DOI:
10.1039/C6RA19431A
(Paper)
RSC Adv., 2016,
6, 94682-94686
Synthesis of Ag nanoparticles decorated MnO2/sulfonated graphene composites with 3D macroporous structure for high performance capacitors electrode materials†
Received
1st August 2016
, Accepted 25th September 2016
First published on 28th September 2016
Abstract
An effective strategy to improve the conductivity of MnO2-based electrodes is to combine them with some conductive materials, such as metal or carbon-based materials. In this work, Ag nanoparticles decorated MnO2/Sulfonated Graphene composite with 3D macroporous structure (3D Ag–MnO2/SG) was synthesized and their performance as electrode material in supercapacitors was studied. The improved conductivity and the elaborate design of 3D porous structure allowed the 3D Ag–MnO2/SG electrode to possess enhanced electrochemical performance. For example, the electrochemical testing results demonstrated that the 3D Ag–MnO2/SG presents a capacitance of 537 F g−1 at 0.5 A g−1, which is much higher than that of MnO2 (∼172 F g−1). Moreover, the 3D Ag–MnO2/SG electrode delivers a higher capacity retention rate in high current density and smaller charge transfer resistance than that of MnO2, which is contributed by the synergistic effect from the Ag nanoparticles and the 3D porous SG.
1. Introduction
The shortage of fossil fuels has hindered the development of industry; it is extremely urgent to explore new energy storage systems. Supercapacitors have been considered promising devices for their fast charge and discharge rates, high power density, long cycle lifetime and safety, which have considerable prospects in many fields, such as wind power, electrical vehicle, mobile communications, etc.1–3 Considerable research efforts have been focused on the supercapacitors in recent years, and the results indicate that the performance of supercapacitors is mainly related to the property of their electrode materials. Among the various electrode materials reported, manganese oxide (MnO2) has attracted the most attention because of its cost effectiveness, environmentally friendliness and high theoretical capacity (1370 F g−1).4–7 However, MnO2 suffers the poor electrical conductivity (range from 10−6 to 10−5 S cm−1), leading to an unsatisfactory capacitive performance and poor cycling performance.8
In order to circumvent these problems, scientists devoted themselves to combine MnO2 with some conductive materials, such as carbon materials,9–12 metal nanoparticles13,14 and conducting polymers.15,16 For example, Zhou et al.8 prepared a sandwich-like graphene/MnO2 nanosphere by a filtration-directed self-assembly method. Benefit from the graphene film, a high specific capacitance of 446 F g−1 at 5 mV s−1 was obtained and a capacitance retention rate of 96% after 1000 cycles was achieved. Xia et al.17 prepared a hierarchical heterostructure of Ag/MnO2 nanowires, the as-designed Ag/MnO2 hybrid electrode delivers a specific capacitance of 293 F g−1. Although graphene/MnO2 materials exhibit excellent capacity behavior, the electrolyte access and electron transportation property are unsatisfactory and hindered the full use of its properties. What's more, the traditional preparation process for the Ag nanoparticles need about a week. In view of these, a simple process for synthesis of Ag nanoparticles and reasonable design of architecture that allows for easy electrolyte penetration are needed.
Here, Ag nanoparticles decorated MnO2/sulfonated graphene composite with 3D macroporous structure was synthesized, which combined the advantages of the Ag nanoparticles and sulfonated graphene. Moreover, the 3D porous graphene skeleton can increase the surface areas,18 mitigate the aggregation of the SG sheets and provide fast ion/electron transfer paths,19 therefore it was expected to enhance the stability and specific capacitance of the electrodes.20 For example, Zhang et al.21 reported a facile synthesis of 3D MnO2–graphene foam, the as-prepared 3D MnO2–graphene foam possess large accessible surface area, high chemical stability and excellent flexibility in the application of flexible all-solid-state asymmetric supercapacitors.
2. Experimental
2.1. Materials and chemicals
All chemicals were used as received without further purification. Sulfonated graphene (3–5 layers, the thickness is 1.0–1.7 nm) was obtained from Graphene-Tech. Polystyrene nanobeads (300 ± 5 nm, the solid content is 5%) was obtained from Janus New-materials. Potassium permanganate (KMnO4, ≥99.5%, AR) was obtain from the Yantai Sanhe Chemical Co., LTD. Carbon black and nickel foam were supplied by Shanghai Xiaoyuan Energy Technology Co., Ltd. Polytetrafluoroethylene (PTFE, 60%), anhydrous sodium sulfate (Na2SO4, ≥99%) and other chemical reagents used in experiments were purchased from Aladdin Reagent.
