DOI:
10.1039/C6RA19152E
(Paper)
RSC Adv., 2016,
6, 100984-100992
Photodynamic therapy of drug-resistant human colon adenocarcinoma using verteporfin-loaded TPGS nanoparticles with tumor homing and penetrating peptide functionalization†
Received
28th July 2016
, Accepted 3rd October 2016
First published on 5th October 2016
Abstract
Photodynamic therapy (PDT) does well in both curing early stage tumors and improving patients’ quality of life. However, the limited selectivity of photosensitizers (PSs) to tumors has become an urgent issue during the application of PDT clinically, which would exhibit a poor therapeutic outcome and induce skin phototoxicity. To solve this problem, PS-loaded D-α-tocopheryl polyethylene glycol succinate (vitamin E TPGS, or simply TPGS) nanoparticles with tumor homing and penetrating peptide tLyp-1 decoration (t-NP) were constructed for PDT of tumors in this study. Verteporfin (VP), as an effective PS, was encapsulated in the core of t-NP, and tLyp-1 was functionalized on the surface as a functional ligand to increase vascular permeability and target the tumor cells. These nanoparticles were spherical in shape and exhibited a narrow size distribution of 23.3 ± 0.80 nm, and had a high encapsulation efficiency and loading capacity of hydrophobic VP. Compared with unmodified nanoparticles (NP), t-NP exhibited enhanced cellular uptake in both primary human umbilical vein endothelial cells (HUVECs) and drug-resistant human colon adenocarcinoma cells (HCT15); and t-NP showed higher selectivity to HCT15 tumors in vivo after intravenous administration of the same dose of VP. The anti-tumor experiment in vivo strongly indicated that nanoparticles with tLyp-1 peptide modification can enhance the selectivity of the PS, and improve the PDT effect.
Introduction
Cancer attracts more and more attention as one of the deadly diseases of the world, and billions of dollars have been spent to investigate how to cure this fatal disease and improve patients’ quality of life very year.1 The traditional treatment of cancer, that is, surgery, chemotherapy, and radiation therapy, usually results in serious side effects, such as the loss of normal organ function, systemic toxicity or multi-drug resistance (MDR). Currently, PDT has attracted great attention for its minimally invasive therapeutic procedure during cancer treatment, and it can be used for inoperable tumors or on patients who are unable to withstand traditional cancer treatments.1–3 As a clinically approved therapeutic modality, PDT can be applied in the treatment of early-stage tumors or as a valuable therapeutic option for combination treatments.4–7 During PDT treatment, light of a corresponding wavelength is used to trigger the PS, and when the PS returns to its ground state the tissue oxygen is transformed into singlet oxygen (1O2) which is cytotoxic and believed to induce apoptotic cell death.8–10 Therefore, the PS plays an important role in the PDT procedure, but the limited selectivity and high hydrophobicity of the PS have been a bottleneck of clinical PDT.11–13 So it is imperative to enhance the targeting effect of PS.
Verteporfin (benzoporphyrin derivative monoacid ring A, BPD-MA, or simply VP) is a potent photosensitizing agent, and is approved as a PS for the treatment of age-related macular degeneration (AMD) by the FDA. Because of its high 1O2 yield and high absorption peak at 690 nm, VP is widely used to treat cancer of the eyes, pancreas, and skin, or is utilized for the diagnosis of cancer (fluorescence diagnosis, FD) as well.14–16 However, like most of the commonly used PSs at present,17,18 VP is such a highly hydrophobic compound that it barely dissolves in injections directly.
TPGS is a feasible choice to form the architecture of nanoparticles to make VP well dispersed in the aqueous phase. TPGS is non-ionic and consists of a lipophilic alkyl tail and a hydrophilic polar head, so it is appropriate to form nanoparticles for encapsulation of hydrophobic drugs; in addition, hydroxyl-terminated TPGS can be modified for ligand conjugation.19–22 Furthermore, it also has the ability to prevent loaded drugs from being excreted by P-glycoprotein (P-gp), an efflux transporter of diverse lipophilic, neutral, or cationic compounds.23–25 There are also another two advantages of using TPGS to create drug-loading nanoparticles. First is P-gp inhibition: TPGS nanoparticles can prevent VP from being pumped out of the tumor cells.26,27 Second is the enhanced permeability and retention effect (EPR effect): once injected intravenously, the TPGS nanoparticles will selectively egress at leaky tumor vasculatures and remain in the tumor interstitium for an extended period of time.28–30
Nevertheless, preparation which only has a passive targeting mechanism contains some limitations; the chief problem is that drug delivery, which depends on the EPR effect, may be hampered by heterogeneity in different tumors and experimental data from patients on accumulation effectiveness of this mechanism are limited.12,31 Thus, compared with the simple EPR effect, active targeting resulting from targeting ligands is considered to be more effective.32 Recent reports have suggested that peptides containing a C-terminal arginine with a free carboxyl group (CendR) can be a ligand for neuropilin-1 (NRP-1), and responsible for cell internalization and tissue penetration activity.33,34 NRP-1 is a 120–130 kDa single spanning transmembrane glycoprotein, and has an important role in promoting cell proliferation in tumors and migrating over the body as a receptor of vascular endothelial growth factor.35–37 It has been demonstrated that NRP-1 is over-expressed in multiple tumors and tumor vasculature,38,39 like human colon adenocarcinoma cells40 and endothelial cells of angiogenic blood vessels.41 With the exposed CendR motif R/KXXR/K, the tLyp-1 peptide which is sequenced by CGNKRTR can interact with NRP-1.42,43 This implies that the tLyp-1 peptide can be used as an effective targeting ligand for tumor homing, penetrating from the blood vessels into the tumor parenchyma.44,45
In this work, tLyp-1 peptide-decorated TPGS nanoparticles were developed as the verteporfin (VP) carrier, which could actively transport VP to the tumor site, enhance the uptake of cells and could not be removed by P-gp exocytosis, (Fig. 1). The study was conducted via comparison of the in vitro and in vivo therapeutic effects between the unmodified TPGS nanoparticles loaded with VP (NP) and the tLyp-1-modified TPGS nanoparticles loaded with VP (t-NP) on the HUVEC and HCT15 cells. HCT15 cells are drug-resistant cancer cells with over-expressed P-gp.46–52 While enhancing the targeting ability of the nanoparticles, the treatment efficacy of PDT can be safer, more selective and effective. In addition, compared with previous work,18,53 the delivery system proposed in this paper has high hydrophobic drug encapsulation efficiency and drug loading capacity, while its preparation process was more convenient and rapid.
