Aerobic vs. anaerobic milling
A. Reactivity. In sharp contrast to the aerobically-milled SiNPs, all samples of anaerobically-milled SiNPs reacted visibly and vigorously when exposed to air. For example, the bottoms of glass scintillation vials containing ca. 50 mg samples of MP-2 and MP-6 became warm when the samples were rapidly exposed to air. In some experiments a small amount of smoke was observed. Samples of SiNPs milled anaerobically in HEP were even more reactive, igniting into a fireball when poured through air. The pyrophoric nature of a SiNP sample milled for 5.5 h in HEP is clearly evident in a video available from the authors.Samples of MP-5 and HM-5 reacted irreversibly with doses of dry O2, presumably by dissociative chemisorption and formation of Si–O–Si moieties on the surface, as shown in the plots of P(O2) vs. time in Fig. S-8.† Both samples reacted rapidly (minutes) and completely with small initial doses of 2–5 Torr O2, followed by treatments with doses up to 85 Torr O2 for MP-5 and 143 Torr O2 for HM-5, which were monitored until the uptake of O2 was negligible (i.e., ΔP(O2)/Δt ≤ 1 Torr h−1). The total amounts of O2 absorbed by the samples corresponded to 1.2 mmol O2 g−1 for MP-5 and 1.7 mmol O2 g−1 for HM-5. To put these results in perspective, the BET-determined surface areas of MP-5 and HM-5 correspond to a monolayer of N2 at −196 °C consisting of 2.6 mmol N2 g−1 for MP-5 and 3.0 mmol N2 g−1 HM-5. Therefore, the HEP/MES milled SiNPs reacted with proportionally more O2 than the MES/PYR milled SiNPs per unit surface area (i.e., 42% more O2 for HM-5, which has a surface area only 14% larger than MP-5). The reactions of dry O2 with clean, clearly-defined silicon surfaces such as Si(100) and Si(111) at ambient temperatures have been very well studied both experimentally and theoretically.79–83 The mechanisms, stoichiometry, and speciation of Si–O moieties formed as a function of time are very complicated and depend on many factors. Consequently, other than noting that HM-5 appears to be more reactive towards O2 than MP-5, we are not implying anything quantitative about the nature, surface coverage, or number of layers of Si–O species formed by comparing the uptake of O2 by these two samples.
B. Nanoparticle surface area. When neat HEP was the milling fluid, aerobic milling resulted in SiNPs with four times the surface area of anaerobically-milled SiNPs (169 m2 g−1 for H(air)-5 vs. 40 m2 g−1 for H-5). Using a vibrating micromill connected to a vacuum/gas-handling system to produce SiNPs from silicon powder, Butyagin et al. found that an atmosphere of 10 or 100 Torr O2 resulted in SiNPs with more than two times the surface area of SiNPs milled under vacuum.84 Thus, in the absence of a milling fluid or with a presumably chemically-inert milling fluid such as HEP (see below), anaerobic milling resulted in SiNPs with larger diameters relative to aerobic milling.In contrast, when the milling fluid contained mesitylene, anaerobic milling resulted in SiNPs with larger surface areas per gram (and hence with SiNPs having smaller diameters) relative to SiNPs milled aerobically. When only a small amount of mesitylene was added to heptane, the BET-SA of the anaerobically-milled SiNPs was 21% larger (295 m2 g−1 for HM-5.5 vs. 243 m2 g−1 for HM(air)-5). When mesitylene containing 22 mM pyrene was the milling fluid, the BET-SA of the anaerobically-milled SiNPs was twice as large (258 m2 g−1 for MP-5 vs. 130 m2 g−1 for MP(air)-5). These comparisons are shown graphically in Fig. S-9.† The effects of HEP vs. MES/PYR on the limiting NP size and surface area (i.e., limiting fineness) is discussed below in Section IV.