2.2. Synthesis of 3D SG/MnO2
In a typical preparation, 40 mg sulfonated graphene was added into the deionized water, followed by a sonication process for 1 h. In the meantime, the PS dispersion (300 ± 5 nm) was sonicated for 30 min for preventing the aggregation. Then, 2 mL of the above PS suspension was dropped into the SG suspension. After sonicated for 60 min, the resulting mixture was dried at room temperature for 12 h. Further calcination was conducted in a quartz tube furnace. The sample was kept at 450 °C (the heating rate was set to 1 °C min−1) for three hours in a nitrogen atmosphere and the 3D porous SG structure was obtained after the PS particles was removed. To get 3D SG/MnO2, appropriate KMnO4 was added into the 3D SG dispersion and stirred for 1 h. Then, the mixture was poured into the Teflon-lined stainless steel autoclave and reacted at 150 °C for 6 h. The product was collected by vacuum filtration, washed with DI water and ethanol, and then dried at 60 °C overnight. As a comparison, the pure MnO2 was also prepared in the same way without adding 3D SG.
2.3. Synthesis of 3D Ag–MnO2/SG
50 mg of the 3D MnO2/SG was dispersed in 50 mL deionized water followed by a sonication process for 0.5 h. In the meantime, 5 mg of AgNO3 and 5 mL of NaOH solution (1 M) was added into the dispersion, successively. After stirred for 3 h, the resulting mixture was transferred into a 200 mL round-bottomed flask and refluxed at 90 °C for 1 h. The final product was collected by vacuum filtration, washed with DI water and ethanol, and then dried in a vacuum oven at 60 °C overnight.
2.4. Material characterization
The crystal structures of the products were characterized by an X-ray diffractometer (XRD; D/Max 2500 V PC, Cu-Kα radiation) with a scan speed of 2° min−1. The morphology of the products was observed by using scanning electron microscopy (SEM, HITACHI S-3400N), which were operated at 5 kV. The inside details of the two kinds of structures were observed by transmission electron microscopy (TEM), which were carried out on a JEM-2100 with an accelerating voltage of 100 kV.
2.5. Electrochemical characterization
The working electrodes were prepared by pressing the mixture of 80 wt% as-prepared materials, 10 wt% carbon black and 10 wt% PTFE binder onto nickel foam and dried in a vacuum oven at 80 °C over 12 h. Electrochemical measurements (GAMRY Reference 3000 electrochemistry workstation) were conducted in a 1 M Na2SO4 aqueous solution using a three-electrode mode, with platinum electrode as the counter electrode and SCE (saturated calomel electrode) as the reference electrode.
3. Results and discussion
3.1. Characterization
XRD patterns of the 3D SG, 3D MnO2/SG, 3D Ag–MnO2/SG are shown in Fig. 1. Remarkably, a broad diffraction peak between 20° and 35° is observed for 3D SG, which matched well with previous reports related to sulfonated graphene nanosheets.22–24 For MnO2, three diffraction peaks at 2θ = 12.8° (110), 37.1° (211) and 65.6° (002) were observed, correlate well with the standard values for the α-phase MnO2 (JCPDS no. 44-0141).23,25 In the 3D Ag–MnO2/SG, four strong peaks located at about 38.1°, 44.2°, 64.5°, and 77.4°, which can be indexed to the (111), (200), (220), and (311), respectively, corresponded well with standard pattern of the metal Ag (JCPDS no. 65-2871).26,27 The XRD results demonstrated the successful synthesis of the Ag–MnO2/SG.