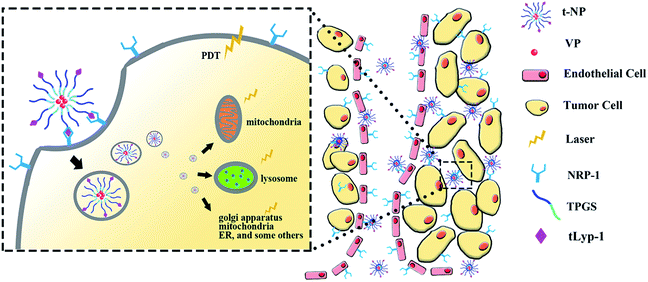 |
| Fig. 1 tLyp-1-modified nanoparticles loaded with VP (t-NP) as a dual-targeting drug delivery system via NRP-1-mediated endocytosis and penetration. | |
Results and discussion
Preparation and characterization of nanoparticles
The 1H NMR spectra indicated that the sulfhydryl of tLyp-1 had reacted with the alkenyl of TPGS-MAL, and the efficiency of ligation is about 63% (Fig. S2†).
The nanoparticles were prepared via the solvent casting method.19 The size, polydispersity index (PI) and zeta potential measured by DLS are shown in Table 1. It was found that the mean sizes of NP and t-NP were 22.4 ± 0.71 nm and 23.3 ± 0.80 nm (Table 1 and Fig. 2A and B), respectively, with a narrow distribution (PI 0.219 ± 0.05 and 0.262 ± 0.07, respectively). It could also be seen that the tLyp-1 conjugation had little impact on particle size. This result could also be observed from the TEM images, and the VP-loaded nanoparticles were spherical in shape as shown in Fig. 2C and D. The sizes of NP and t-NP were regarded as suitable for the EPR effect.
Table 1 Characterization of NP and t-NP with 1 wt% VP loadeda
Nanoparticle |
Particle size (nm) |
Polydispersity index (PI) |
Zeta potential (mV) |
Encapsulation efficiency (%) |
Data represented as mean ± SD (n = 3). |
NP |
22.4 ± 0.71 |
0.219 ± 0.05 |
−6.02 ± 0.48 |
99.15 ± 0.62 |
t-NP |
23.3 ± 0.80 |
0.262 ± 0.07 |
−3.40 ± 0.72 |
99.26 ± 0.43 |
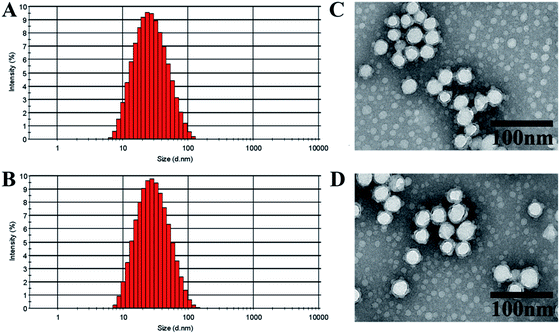 |
| Fig. 2 Characterization of NP and t-NP. The particle size distribution and TEM images of NP (A and C) and t-NP (B and D). | |
A negative surface charge was detected for NP (−6.02 ± 0.48 mV), which was only slightly changed following the peptide conjugation (−3.40 ± 0.72 mV) for t-NP. It might have been caused by the positively charged tLyp-1. The encapsulation efficiency of NP and t-NP was 99.15 ± 0.62% and 99.26 ± 0.43%, respectively. The TPGS nanoparticles showed a high hydrophobic drug (VP) encapsulation efficiency and high nanoparticle stability.
The actual drug loading of MAX-NP and MAX-t-NP was measured, and the loading capacity of MAX-NP and MAX-t-NP was 5.91% and 5.78%, respectively; that is, the maximum drug loading capacity of VP-loaded NP and t-NP was 5.91% and 5.78%, respectively.
In vitro experiments
Cellular association. Coumarin-6-loaded NP and t-NP were used to investigate the cell internalization of NP and t-NP. The cells were incubated with 600 ng mL−1 coumarin-6-labelled NP and t-NP for 0.5, 1, and 2 h. Comparing the incubation results at 0.5, 1 and 2 h (Fig. S4†), the uptake of 1 h incubation was significantly higher than that of 0.5 h, and the uptake of 2 h incubation was similar to that of 1 h. It could be observed that HCT15 and HUVEC cells took 1 h to reach the maximum uptake of NP and t-NP.Qualitative fluorescence images that were taken with fluorescent microscopy showed that following 1 h exposure to coumarin-6-loaded NP and t-NP, HCT15 cells treated with t-NP exhibited a higher level of internalization than those treated with NP at the same coumarin-6 concentration. The situation is similar in HUVEC cells, and the fluorescence signals in HUVEC cells treated with t-NP were stronger than those treated with NP. The cellular associated fluorescence intensity in both HCT15 and HUVEC cells increased with the increase of the concentration (Fig. 3A and B). Consistent with the qualitative analysis, quantitative analysis displayed the advantage of t-NP in uptake and the same concentration-dependent cell internalization behaviour in both HCT15 and HUVEC cells (Fig. 3C and D). It was found that the uptake of t-NP was significantly higher than that of undecorated NP in both cells. With the coumarin-6 concentrations varied from 200 ng mL−1 to 1000 ng mL−1, the fluorescence signals subsequently increased in both cells. Compared with that of undecorated NP, the fluorescence intensity of t-NP was 1.54-fold higher in HCT15 cells and 1.65-fold higher in HUVEC cells at a coumarin-6 concentration of 1000 ng mL−1.