C. Surface/near-surface composition determined by weighted XPS peak integration. As expected, aerobic milling resulted in a higher percentage of O atoms in the surface/near-surface environment, by at least a factor of three, than anaerobic milling (33% O for H(air)-5 vs. 12% for H-5; 32% O for HM(air)-5 vs. 6% for HM-5; and 20% O for MP(air)-5 vs. 7% for MP-5). Furthermore, the aerobically milled samples exhibited a SiOx band ca. two or three times as intense as the corresponding anaerobically milled samples (x < 2; 7% SiOx for H(air)-5 vs. 4% for H-5; 9% SiOx for HM(air)-5 vs. 3% for HM-5; and 5% SiOx for MP(air)-5 vs. 3% for MP-5). Significantly, in contrast to the aerobically milled samples, the Si XPS envelope for anaerobically milled SiNP samples did not exhibit an SiO2 band at 103.2 ± 0.6 eV (12%, 5%, and 3% SiO2 for H(air)-5, HM(air)-5, and MP(air)-5, respectively, vs. 0% SiO2 for H-5, HM-5, and MP-5).Further evidence in support of significantly different surface compositions for anaerobically and aerobically milled SiNPs was shown by the following experiment. Samples of H-5 and H(air)-5 were suspended in heptane using a probe sonicator. The particles of H-5 remained suspended for longer than 30 min. The particles of H(air)-5 settled out almost immediately even though, on average, particles of H(air)-5 were smaller, nominally by a factor of two, than particles of H-5 (recall that the BET-SAs are 169 m2 g−1 for H(air)-5 and only 40 m2 g−1 for H-5).
D. ATR-FTIR spectra. The spectra of anaerobically and aerobically milled SiNP samples were surprisingly similar in the ν(CH) spectral region. Since the spectra could not be recorded in the absence of air, we cannot rule out contamination of the samples with adventitious hydrocarbon vapors (such contamination of SiNPs has been routinely observed1). For this reason, the presence of characteristic aliphatic ν(CH) bands in the HEP milled samples may not indicate the presence of chemisorbed aliphatic hydrocarbyl moieties on the surface of the SiNPs. However, the presence of ν(CH) bands due to MES, even after prolonged evacuation of the samples to remove weakly physisorbed MES, strongly suggests that MES or MES-derived hydrocarbyl species are strongly bound to the surfaces of HM-5, HM(air)-5, MP-5, and MP(air)-5. The clear presence of MES-like ν(CH) bands in the spectra of HM-5 and HM(air)-5 further demonstrates the greater reactivity of aromatic hydrocarbons with SiNP surfaces relative to aliphatic hydrocarbons.
IV. Anaerobic milling. The effects of milling fluid and milling time on SiNP properties
The TEM and PXRD results indicate that the SiNPs became less crystalline, or more amorphous, as the milling time increased for anaerobic milling in both HEP and MES/PYR. This is in harmony with results reported for anaerobically ball-milled Eu-doped Y2O3 NPs,10 for aerobically ball-milled coconut shell NPs,24 and for SiNPs prepared aerobically in 1-butanol using a stirred media mill.1 In the latter study, the average crystallite size decreased by 50% and the crystalline volume decreased by a factor of 3 after 24 h of milling.1 The process of amorphization during high-energy milling is well documented.19,85
For any milling time period, the BET-SAs of SiNPs anaerobically milled in MES/PYR or in HEP/MES were significantly larger than for SiNPs milled in HEP. Furthermore, the BET-SA of MES/PYR-milled SiNPs increased from 75 to 258 m2 g−1 as the milling time increased from 1 to 5 h, whereas the BET-SA of the HEP-milled SiNPs remained constant at 39 ± 6 m2 g−1 from 1 to 5 h. In addition, the BET-SAs of MP-5 and HM-5 were similar, 258 and 295 m2 g−1, respectively. Interestingly, anaerobic MES/PYR milled SiNPs had an estimated average crystallite size of 14 nm after 1 h whereas anaerobic HEP milled SiNPs had a considerably larger average crystallite size of 43 nm after 1 h, which only dropped to 13 nm after 3 h of milling.
The HEP-milled SiNPs apparently reached the true grinding limit (i.e., the grinding limit defined by the maximum BET-SA)86 sooner, and with larger primary particles, than HEP/MES- or MES/PYR-milled SiNPs. (A primary particle is defined as a nonporous spherical particle with a theoretical density of the bulk material. With the priviso that the SiNPs produced in this work are neither monomodal in size nor spherical, the effective primary particle diameters of HM-5, MP-5, and H-5 are 9, 10, and 64 nm, respectively).