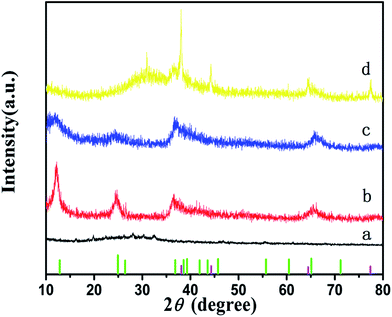 |
| Fig. 1 XRD patterns of the (a) 3D SG, (b) MnO2, (c) 3D MnO2/SG, (d) 3D Ag–MnO2/SG and the standard diffraction of α-phase MnO2 (JCPDS no. 44-0141) [the green line], the standard diffraction of Ag (JCPDS no. 65-2871) [the purple line]. | |
Scanning electron microscopy (SEM) images of the 3D porous SG and the 3D MnO2/SG are shown in Fig. 2a and b, respectively. As the result of pyrogenic decomposition of the PS particles, the hollow SG nanobeads were generated with well-ordered 3D porous structure and the average diameter of the hollow beads is ∼300 nm (Fig. 2a). The cross-sectional SEM image of the 3D porous SG film (Fig. S1, ESI†) illustrates that whether on the surface or in the internal part, the 3D porous morphology is ubiquitous. In order to further demonstrate the 3D porous framework, TEM analysis was carried out. As shown in Fig. S2 (ESI†), all the hollow beads are connected with each other and 3D interpenetrating networks are formed, which would provide a fast transport channel for electrolytes. This is important for the high performance supercapacitors.
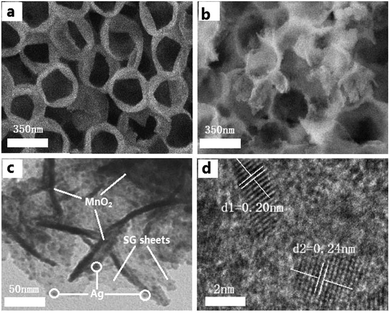 |
| Fig. 2 (a) SEM image of the 3D SG; (b) SEM image of the 3D MnO2/SG; (c) TEM image of the 3D Ag–MnO2/SG; (d) HRTEM image of the 3D Ag–MnO2/SG. | |
Fig. 2b shows the SEM images of the 3D MnO2/SG. Obviously, the MnO2 nanoparticles were deposited on the surface of SG hollow structure successfully. As the same time, the uniform morphology of 3D SG architecture is still intact even suffered through a high pressure threat during the hydrothermal process (Fig. S3, ESI†), reflecting the good mechanical stability of the self-supported 3D SG frame. After decorated with Ag particles, the existence of MnO2 and Ag was further confirmed by the TEM and high-resolution TEM (HRTEM). According to the TEM images at a low magnification, some black dots with an average diameter of 8 nm were observed. From the HRTEM image (Fig. 2d), we can see the lattice fringe with lattice spacing of 0.24 nm, which can be indexed as the (111) plane of the Ag particles.28 The distribution of these Ag particles on the surface of the SG sheets and the MnO2 can not only enhanced the conductivity of the composites, but also prevent the SG sheets from accumulation.28
The energy-dispersive X-ray spectroscopy (EDS) line-scanning result of the 3D Ag–MnO2/SG is shown in Fig. S4 (ESI†). The detectable elements in 3D Ag–MnO2/SG include carbon, sulfur, manganese, oxygen elements and silver, which further demonstrated the formation of Ag–MnO2/SG composites. EDS element maps were also given in Fig. S5 (ESI†), illustrated the uniform distribution of silver nanoparticles.
3.2. Electrochemical measurements
The electrochemical performances of MnO2 electrodes, 3D MnO2/SG electrodes and 3D Ag–MnO2/SG electrodes have been evaluated by a three-electrode system. Fig. 3a shows the cyclic voltammetry (CV) curves of MnO2 electrodes, 3D MnO2/SG electrodes and 3D Ag–MnO2/SG electrodes, which were carried under scan rate of 5 mV s−1 within the potential window of 0.0–1.0 V (vs. SCE). The symmetric and quasi-rectangular shape curves indicated the electrical double layer capacitive behavior.29,30 For the specific capacitance is directly proportional to the CV integration areas,31–33 we can see that 3D Ag–MnO2/SG electrode possess enhanced charge stored ability than MnO2 and MnO2/SG samples, which indicates the composite of the 3D SG and Ag particles can improve the performance of MnO2. CV curves of 3D Ag–MnO2/SG electrodes over a range of scan rates from 5 to 100 mV s−1 are shown in Fig. 3b. Even at a high scan rate of 100 mV s−1, the CV curve still retains a quasi-rectangle shape, indicating the fast charge propagation capability and facile ion transport of the obtained 3D Ag–MnO2/SG materials.