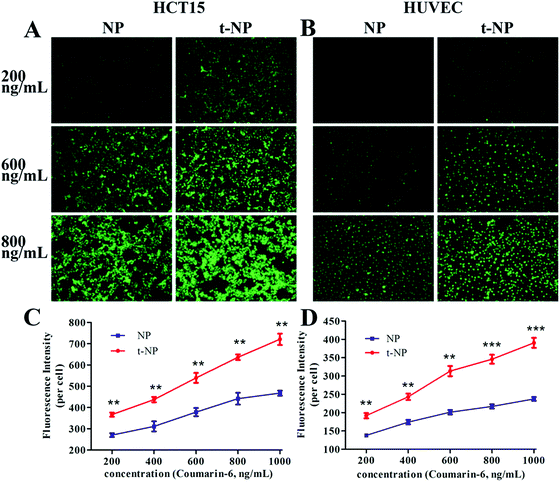 |
| Fig. 3 Fluorescence images of NP and t-NP with coumarin-6 as the fluorescent probe at a concentration of 200 ng mL−1, 600 ng mL−1, and 800 ng mL−1 at 37 °C in HCT15 cells (A) and HUVEC cells (B) after 1 h incubation. Original magnification: ×20. Cellular association of coumarin-6-labelled NP and t-NP at a concentration from 200 ng mL−1 to 1000 ng mL−1 for 1 h at 37 °C in HCT15 cells (C) and HUVEC cells (D). The data are represented as the mean ± SD (n = 3). **p < 0.01, ***p < 0.001, significantly higher than the cellular uptake of unmodified NP at 37 °C. | |
The high cellular uptake of t-NP might be attributed to the P-gp inhibition produced by TPGS and the interaction between peptide tLyp-1 and NRP-1. The tLyp-1-decorated nanoparticles were easily taken in by HCT15 cells and HUVEC cells which over-expressed NRP-1. It was hard to pump the t-NP out through P-gp exocytosis, once the target cells took in the nanoparticles.
Colocalization assay. As shown in Fig. 4A, after incubating HCT15 cells with VP-loaded t-NP for 1 h, the intracellular fluorescent signals of VP (blue) were mostly colocalized with lysosomes (red), which demonstrated that the lysosomes were exactly involved in the cellular uptake of t-NP. On the other hand from Fig. 4B, it can be clearly observed that the lysosomes of cells applied with a laser were damaged, and the fluorescent signal of VP was decreased.
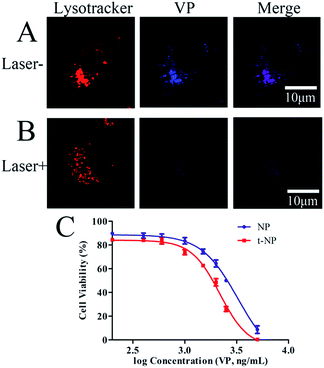 |
| Fig. 4 Colocalization assay of t-NP in HCT15 cells without laser (A) and with laser (B). Lysotracker (Ex/Em, 577/570–590 nm) (red), VP (Ex/Em, 350/440–460 nm) (blue). (C) Cell viability of HCT15 cells after treatment with VP-loaded NP and VP-loaded t-NP with an irradiance of 0.3 J cm−2. | |
The colocalization assay above could demonstrate that t-NP was localized in the lysosome, and when irradiated the membranous structure would be broken by reactive oxygen species (ROS) which were generated in the presence of the photosensitizer VP. It is widely accepted that lysosomal destruction and the released lysosomal cathepsins would cause cell death.54,55
In vitro cytotoxicity. Many experiments confirmed no cytotoxicity of irradiation only or TPGS only in MTT assay even with a larger amount of TPGS.56,57 Four control groups (no operation, irradiation only, TPGS only, and TPGS with irradiation) were tested, and the results are shown in Fig. S5.† There seemed to be no significant difference between groups treated with irradiation only, TPGS only, TPGS with irradiation, and no operation. The MTT assay was used to evaluate the anti-proliferative effect of VP-loaded NP and t-NP with laser irradiation. In Fig. 4C, it is revealed that the t-NP exhibited a higher anti-proliferative ability than the unmodified NP. The IC50 values of t-NP and NP were 2.17 μg mL−1 and 3.25 μg mL−1, respectively.With the advantages mentioned above, it seemed that the accumulation ability of t-NP was better than that of NP in cells, therefore it showed a superior anti-proliferative ability.
In vivo experiments
In vivo real-time imaging. To evaluate whether the peptide-modified nanoparticles could indeed specifically target tumors, the real-time distribution of NP and t-NP was studied in nude mice bearing a subcutaneous tumor using VP as the probe for imaging. VP is an effective theranostic agent and is often used in clinical applications for imaging and therapy.16,58 In Fig. 5A and B, it is observed that t-NP exhibited a higher fluorescence intensity in the tumor. This result was confirmed by imaging those excised organs and tumors from treated mice after 24 h administration (Fig. 5C), and the bright field image of the excised organs and tumors is shown in Fig. S3.† Semi-quantitative analysis is shown in Fig. 5D: compared with the isolated tumors with NP, those with t-NP showed a markedly increased fluorescence intensity. Under the same VP dose and EPR effect, the targeting effect of tLyp-1 might result in the outstanding accumulation of t-NP.