The difference in limiting particle size cannot be attributed to a bulk solvent effect (cf. HM-5 with H-5). Furthermore, it cannot be attributed to differences in the degree of SiNP crystallinity as measured by PXRD because the BET-SA values of H-1, H-2, and H-3 are essentially constant even though their average crystallite sizes decreased from 43 to 22 to 13 nm, respectively. In addition, the average crystallite sizes of MP-1, MP-2, and MP-5 only decreased from 14 to 11 to 9 nm, respectively, while their BET-SA values increased from 75 to 162 to 258 m2 g−1, respectively. Moreover, differences in particle agglomeration, which can affect the apparent grinding limit but not the true grinding limit,86 and which might be affected by differences in steric stabilization by surface-bound aliphatic vs. aromatic molecular fragments, cannot explain the BET-SA differences. Our results show that mechanochemical equilibrium, the point at which the rates of primary particle size reduction and primary particle size enlargement are the same, is apparently very sensitive to the presence of aromatic molecules or aromatic molecular fragments on the surfaces of the SiNPs.
As particles decrease in size, attractive interactions between particle surfaces can prevent further comminution.19,85 One possible explanation of our results is that SiNPs milled anaerobically in HEP have more reactive surfaces than SiNPs milled anaerobically in the presence of aromatic molecules, perhaps because the HEP-milled particles have more exposed Si atoms that can more effectively reform Si–Si bonds, and hence larger particles, by particle–particle collisions. In this regard, note that the weighted XPS peak-integration % C is 62% for MP-5, 55% for HM-5, but only 26% for H-5. The corresponding % Si(Si) values are 18% for MP-5, 21% for HM-5, and 44% for H-5. The proposed high reactivity of H-5 surfaces with respect to reformation of larger particles is supported by the observation that SiNPs milled aerobically in 100% HEP were nominally two times smaller (the BET-SAs of H-5 and H(air)-5 are 40 and 169 m2 g−1, respectively). The corresponding XPS % Si(Si) and % O values are 44 and 12%, respectively, for H-5 and 10 and 33%, respectively, for H(air)-5. These results are similar to those reported by Dorofeev and co-workers, who found that titanium NPs milled in the aromatic solvent toluene were ca. ten times smaller than the NPs milled in heptane.87 Furthermore, they reported that the wt% of C and H on the TiNPs was more than twice as high when they were milled in toluene than when they were milled in heptane.87
Another possibility is that the morphology of SiNPs milled anaerobically in the presence of aromatic molecules is significantly different than SiNPs milled anaerobically in 100% HEP and that this difference can affect the re-growth of SiNPs by particle–particle collisions. Unfortunately, in this initial study we were not able to compare the effect of anaerobic aliphatic-solvent- vs. aromatic-solvent-milling on SiNP morphology in an unambiguous way. Nevertheless, it is known that the morphology of NPs prepared in stirred-media mills can be affected by the solvent used. For example, comminution of pieces of amorphous glass produced irregularly-shaped NPs when milled in water but plate-like NPs when milled in 2-propanol or benzyl alcohol.88
Conclusions
Rigorously-anaerobic stirred media milling of silicon can produce nanoparticles (SiNPs) with significantly different physical and spectroscopic properties than SiNPs milled aerobically. Furthermore, those properties are strongly affected by the presence or absence of aromatic molecules in the milling medium. Perhaps the most interesting results are that the SiNPs milled anaerobically in the aromatic hydrocarbon solvent mesitylene (MES) are up to two times smaller than those milled aerobically in MES, but the SiNPs milled anaerobically in the aliphatic hydrocarbon solvent heptane (HEP) are four times larger than those milled aerobically in HEP.
Acknowledgements
The authors thank Dr T. D. Newbound and J. Norris of Kratos LLC for financial support, for the use of their Netzsch MiniCer mill, and for helpful discussions. We also acknowledge the Colorado State University Foundation for financial support and E. P. Stuckert, M. J. Hawker, P. R. McCurdy, and R. H. Geiss for experimental assistance and helpful discussions.