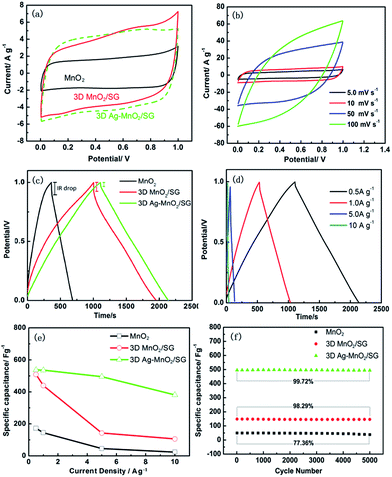 |
| Fig. 3 (a) CV curves of the MnO2, 3D MnO2/SG, 3D Ag–MnO2/SG at a scan rate of 5.0 mV s−1; (b) CV curves of 3D Ag–MnO2/SG electrode over a range of scan rates from 5 to 100 mV s−1; (c) GCD curves of the MnO2, 3D MnO2/SG, 3D Ag–MnO2/SG; (d) GCD curves of 3D Ag–MnO2/SG electrode at current density various from 0.5 A g−1 to 10 A g−1; (e) specific capacitances of the three electrodes at different current density; (f) long-term cycling test by measuring 5000 charge/discharge cycles with a current density of 5 A g−1. | |
As shown in Fig. 3c, the capacitive performances of the electrodes were further investigated with galvanostatic charge/discharge (GCD) cycling experiments (at current density of 0.5 A g−1). It can be seen that the 3D Ag–MnO2/SG shows a smaller voltage IR drop than that of MnO2 and 3D MnO2/SG, indicated the high conductivity for 3D Ag–MnO2/SG. Moreover, the 3D Ag–MnO2/SG electrode exhibits the longest discharge time, indicating the best capacitive behaviour. In order to investigate the rate performance of the obtained materials, GCD experiments at different current densities were carried out. As shown in Fig. 3d, the GCD curves of the 3D Ag–MnO2/SG are nearly symmetric triangle, indicated the electrical double layer capacitive behaviour and good rate performance, consistent with the CV result shown in Fig. 3b. The specific capacitances at various current densities were calculated according to the GCD curves. As shown in Fig. 3e, at a low current density of 0.5 A g−1, the maximum specific capacitances for 3D Ag–MnO2/SG can reach 537.0 F g−1, higher than the 3D MnO2/SG (508.8 F g−1) and MnO2 (172.26 F g−1). In addition, when the current density increased from 0.5 to 10 A g−1, the capacitive retention is about 71.02% for 3D Ag–MnO2/SG, 20.64% for 3D MnO2/SG and 13.81% for MnO2.
Cycling performance of the MnO2, 3D MnO2/SG, 3D Ag–MnO2/SG were evaluated by measuring 5000 charge/discharge cycles with a constant current density of 5 A g−1 and the results are shown in Fig. 3f. After 5000 cycles, the retention of 98.29% for 3D MnO2/SG and 99.72% for 3D Ag–MnO2/SG were observed, much higher than that of pure MnO2 (77.36%). The high electrochemical stability mainly attributed to the 3D porous SG structure.
Compared with other reported MnO2/grapheme materials, the obtained 3D Ag–MnO2/SG also shows better performance, such as nanostructured graphene/amorphous MnO2 composites (367 F g−1 at a current density of 1 A g−1 and only 73.9% of the original capacitance were maintained after 1000 cycles),34 Sandwich-like RGO/MnO2 hybrid film (446 F g−1 at 5 mV s−1 and 96% of its original capacitance were retained after 1000 cycles),8 the low rate capability of RGO–MnO2 hollow sphere (136 F g−1 at current densities of 1.5 A g−1).35
According to the above discussions, we can make a conclusion that the superior performances of 3D Ag–MnO2/SG are attributed to the following reasons: (1) the interconnected 3D porous SG networks reduce the diffusion distance for the electrolyte and accumulating more electrolyte ions, improving the rate performance and capacity; (2) after decorating with Ag nanoparticles, the capacitive performance were enhanced because of the improved conductivity.