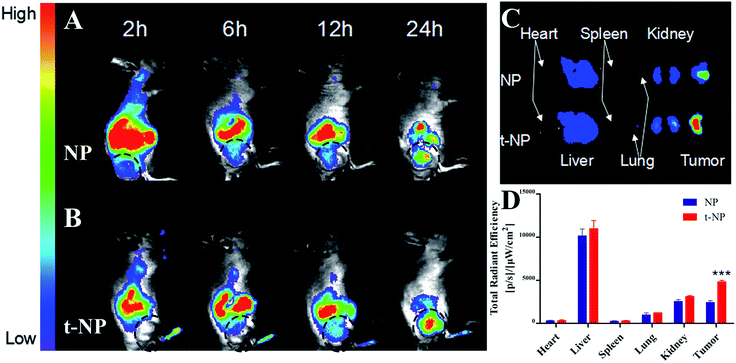 |
| Fig. 5 In vivo near-infrared imaging of mice bearing subcutaneous HCT15 cells intravenously treated with VP-loaded NP (A) and VP-loaded t-NP (B) at the time points of 2 h, 6 h, 12 h, and 24 h. (C) Fluorescent signals of NP and t-NP were detected in the organs and tumors 24 h post-administration. (D) Semi-quantitative analysis of the total radiant efficiency from the formulations in organs and tumors. The black circles indicate the tumor site. The data represent the mean ± SD (n = 6). ***p < 0.001, significantly different with that of the NP group. | |
In vivo tumor distribution. CLSM analysis was conducted to evaluate the abilities of penetration and accumulation of NP and t-NP in tumors. As shown in Fig. 6A, lots of coumarin-6-labelled NP (green) was entrapped in the blood vessels (red), while it was rarely accumulated in tumor tissue. In Fig. 6B, it is seen that a considerable amount of coumarin-6-labelled t-NP extravasated from the blood vessels and accumulated in the tumor tissue. This finding might have resulted because of the tLyp-1 peptide which could heighten vascular extravasation and deep penetration into the tumor tissue.44,45
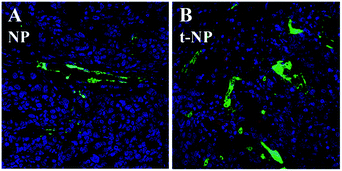 |
| Fig. 6 In vivo tumor distribution of coumarin-6-labelled NP (A) and coumarin-6-labelled t-NP (B) 3 h post-administration. Blue: DAPI stained cell nuclei. Red: CD31 stained tumor angiogenesis. Green: coumarin-6-labelled nanoparticles. Original magnification: ×20. | |
Anti-tumor effect. For the evaluation of in vivo anti-tumor activity, VP-loaded NP and t-NP were injected into tumor-bearing mice, and PBS served as the control. As shown in Fig. 7A and C, no inhibition was detected following the PBS-treated control with the laser irradiation. NP and t-NP could inhibit tumor growth to different degrees, between which t-NP with the laser had a better therapeutic effect. In the NP group, the tumors began to swell post-treatment, and then returned to the original size or decreased slightly in day 2. In the t-NP group, the tumors swelled as those in the NP group did, and then began to decrease or even disappear in day 2. As a result, the tumors of the t-NP group were significantly smaller than those of the PBS control group and NP group in day 14. The weight change of the mice in treatment is shown in Fig. 7B. It seemed that there was no significant change in either group. Although the mice in the NP and the t-NP groups lost some weight, they recovered quickly.
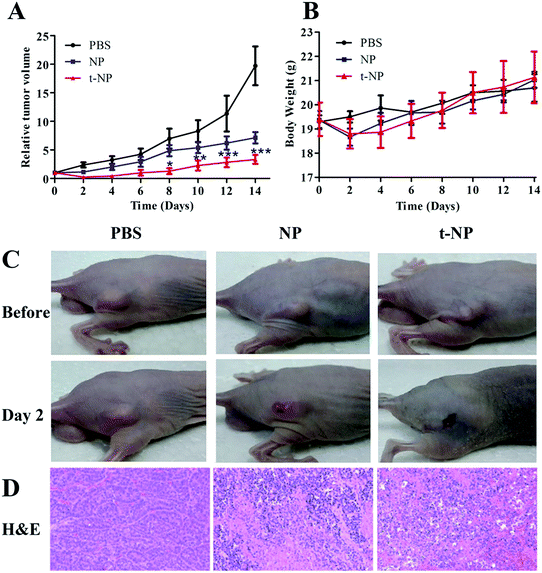 |
| Fig. 7 Anti-tumor efficiency of t-NP on nude mice bearing HCT15 tumors. Tumor growth curves (A), body weight change (B), and visible images (C) of mice following one treatment of PBS. NP and t-NP (5 mg kg−1 of VP) were applied intravenously by tail. 24 h later, the tumors were irradiated with a total light dose of 102 J cm−2. (D) Hematoxylin-eosin-stained tumors in the mice treated with formulations. The animals treated with PBS were used as the control. Original magnification: ×100. *p < 0.05, **p < 0.01, ***p < 0.001, significantly smaller than the tumor volume of the control group. | |
To confirm the targeting cytotoxicity effect, histology analysis with H&E was performed at 30 min after irradiation. As shown in Fig. 7D, numerous apoptosis and necrosis existed in the tumors of the t-NP group, more than that in the tumors of the NP group.
Experimental
Materials
Coumarin-6, Hoechst 33258, vitamin E TPGS (D-α-tocopheryl polyethylene glycol 1000 succinate), and 3-[4,5-dimethylthiazol-2-yl]-2,5-diphenyl tetrazolium bromide (MTT) were all purchased from Sigma-Aldrich (St. Louis, MO, USA). DAPI (4,6-diamidino-2-phenylindole) was provided by Molecular Probes (Eugene, OR, USA). The tLyp-1 peptide (sequence: CGNKRTR) was provided by ChinaPeptides Co. Ltd. (Shanghai, China). Alexa Fluor 594 anti-mouse CD31 antibody was obtained from BioLegend (San Diego, CA). 6-Maleimidocaproic acid, N-hydroxybenzotrizole (HOBT) and dicyclohexylcarbodiimide (DCC) were obtained from Aladdin Co., Ltd. (Shanghai, China). Verteporfin (VP) was provided by Shanghai Xianhui Pharmaceutical Co. Ltd. (Shanghai, China). All other chemicals were purchased from Sinopharm Chemical Reagent Co., Ltd. (Shanghai, China) and used as received unless otherwise mentioned. Fetal bovine serum (FBS), DMEM, (RPMI) 1640 medium, trypsin-EDTA, and penicillin–streptomycin were supplied by Gibco BRL (Carlsbad, CA, USA).