References
- A. Reindl, S. Aldabergergenova, E. Altin, G. Frank and W. Peukert, Phys. Status Solidi A, 2007, 204, 2329–2338 CrossRef CAS.
- A. Monteiro, A. Afolabi and E. Bilgili, Drug Dev. Ind. Pharm., 2013, 39, 266–283 CrossRef CAS PubMed.
- B. W. McMahon, J. P. L. Perez, J. Yu, J. A. Boatz and S. L. Anderson, ACS Appl. Mater. Interfaces, 2014, 6, 19579–19591 CAS.
- A. B. G. Simpson, J. A. Byrne, J. A. D. McLaughlin and M. Strawhorne, Chem. Eng. Res. Des., 2015, 93, 287–292 CrossRef CAS.
- Z. Zhang, F. Huo, X. Zhang and D. Guo, Scr. Mater., 2012, 67, 657–660 CrossRef CAS.
- S. Fadda, A. Cincotti, A. Concas, M. Pisu and G. Cao, Powder Technol., 2009, 194, 207–216 CrossRef CAS.
- N. G. Akdogan, G. C. Hadjipanayis and D. J. Sellmyer, J. Appl. Phys., 2009, 105, 07A710 Search PubMed.
- T. Xing, J. Sunarso, W. Yang, Y. Yin, A. M. Glushenkov, L. H. Li, P. C. Howlett and Y. Chen, Nanoscale, 2013, 5, 7970–7976 RSC.
- L. Kuang, B. S. Mitchell and M. J. Fink, J. Exp. Nanosci., 2014, 10, 1214–1222 CrossRef.
- T. L. Phan, D. N. Chung, P. D. Thang, P. T. Huyen, T. V. Manh, T. A. Ho, T. D. Thanh, N. M. Vuong, B. W. Lee and S. C. Yu, Mater. Trans., 2015, 56, 1412–1415 CrossRef CAS.
- H. Wang, Z. Xu, M. J. Fink, D. Shchukin and B. S. Mitchell, Chem. Commun., 2015, 51, 1465–1468 RSC.
- J. Hwang, Y. Jeong, K. H. Lee, Y. Seo, J. Kim, J. W. Hong, E. Kamaloo, T. A. Camesano and J. Choi, Ind. Eng. Chem. Res., 2015, 54, 5982–5989 CrossRef CAS and references therein.
- M. A. Basith, D.-T. Ngo, A. Quader, M. A. Rahman, B. L. Sinha, B. Ahmmad, F. Hirose and K. Mølhave, Nanoscale, 2014, 6, 14336–14342 RSC.
- N. Luo, C. Yang, X. Tian, J. Xiao, J. Liu, F. Chen, D. Zhang, D. Xu, Y. Zhang, G. Yang, D. Chen and L. Li, J. Mater. Chem. B, 2014, 2, 5891–5897 RSC.
- A. Jankovic, Miner. Eng., 2003, 16, 337–345 CrossRef CAS.
- D. L. Zhang, Prog. Mater. Sci., 2004, 49, 537–560 CrossRef CAS.
- F. Stenger, S. Mende, J. Schwedes and W. Peukert, Chem. Eng. Sci., 2005, 60, 4557–4565 CrossRef CAS.
- W. Peukert, H.-C. Schwarzer and F. Stenger, Chemical Engineering and Processing: Process Intensification, 2005, 44, 245–252 CrossRef CAS.
- P. Baláž, in Mechanochemistry in Nanoscience and Minerals Engineering, Springer-Verlag, Berlin Heidelberg, 2008, ch. 2, p. 413 Search PubMed.
- C. Knieke, C. Steinborn, S. Romeis, W. Peukert, S. Breitung-Faes and A. Kwade, Chem. Eng. Technol., 2010, 33, 1401–1411 CrossRef CAS.
- C. Knieke, S. Romeis and W. Peukert, AIChE J., 2011, 57, 1751–1758 CrossRef CAS.
- P. R. Pradeep and B. Pitchumani, Asia-Pac. J. Chem. Eng., 2011, 6, 154–162 CrossRef CAS.