To study the relationship between the excellent electrochemical performance and 3D Ag–MnO2/SG nanocomposite, electrical impedance spectroscopy (EIS) measurement was carried out and the collected result is shown in Fig. 4. Each Nyquist plot consists of a small semicircle in high-frequency and a straight line in the low-frequency region. The smaller diameter of the semicircle corresponds to smaller charge transfer resistance at the electrode/electrolyte interface.5,12 From the Nyquist plots, we can infer that the 3D Ag–MnO2/SG shows the minimum charge transfer resistance (Rct). The smallest Rct is mainly due to its fast electrode kinetics, which enhanced by the Ag decoration and the 3D SG conductive framework.
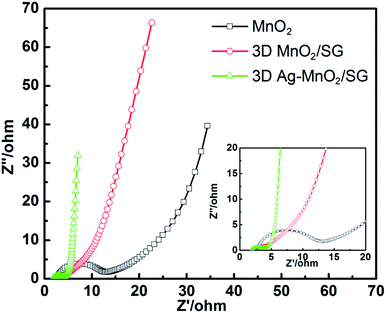 |
| Fig. 4 Electrochemical impedance spectra of the obtained electrodes (the inset is the expanded view for the high frequency range). | |
4. Conclusions
In this work, the synthesis of 3D Ag–MnO2/SG and its application in supercapacitor electrode was investigated. The 3D macroporous SG provides convenient channel for easy electrolyte penetration, which improved the rate performance of the electrode. In addition, the introduction of the silver nanoparticles not only improves electrical conductivity of the MnO2, but also prevents the SG sheets from accumulation. Due to the synergistic effect of Ag particles and 3D interpenetrating SG networks, the 3D Ag–MnO2/SG exhibits a maximum specific capacitance of 537.0 F g−1 at 0.5 A g−1 and excellent rate performance. Furthermore, the method delivered in our experiment could be applied in many other kinds of materials that the electrochemical performance needs to be improved.
Acknowledgements
FL acknowledges the support from the National Science Foundation of China (21371105, 51402162, and 51272116), the Scientific Development Plan of Qingdao (14-2-4-41-jch) and the college student innovation fund project of Qingdao Technological University (1409020302). WL acknowledges the support from National Science Fund for Distinguished Young Scholars (51525903), National 863 program (2015AA034404), and Marine science and technology projects of Huangdao district (2014-4-1).
Notes and references
- J. Zhang and J. W. Lee, ACS Sustainable Chem. Eng., 2014, 2, 735–740 CrossRef CAS.
- N. Md Disa, S. Abu Bakar, S. Alfarisa, A. Mohamed, I. Md Isa, A. Kamari, N. Hashim and M. Rusop Mahmood, Adv. Mater. Res., 2015, 1109, 40–44 CrossRef.
- P. Simon, Y. Gogotsi and B. Dunn, Science, 2014, 343, 1210–1211 CrossRef CAS PubMed.
- S. R. Sivakkumar, J. M. Ko, D. Y. Kim, B. C. Kim and G. G. Wallace, Electrochim. Acta, 2007, 52, 7377–7385 CrossRef CAS.
- M. Pang, G. Long, S. Jiang, Y. Ji, W. Han, B. Wang, X. Liu and Y. Xi, Electrochim. Acta, 2015, 161, 297–304 CrossRef CAS.
- X.-F. Lu, A.-L. Wang, H. Xu, X.-J. He, Y.-X. Tong and G.-R. Li, J. Mater. Chem. A, 2015, 3, 16560–16566 CAS.
- H. Li, L. Jiang, Q. Cheng, Y. He, V. Pavlinek, P. Saha and C. Li, Electrochim. Acta, 2015, 164, 252–259 CrossRef CAS.
- H. Zhou, X. Yang, J. Lv, Q. Dang, L. Kang, Z. Lei, Z. Yang, Z. Hao and Z.-H. Liu, Electrochim. Acta, 2015, 154, 300–307 CrossRef CAS.
- C. Choi, H. J. Sim, G. M. Spinks, X. Lepró, R. H. Baughman and S. J. Kim, Adv. Energy Mater., 2016 DOI:10.1002/aenm.201502119.
- Y. Dai, L. Chen, V. Babayan, Q. Cheng, P. Saha, H. Jiang and C. Li, J. Mater. Chem. A, 2015, 3, 21337–21342 CAS.
- J. Deng, L. Chen, Y. Sun, M. Ma and L. Fu, Carbon, 2015, 92, 177–184 CrossRef CAS.