Cell lines and animals
HUVEC cells and HCT15 cells were obtained from Cascade Biologics (Portland, OR, USA). The HUVEC and HCT15 cells were routinely incubated in DMEM and (RPMI) 1640, respectively, and supplemented with penicillin–streptomycin and 10% FBS at 37 °C, 5% CO2, and 95% humidity.
Animal experimentation was performed according to the guidelines evaluated and approved by the Animal Experimentation Ethics Committee of Fudan University (Shanghai, China). Balb/c nude mice (male, 6 weeks, 20 ± 2 g) were provided by BK Lab Animal Ltd. (Shanghai, China) and housed by Department of Experimental Animals of Fudan University (Shanghai, China) at 25 ± 1 °C with access to food and water ad libitum.
Preparation of nanoparticles
The TPGS-tLyp-1 was prepared and characterized, and the synthesis procedure is mentioned in the ESI.† Firstly, in order to obtain TPGS-MAL with exposed maleimide, we made the 6-maleimidocaproic acid react with TPGS through an esterification process. Secondly, the tLyp-1 peptide was reacted with TPGS-MAL via an addition reaction. The 1H NMR spectra of TPGS-MAL and TPGS-tLyp-1 are shown in Fig. S1 and S2.† Undecorated nanoparticles with VP (NP) were prepared via the solvent casting method. The VP (500 μg) or coumarin-6 (500 μg) was mixed with TPGS (50 mg) in chloroform (3 mL). The solution was evaporated to remove the organic solvent in a bath at 40 °C, using a round-bottom flask. After formation of a film, the drug-dispersed TPGS was evaporated for another 10 min, and then hydrated in 0.01 M phosphate buffered saline (PBS, pH 7.4). The mixture was stirred constantly at 37 °C for 12 h. The excess amount of VP or coumarin-6 was removed through a 0.22 μm filter before characterization. Similarly, the tLyp-1-functionalized nanoparticles (t-NP) were prepared following the same procedure described above, except that 10 wt% of TPGS was replaced by TPGS-tLyp-1. NP and t-NP were used to do the following experiment.
In addition, the maximum drug loading capacity of VP-loaded NP and t-NP was determined by excess input of VP; 5 mg VP was mixed with 50 mg TPGS (or 45 mg TPGS and 5 mg TPGS-tLyp-1) to form the maximum VP loading NP (or t-NP) (referred to as simply MAX-NP (or MAX-t-NP)).
Nanoparticle characterization
Particle size and surface charge. The size, size distribution, and zeta potential of NP and t-NP were measured by a dynamic light scattering (DLS) detector (Zetasizer, Nano-ZS, Malvern, UK).The shape and surface morphology of the nanoparticles were studied by transmission electron microscopy (TEM) (H-600, Hitachi, Tokyo, Japan), negatively stained with 1% phosphotungstic acid.
Drug loading capacity and encapsulation efficiency. The amount of VP encapsulated in NP and t-NP was measured by a fluorescence spectrophotometer (Ex/Em, 441 nm/690 nm). The loading capacity (LC) and encapsulation efficiency (EE) were calculated as follows (eqn (1) and (2)):59 |
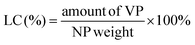 | (1) |
|
 | (2) |
In vitro experiments
Cellular association. Because the excitation wavelength for Hoechst 33258 (Ex/Em, 352 nm/461 nm) (blue) and DAPI (Ex/Em, 364 nm/454 nm) (blue) can excite VP as well, coumarin-6 was used as the fluorescent probe instead of VP in the experiment of cellular association and in vivo tumor distribution. HUVEC and HCT15 cells were counted and seeded at a density of 5 × 103 cells per well in 96-well plates overnight. The medium was replaced by coumarin-6-loaded NP and t-NP with a coumarin-6 concentration of 200–1000 ng mL−1, and the cells were incubated for 1 h. After the incubation, the cells were washed twice with PBS (pH 7.4), and fixed with 4% formaldehyde for 15 min. The nuclei were stained with 2 μg mL−1 Hoechst 33258 at room temperature for 10 min. Then the cells were detected by quantitative analysis under a KineticScan® HCS Reader (version 3.1, Cellomics Inc., Pittsburgh, PA, USA), and were subjected to observation for qualitative analysis under a fluorescence microscope (Leica DMI 4000B, Germany).For the pattern studies of absorption time, the cells were incubated with 600 ng mL−1 coumarin-6-labelled NP and t-NP for 0.5, 1, and 2 h. After the incubation, the cells were washed twice with PBS (pH 7.4), and fixed with 4% formaldehyde for 15 min. The nuclei were stained with 2 μg mL−1 Hoechst 33258 at room temperature for 10 min. Then the cells were detected by quantitative analysis under a KineticScan® HCS Reader.
Colocalization assay. HCT15 cells were seeded in a confocal dish at a density of 5 × 104 cells per well. After being cultured for 24 h, the cells were exposed to 1000 ng mL−1 VP-loaded t-NP for 1 h and then rinsed in the fresh medium and incubated with 50 nmol L−1 Lysotracker red for 30 min. After being stained, the cells were fixed with 4% paraformaldehyde, then examined by fluorescence with a confocal microscope (Carl Zeiss LSM710, Germany). Lysotracker red was excited at 577 nm, and emitted at 570–590 nm (red); as mentioned, VP could be excited at about 350 nm, while it emitted at 440–460 nm (blue). For the investigation of PDT, HCT-15 cells were applied with an irradiance of 0.3 J cm−2 after being exposed to 1000 ng mL−1 VP-loaded t-NP for 1 h. Thereafter, 50 nmol L−1 LysoTracker red was introduced followed by the processing as above, and the results were analyzed via a confocal microscope.