- P. Baláž, M. Achimovičová, M. Baláž, P. Billik, Z. Cherkezova-Zheleva, J. M. Criado, F. Delogu, E. Dutková, E. Gaffet, F. J. Gotor, R. Kumar, I. Mitov, T. Rojac, M. Senna, A. Streletskii and K. Wieczorek-Ciurowa, Chem. Soc. Rev., 2013, 42, 7571–7637 RSC.
- S. A. Bello, J. O. Agunsoye and S. B. Hassan, Mater. Lett., 2015, 159, 514–519 CrossRef CAS.
- J. V. Williams, N. A. Kotov and P. E. Savage, Ind. Eng. Chem. Res., 2009, 48, 4316–4321 CrossRef CAS.
- M. E. Anderson, S. S. N. Bharadwaya and R. E. Schaak, J. Mater. Chem., 2010, 20, 8362–8367 RSC.
- B. M. Nolan, T. Henneberger, M. Waibel, T. F. Fässler and S. M. Kauzlarich, Inorg. Chem., 2015, 54, 396–401 CrossRef CAS PubMed.
- U. Ghorpade, M. Suryawanshi, S. W. Shin, K. Gurav, P. Patil, S. Pawar, C. W. Hong, J. H. Kim and S. Kolekar, Chem. Commun., 2014, 50, 11258–11273 RSC.
- K. V. Mahesh, S. K. Singh and M. Gulati, Powder Technol., 2014, 256, 436–449 CrossRef CAS.
- C. Wan and B. M. Leonard, Chem. Mater., 2015, 27, 4281–4288 CrossRef CAS.
- A. Malumbres, G. Martínez, J. L. Hueso, J. Gracia, R. Mallada, A. Ibarra and J. Santamaria, Nanoscale, 2015, 7, 8566–8573 RSC.
- G. Priyadarshana, N. Kottegoda, A. Senaratne, A. de Alwis and V. Karunaratne, J. Nanomater., 2015, 317312 Search PubMed.
- A. S. Heintz, M. J. Fink and B. S. Mitchell, Adv. Mater., 2007, 19, 3984–3988 CrossRef CAS.
- A. S. Heintz, M. J. Fink and B. S. Mitchell, Appl. Organomet. Chem., 2010, 24, 236–240 CrossRef CAS.
- T. Wakihara, A. Ihara, S. Inagaki, J. Tatami, K. Sato, K. Komeya, T. Meguro, Y. Kubota and A. Nakahira, Cryst. Growth Des., 2011, 11, 5153–5158 CAS.
- H. K. Han, C. Loka, Y. M. Yang, J. H. Kim, S. W. Moon, J. S. Cho and K.-S. Lee, J. Power Sources, 2015, 281, 293–300 CrossRef CAS.
- R. Shuba and I.-W. Chen, J. Am. Ceram. Soc., 2006, 89, 1110–1113 CrossRef CAS.
- S. Bhattacharjee, I. M. C. M. Rietjens, M. P. Singh, T. M. Atkins, T. K. Purkait, Z. Xu, S. Regli, A. Shukaliak, R. J. Clark, B. S. Mitchell, G. M. Alink, A. T. M. Marcelis, M. J. Fink, J. G. C. Veinot, S. M. Kauzlarich and H. Zuilhof, Nanoscale, 2013, 5, 4870–4883 RSC , and references therein.
- J. H. Dymond and H. A. Øye, J. Phys. Chem. Ref. Data, 1994, 23, 41–53 CrossRef CAS.
- J. D. L. S. López-Lázaro, G. A. Iglesias-Silva, A. Estrada-Baltazar and J. Barajas-Fernández, J. Chem. Eng. Data, 2015, 60, 1823–1834 CrossRef.
- J. A. Al-Kandary, A. S. Al-Jimaz and A.-H. M. Abdul-Latif, J. Chem. Eng. Data, 2006, 51, 2074–2082 CrossRef CAS.
- C. Pan, Q. Ke, G. Ouyang, X. Zhen, Y. Yang and Z. Huang, J. Chem. Eng. Data, 2004, 49, 1839–1842 CrossRef CAS.