- L. Li, R. Li, S. Gai, P. Gao, F. He, M. Zhang, Y. Chen and P. Yang, J. Mater. Chem. A, 2015, 3, 15642–15649 CAS.
- F. Y. Cheng, J. Chen, X. L. Gou and P. W. Shen, Adv. Mater., 2005, 17, 2753–2756 CrossRef CAS.
- M. Huang, F. Li, F. Dong, Y. X. Zhang and L. L. Zhang, J. Mater. Chem. A, 2015, 3, 21380–21423 CAS.
- P. Prabunathan, K. Sethuraman and M. Alagar, RSC Adv., 2014, 4, 47726–47734 RSC.
- K. Liang, T. Gu, Z. Cao, X. Tang, W. Hu and B. Wei, Nano Energy, 2014, 9, 245–251 CrossRef CAS.
- H. Xia, C. Hong, X. Shi, B. Li, G. Yuan, Q. Yao and J. Xie, J. Mater. Chem. A, 2015, 3, 1216–1221 CAS.
- F.-Q. Liu, J. Su, W. Wang, W.-H. Li, H.-Q. Hu, L. Wang and R.-X. Li, J. Mater. Chem. A, 2015, 3, 3136–3143 CAS.
- M. Chen, X. Xia, J. Yuan, J. Yin and Q. Chen, J. Power Sources, 2015, 288, 145–149 CrossRef CAS.
- S. H. Choi, Y. N. Ko, J.-K. Lee and Y. C. Kang, Adv. Funct. Mater., 2015, 25, 1780–1788 CrossRef CAS.
- Z. Zhang, F. Xiao, L. Qian, J. Xiao, S. Wang and Y. Liu, Adv. Energy Mater., 2014, 4, 1–10 Search PubMed.
- A. Liu, C. Li, H. Bai and G. Shi, J. Phys. Chem. C, 2010, 114, 22783–22789 CAS.
- G. Wang, Q. Tang, H. Bao, X. Li and G. Wang, J. Power Sources, 2013, 241, 231–238 CrossRef CAS.
- C. Bora, J. Sharma and S. Dolui, J. Phys. Chem. C, 2014, 118, 29688–29694 CAS.
- C. Xu, B. Li, H. Du, F. Kang and Y. Zeng, J. Power Sources, 2008, 184, 691–694 CrossRef CAS.
- G. Zhang, L. Zheng, M. Zhang, S. Guo, Z.-H. Liu, Z. Yang and Z. Wang, Energy Fuels, 2012, 26, 618–623 CrossRef CAS.
- L. Ma, X. Shen, Z. Ji, G. Zhu and H. Zhou, Chem. Eng. J., 2014, 252, 95–103 CrossRef CAS.
- Z. Qiao, X. Yang, S. Yang, L. Zhang and B. Cao, Chem. Commun., 2016, 52, 7998–8001 RSC.
- H. Jiang, C. Li, T. Sun and J. Ma, Chem. Commun., 2012, 48, 2606–2608 RSC.
- J. L. Shi, C. Tang, H. J. Peng, L. Zhu, X. B. Cheng, J. Q. Huang, W. Zhu and Q. Zhang, Small, 2015, 11, 5243–5252 CrossRef CAS PubMed.
- J. Hao, Y. Liao, Y. Zhong, D. Shu, C. He, S. Guo, Y. Huang, J. Zhong and L. Hu, Carbon, 2015, 94, 879–887 CrossRef CAS.
- Y. Huang, J. Liang and Y. Chen, Small, 2012, 8, 1805–1834 CrossRef CAS PubMed.
- H. Jiang, P. S. Lee and C. Li, Energy Environ. Sci., 2013, 6, 41–53 CAS.
- M. Pang, G. Long, S. Jiang, Y. Ji, W. Han, B. Wang, X. Liu and Y. Xi, Mater. Sci. Eng., B, 2015, 194, 41–47 CrossRef CAS.
- H. Chen, S. Zhou, M. Chen and L. Wu, J. Mater. Chem., 2012, 22, 25207 RSC.
Footnote |
† Electronic supplementary information (ESI) available: SEM and TEM images of the obtained 3D SG nanostructures and 3D MnO2/SG; the EDS elemental mapping of C, S, Mn, O and Ag for the 3D Ag–MnO2/SG. See DOI: 10.1039/c6ra19431a |
|
This journal is © The Royal Society of Chemistry 2016 |