In vitro cytotoxicity. The cytotoxicity of NP and t-NP to HCT15 cells was compared using the MTT assay. HCT15 cells were seeded at a density of 5 × 103 cells per well in 96-well plates and incubated overnight to allow cell attachment. Those cells were randomly divided into six groups: two experimental groups (NP and t-NP with irradiation) and four control groups (no operation, irradiation only, TPGS only, and TPGS with irradiation). Subsequently, the medium was substituted by VP-loaded NP and t-NP with a VP concentration of 200–5000 ng mL−1 in the experimental groups. In the group of irradiation only, the medium was substituted by the fresh medium, and in the TPGS only and TPGS with irradiation groups the medium was substituted by the prepared medium with 50 μg mL−1 TPGS (equal parts of TPGS of NP and t-NP with a VP concentration of 5000 ng mL−1). After 1 h incubation protected from light, the wells were washed with PBS and fresh medium was added. The cells, except for in the TPGS only groups (protected from light), were applied with an irradiance of 0.3 J cm−2 (7 mW cm−2 for 0.75 min). And all cells were incubated for another 24 h. A 660 nm fiber coupled laser system (ADR1860, Feimiao Scientific Co., Changchun, China) was used as the laser source. Cell viability was determined by MTT assay standard protocol. A microplate reader (Thermo Multiskan MK3, USA) was used to examine the plates at a wavelength of 570 nm.
In vivo experiments
In vivo real-time imaging. HCT15 cells (2 × 106 cells per 100 μL) were inoculated into the 6-week old nude mice in the subcutaneous tissue of the right hindlimb. After two weeks, the nude mice bearing spherical tumors were randomly divided into two groups (n = 6) and injected through the tail vein with VP-loaded NP and t-NP (5 mg kg−1 of VP), respectively. As a photosensitizer, VP could be used in imaging to monitor the distribution of nanoparticles.16,58 The fluorescence images of the mice were acquired at 2, 6, 12 and 24 h after injection using an in vivo IVIS spectrum imaging system (Perkin Elmer, USA). When the in vivo imaging was finished, the hearts, livers, spleens, lungs, kidneys and tumors were collected for ex vivo imaging.
In vivo tumor distribution. Mice bearing subcutaneous HCT15 tumors were injected intravenously with coumarin-6-loaded NP and t-NP at a coumarin-6 dose of 5 mg kg−1. Three hours after the administration, two groups of mice were sacrificed, and heart perfusion was performed with 40 mL saline and 20 mL 4% paraformaldehyde. Afterward, tumors were taken out, fixed in 4% paraformaldehyde for 48 h, and dehydrated with 15% sucrose solution until subsidence (6 h), then in 30% sucrose solution until subsidence (24 h). The tumors were then frozen in OCT (Sakura, Torrance, CA, USA) at −20 °C overnight, and the 14 μm-thick slices were prepared with a freezing microtome (CM3050S, Leica, Germany). Before being exacmined under a confocal microscope (Carl Zeiss LSM710, Germany), the nuclei of the slices were stained with DAPI, and tumor angiogenesis reacted with the CD31 antibody.
Anti-tumor effect. 6-week old male nude mice were injected with HCT15 cells subcutaneously, and randomly divided into three groups (n = 6). When the tumors grew up to about 50 mm3, the three groups were treated with PBS, NP, and t-NP (5 mg kg−1 of VP) by a tail intravenous injection, respectively. 24 h later, the tumors of all those mice were irradiated for 10 min with a total light dose of 102 J cm−2 at a fluence rate of 170 mW cm−2. The tumor size was observed for 14 days to reveal the anti-tumor effect. The volume of the tumor was calculated using eqn (3). The relative tumor volume was defined as the ratio of the volume at a given time to the volume on day 0. The weight of the mice was also monitored. |
Tumor volume = 0.5 × length × (width)2
| (3) |
Histology examination was conducted on the three groups of nude mice bearing subcutaneous HCT15 tumors (n = 6). The groups were treated with PBS, NP, and t-NP (5 mg kg−1 of VP). Tumors were harvested 30 min after treatment. The mice were sacrificed, and heart perfusion was performed with 40 mL saline and 20 mL 4% paraformaldehyde. Then the tumor tissues were separated and fixed with 4% paraformaldehyde for paraffin section. The sliced tissues were stained with hematoxylin–eosin (H&E) and examined under a polarizing microscope (Nikon Eclipse 50i, H550S, and Japan).
Statistical analysis
Quantitative data were presented as the mean ± standard deviation. Analysis of variance (ANOVA) and the Student’s t test were used to determine the statistically significant difference. p values < 0.05 were defined as statistically significant.
Conclusions
In this study, we have presented a kind of targeting delivery system of VP-loaded TPGS nanoparticles with tLyp-1 peptide conjugation (t-NP) for effective and targeted PDT. The acquired t-NP was observed to be spherical in shape with a diameter of 23.3 ± 0.80 nm. The maximum drug loading capacity of t-NP could reach 5.78%, and the drug encapsulation efficiency was approximately 99%. t-NP could enhance cellular uptake of VP in both tumor cells and tumor vasculature cells, and improve the cytotoxicity in tumor cells in the presence of a laser. Further results of in vivo experiments showed that tLyp-1 could improve the targeting efficiency and penetration of nanoparticles into tumor sites. With efficient extravasation caused by tLyp-1, t-NP was an effective therapeutic agent to kill cancer cells through PDT, as proved in the experiment of in vivo anti-tumor activity. With the high selectivity of t-NP, the side effects like skin sensitivity or phototoxic response can be avoided.
Acknowledgements
This work was supported by National Natural Science Foundation of China (81373353) and grants from Shanghai Science and Technology Committee (13NM1400500).
References
- M. Ethirajan, Y. Chen, P. Joshi and R. K. Pandey, Chem. Soc. Rev., 2011, 40, 340–362 RSC.
- P. Agostinis, K. Berg, K. A. Cengel, T. H. Foster, A. W. Girotti, S. O. Gollnick, S. M. Hahn, M. R. Hamblin, A. Juzeniene, D. Kessel, M. Korbelik, J. Moan, P. Mroz, D. Nowis, J. Piette, B. C. Wilson and J. Golab, Ca-Cancer J. Clin., 2011, 61, 250–281 CrossRef PubMed.