- F. Tao, S. L. Bernasek and G.-Q. Xu, Chem. Rev., 2009, 109, 3991–4024 CrossRef CAS PubMed.
- W.-C. Zhao, H. Wang, X.-M. Ou, C.-S. Lee and X.-H. Zhang, Appl. Phys. Lett., 2011, 98, 253101–253103 CrossRef.
- Y. Liu, Z. Yu, S. Zhou and L. Wu, J. Dispersion Sci. Technol., 2006, 27, 983–990 CrossRef CAS.
- A. Dromgoole, Personal communication, Netzsch Premier Technologies, LLC, 2016 Search PubMed.
- E. V. Bukovsky, Ph.D. Dissertation, Colorado State University, 2015.
- S. Brunauer, P. H. Emmett and E. Teller, J. Am. Chem. Soc., 1937, 59, 1553–1564 CrossRef.
- S. Brunauer, P. H. Emmett and E. Teller, J. Am. Chem. Soc., 1938, 60, 309–319 CrossRef CAS.
- A. M. Chockla, J. T. Harris, V. A. Akhavan, T. D. Bogart, V. C. Holmberg, C. Steinhagen, C. B. Mullins, K. J. Stevenson and B. A. Korgel, J. Am. Chem. Soc., 2011, 133, 20914–20921 CrossRef CAS PubMed.
- D. Wheeler and S. Pepper, Surf. Interface Anal., 1987, 10, 153–162 CrossRef CAS.
- T. P. Nguyen and S. Lefrant, J. Phys.: Condens. Matter, 1989, 1, 5197 CrossRef CAS.
- G. Jaksa, B. Stefane and J. Kovac, Surf. Interface Anal., 2013, 45, 1709–1713 CrossRef CAS.
- N. Shirahata, T. Yonezawa, W.-S. Seo and K. Koumoto, Langmuir, 2004, 20, 1517–1520 CrossRef CAS PubMed.
- S. Seal, S. Krezoski, D. Petering and T. L. Barr, Appl. Surf. Sci., 2001, 173, 339–351 CrossRef CAS.
- E. Radvanyi, E. De Vito, W. Porcher and S. Jouanneau Si Larbi, J. Anal. At. Spectrom., 2014, 29, 1120–1131 RSC.
- B. Philippe, R. Dedryvere, M. Gorgoi, H. Rensmo, D. Gonbeau and K. Edstrom, Chem. Mater., 2013, 25, 394–404 CrossRef CAS.
- M. Y. Nie, D. P. Abraham, Y. J. Chen, A. Bose and B. L. Lucht, J. Phys. Chem. C, 2013, 117, 13403–13412 CAS.
- C. Önneby and C. G. Pantano, J. Vac. Sci. Technol., A, 1997, 15, 1597–1602 Search PubMed.
- K. Miyoshi and D. H. Buckley, Appl. Surf. Sci., 1982, 10, 357–376 CrossRef CAS.
- G. B. Smith, D. R. McKenzie and P. J. Martin, Phys. Status Solidi B, 1989, 152, 475–480 CrossRef CAS.
- D. F. Shriver and M. A. Drezdzon, The Manipulation of Air-sensitive Compounds, Wiley-Interscience, New York, 2nd edn, 1986 Search PubMed.
- I. D. Eubanks and F. J. Abbott, Anal. Chem., 1969, 41, 1708–1709 CrossRef CAS.
- J. A. Petrocelli and D. H. Lichtenfels, Anal. Chem., 1959, 31, 2017–2019 CrossRef CAS.
- B. M. Monroe, Photochem. Photobiol., 1982, 35, 863–865 CrossRef CAS.
- H. L. Clever, R. Battino, H. Miyamoto and Y. Yampolski, J. Phys. Chem. Ref. Data, 2014, 43, 033102 CrossRef.
- J. Koruza, B. Malič, O. Noshchenko and M. Kosec, J. Nanomater., 2012, 469143 Search PubMed.
- M. Carbone, M. N. Piancastelli, M. P. Casaletto, R. Zanoni, G. Comtet, G. Dujardin and L. Hellner, Phys. Rev. B: Condens. Matter Mater. Phys., 2000, 61, 8531–8536 CrossRef CAS.
- Y. Taguchi, M. Fujisawa, T. Takaoka, T. Okada and M. Nishijima, J. Chem. Phys., 2001, 95, 6870–6876 CrossRef.
- K. R. Harikumar, J. C. Polanyi and A. Zabet-Khosousi, Surf. Sci., 2012, 606, 1431–1434 CrossRef CAS.
- R. Coustel, O. Pluchery, N. Witkowski and Y. Borensztein, J. Phys. Chem. C, 2014, 118, 10740–10745 CAS.
- D. Lock, S. Sakulsermsuk, R. E. Palmer and P. A. Sloan, J. Phys.: Condens. Matter, 2015, 27, 054003 CrossRef CAS PubMed.
- H. Tomimoto, T. Takehara, K. Fukawa, R. Sumii, T. Sekitani and K. Tanaka, Surf. Sci., 2003, 526, 341–350 CrossRef CAS.
- Q. Li, Z. H. He, X. J. Zhou, X. Yang and K. T. Leung, Surf. Sci., 2004, 560, 191–204 CrossRef CAS.
- K. Okamura, H. Ishii, Y. Kimura and M. Niwano, Surf. Sci., 2005, 576, 45–55 CrossRef CAS.
- K. S. Yong, Y. P. Zhang, S.-W. Yang and Q. X. Xu, Surf. Sci., 2008, 602, 1921–1927 CrossRef CAS.
- T. Suzuki, D. C. Sorescu, K. D. Jordan, J. Levy and J. T. Yates Jr, J. Chem. Phys., 2006, 124, 054701 CrossRef PubMed.
- J. Martínez-Blanco and K. H. Klingsporn, Surf. Sci., 2010, 604, 523–528 CrossRef.
- T. Engel, Surf. Sci. Rep., 1993, 18, 91–144 CrossRef CAS , and references therein.
- M. A. Pamungkas, M. Joe, B.-H. Kim and K.-R. Lee, J. Appl. Phys., 2011, 110, 053513 CrossRef , and references therein.
- S. Wang, J. He, Y. Zhang and G. Q. Xu, Surf. Sci., 2012, 606, 1387–1392 CrossRef CAS , and references therein.
- D. Flötotto, Z. M. Wang, L. P. H. Jeurgens and E. J. Mittemeijer, J. Appl. Phys., 2014, 115, 023501 CrossRef , and references therein.
- A. Yoshigoe and Y. Teraoka, J. Phys. Chem. C, 2014, 118, 9436–9442 CAS , and references therein.
- P. Y. Butyagin, A. N. Streletskii, I. V. Berestetskaya and A. B. Borunova, Colloid J., 2001, 63, 639–644 CrossRef CAS.
- M. Sommer, F. Stenger, W. Peukert and N. J. Wagner, Chem. Eng. Sci., 2006, 61, 135–148 CrossRef CAS.
- C. Knieke, M. Sommer and W. Peukert, Powder Technol., 2009, 195, 25–30 CrossRef CAS.
- G. A. Dorofeev, V. I. Ladyanov, A. N. Lubnin, V. V. Mukhgalin, O. M. Kanunnikova, S. S. Mikhailova and V. V. Aksenova, Int. J. Hydrogen Energy, 2014, 39, 9690–9699 CrossRef CAS.
- A. Vital, S. Zürcher, R. Dittmann, M. Trottmann, P. Lienemann, B. Bommer, T. Graule, E. Apel and W. Höland, Chem. Eng. Sci., 2008, 63, 484–494 CrossRef CAS.
Footnote |
† Electronic supplementary information (ESI) available: Schematic drawing and photographs of the attritor mill housed in a glovebox used in this work, a table of literature XPS peak positions, additional figures, and information about the estimation of crystallite sizes from PXRD peak widths. A video of pyrophoric SiNPs prepared anaerobically being poured in air can be obtained from the authors. See DOI: 10.1039/c6ra19565b |
|
This journal is © The Royal Society of Chemistry 2016 |