- S. B. Brown, E. A. Brown and I. Walker, Lancet Oncol., 2004, 5, 497–508 CrossRef CAS PubMed.
- L.-J. Zhang, J. Bian, L.-L. Bao, H.-F. Chen, Y.-J. Yan, L. Wang and Z.-L. Chen, J. Cancer Res. Clin. Oncol., 2014, 140, 1527–1536 CrossRef CAS PubMed.
- L.-J. Zhang, D. O’Shea, C.-Y. Zhang, Y.-J. Yan, L. Wang and Z.-L. Chen, J. Cancer Res. Clin. Oncol., 2015, 141, 1921–1930 CrossRef CAS PubMed.
- L.-J. Zhang, L.-X. Wang, W.-L. Zhang, Y.-J. Yan and Z.-L. Chen, J. Innovative Opt. Health Sci., 2015, 8, 1540003–1540013 CrossRef CAS.
- J. Chen, L. Mao, S. Liu, Y. Liang, S. Wang, Y. Wang, Q. Zhao, X. Zhang, Y. Che, L. Gao and T. Liu, J. Photochem. Photobiol., B, 2015, 151, 186–193 CrossRef CAS PubMed.
- X. Ding and B.-H. Han, Angew. Chem., Int. Ed., 2015, 54, 6536–6539 CrossRef CAS PubMed.
- J. F. Lovell, T. W. B. Liu, J. Chen and G. Zheng, Chem. Rev., 2010, 110, 2839–2857 CrossRef CAS PubMed.
- C. S. Jin, L. Cui, F. Wang, J. Chen and G. Zheng, Adv. Healthcare Mater., 2014, 3, 1240–1249 CrossRef CAS PubMed.
- D. Hu, Z. Sheng, G. Gao, F. Siu, C. Liu, Q. Wan, P. Gong, H. Zheng, Y. Ma and L. Cai, Biomaterials, 2016, 93, 10–19 CrossRef CAS PubMed.
- Z. Zhen, W. Tang, Y.-J. Chuang, T. Todd, W. Zhang, X. Lin, G. Niu, G. Liu, L. Wang, Z. Pan, X. Chen and J. Xie, ACS Nano, 2014, 8, 6004–6013 CrossRef CAS PubMed.
- J. P. Celli, B. Q. Spring, I. Rizvi, C. L. Evans, K. S. Samkoe, S. Verma, B. W. Pogue and T. Hasan, Chem. Rev., 2010, 110, 2795–2838 CrossRef CAS PubMed.
- R. W. Redmond and J. N. Gamlin, Photochem. Photobiol., 1999, 70, 391–475 CrossRef CAS PubMed.
- S. Wang, G. Huettmann, Z. Zhang, A. Vogel, R. Birngruber, S. Tangutoori, T. Hasan and R. Rahmanzadeh, Mol. Pharm., 2015, 12, 3272–3281 CrossRef CAS PubMed.
- P. Rai, S. Mallidi, X. Zheng, R. Rahmanzadeh, Y. Mir, S. Elrington, A. Khurshid and T. Hasan, Adv. Drug Delivery Rev., 2010, 62, 1094–1124 CrossRef CAS PubMed.
- X. Feng, D. Jiang, T. Kang, J. Yao, Y. Jing, T. Jiang, J. Feng, Q. Zhu, Q. Song, N. Dong, X. Gao and J. Chen, ACS Appl. Mater. Interfaces, 2016, 8, 17817–17832 CAS.
- R. Weijer, M. Broekgaarden, M. Kos, R. van Vught, E. A. J. Rauws, E. Breukink, T. M. van Gulik, G. Storm and M. Heger, J. Photochem. Photobiol., C, 2015, 23, 103–131 CrossRef CAS.
- R. V. Kutty and S.-S. Feng, Biomaterials, 2013, 34, 10160–10171 CrossRef CAS PubMed.
- P.-Y. Li, P.-S. Lai, W.-C. Hung and W.-J. Syu, Biomacromolecules, 2010, 11, 2576–2582 CrossRef CAS PubMed.
- M. S. Muthu, S. A. Kulkarni, Y. Liu and S.-S. Feng, Nanomedicine, 2012, 7, 353–364 CrossRef CAS PubMed.
- Y. Zhao, J. Neuzil and K. Wu, Mol. Nutr. Food Res., 2009, 53, 129–139 CAS.
- A. B. Shapiro, K. Fox, P. Lam and V. Ling, Eur. J. Biochem., 1999, 259, 841–850 CrossRef CAS PubMed.
- J. M. Dintaman and J. A. Silverman, Pharm. Res., 1999, 16, 1550–1556 CrossRef CAS.
- W. H. Jiang, L. Yang, L. P. Qiu, J. W. Xu, X. C. Yang, J. Wang, H. Zhou and D. K. Wang, RSC Adv., 2015, 5, 53835–53845 RSC.
- W. Li, W. J. Zhang, K. Ohnishi, I. Yamada, R. Ohno and K. Hashimoto, J. Photochem. Photobiol., B, 2001, 60, 79–86 CrossRef CAS.
- R. M. Lemoli, T. Igarashi, M. Knizewski, L. Acaba, A. Richter, A. Jain, D. Mitchell, J. Levy and S. C. Gulati, Blood, 1993, 81, 793–800 CAS.
- C. S. Jin, J. F. Lovell, J. Chen and G. Zheng, ACS Nano, 2013, 7, 2541–2550 CrossRef CAS PubMed.
- S. A. Sibani, P. A. McCarron, A. D. Woolfson and R. F. Donnelly, Expert Opin. Drug Delivery, 2008, 5, 1241–1254 CrossRef CAS PubMed.
- T. Kang, X. L. Gao, Q. Y. Hu, D. Jiang, X. Y. Feng, X. Zhang, Q. X. Song, L. Yao, M. Huang, X. G. Jiang, Z. Q. Pang, H. Z. Chen and J. Chen, Biomaterials, 2014, 35, 4319–4332 CrossRef CAS PubMed.
- U. Prabhakar, H. Maeda, R. K. Jain, E. M. Sevick-Muraca, W. Zamboni, O. C. Farokhzad, S. T. Barry, A. Gabizon, P. Grodzinski and D. C. Blakey, Cancer Res., 2013, 73, 2412–2417 CrossRef CAS PubMed.
- Z. Hu, B. Rao, S. Chen and J. Duanmu, BMC Cancer, 2010, 10, 235–248 CrossRef CAS PubMed.
- E. E. Paoli, E. S. Ingham, H. Zhang, L. M. Mahakian, B. Z. Fite, M. K. Gagnon, S. Tam, A. Kheirolomoom, R. D. Cardiff and K. W. Ferrara, J. Controlled Release, 2014, 178, 108–117 CrossRef CAS PubMed.
- Z. Hua, S. Tam, E. S. Ingham, L. M. Mahakian, L. Chun-Yen, S. K. Tumbale, T. Teesalu, N. E. Hubbard, A. D. Borowsky and K. W. Ferrara, Biomaterials, 2015, 56, 104–113 CrossRef PubMed.
- K. Grabowska, A. K. Puszko, P. F. J. Lipinski, A. K. Laskowska, B. Wilenska, E. Witkowska and A. Misicka, Bioorg. Med. Chem. Lett., 2016, 26, 2843–2846 CrossRef CAS PubMed.
- C. A. Staton, I. Kumar, M. W. R. Reed and N. J. Brown, J. Pathol., 2007, 212, 237–248 CrossRef CAS PubMed.
- H. Y. Jia, A. Bagherzadeh, B. Hartzoulakis, A. Jarvis, M. Loehr, S. Shaikh, R. Aqil, L. L. Cheng, M. Tickner, D. Esposito, R. Harris, P. C. Driscoll, D. L. Selwood and I. C. Zachary, J. Biol. Chem., 2006, 281, 13493–13502 CrossRef CAS PubMed.
- O. Straume and L. A. Akslen, Angiogenesis, 2003, 6, 295–301 CrossRef CAS PubMed.
- M. Fakhari, D. Pullirsch, D. Abraham, K. Paya, R. Hofbauer, P. Holzfeind, M. Hofmann and S. Aharinejad, Cancer, 2002, 94, 258–263 CrossRef CAS PubMed.
- T. Ochiumi, Y. Kitadai, S. Tanaka, M. Akagi, M. Yoshihara and K. Chayama, Int. J. Oncol., 2006, 29, 105–116 CAS.
- S. Rizzolio and L. Tamagnone, Curr. Med. Chem., 2011, 18, 3563–3575 CrossRef CAS PubMed.
- K. N. Sugahara, T. Teesalu, P. P. Karmali, V. R. Kotamraju, L. Agemy, O. M. Girard, D. Hanahan, R. F. Mattrey and E. Ruoslahti, Cancer Cell, 2009, 16, 510–520 CrossRef CAS PubMed.
- Y. Liu, Q. Chen, M. Xu, G. N. Guan, W. Hu, Y. Liang, X. L. Zhao, M. X. Qiao, D. W. Chen and H. Liu, Int. J. Nanomed., 2015, 10, 1855–1867 CrossRef CAS PubMed.
- L. Roth, L. Agemy, V. R. Kotamraju, G. Braun, T. Teesalu, K. N. Sugahara, J. Hamzah and E. Ruoslahti, Oncogene, 2012, 31, 3754–3763 CrossRef CAS PubMed.
- B. Xu, Y. Ju, Y. Cui, G. Song, Y. Iwase, A. Hosoi and Y. Morita, Langmuir, 2014, 30, 7789–7797 CrossRef CAS PubMed.
- A. M. Matos, M. Reis, N. Duarte, G. Spengler, J. Molnar and M.-J. U. Ferreira, J. Nat. Prod., 2015, 78, 2215–2228 CrossRef CAS PubMed.
- W. Walther, D. Kobelt, L. Bauer, J. Aumann and U. Stein, Int. J. Oncol., 2015, 47, 2276–2285 Search PubMed.
- M. Yun, D. Lee, M.-N. Park, E.-O. Kim, E. J. Sohn, B.-M. Kwon and S.-H. Kim, Cell. Physiol. Biochem., 2015, 35, 1821–1830 CrossRef CAS PubMed.
- S.-K. Lee, A. Shehzad, J.-C. Jung, J.-K. Sonn, J.-T. Lee, J.-W. Park and Y.-S. Lee, Mol. Cells, 2012, 34, 61–69 CrossRef CAS PubMed.
- A. Dubey, J. W. Min, H. J. Koo, H. Kim, T. R. Cook, S. C. Kang, P. J. Stang and K.-W. Chi, Chem.–Eur. J., 2013, 19, 11622–11628 CrossRef CAS PubMed.
- H.-C. Chou, J.-Y. Chen, D.-Y. Lin, Y.-F. Wen, C.-C. Lin, S.-H. Lin, C.-H. Lin, T.-W. Chung, E.-C. Liao, Y.-J. Chen, Y.-S. Wei, Y.-T. Tsai and H.-L. Chan, J. Proteome Res., 2015, 14, 4907–4920 CrossRef CAS PubMed.
- R. Baptista, R. J. Ferreira, D. J. V. A. dos Santos, M. X. Fernandes and M.-J. U. Ferreira, Future Med. Chem., 2016, 8, 629–645 CrossRef CAS PubMed.
- K. Tokarska, M. Bulka, U. Bazylinska, E. Jastrzebska, M. Chudy, A. Dybko, K. A. Wil and Z. Brzozka, J. Pharm. Biomed. Anal., 2016, 127, 39–48 CrossRef CAS PubMed.
- N. Okahashi, M. Nakata, H. Kuwata and S. Kawabata, Infect. Immun., 2016, 84, 2042–2050 CrossRef PubMed.
- J. W. Tian, L. Ding, H. X. Ju, Y. C. Yang, X. L. Li, Z. Shen, Z. Zhu, J. S. Yu and C. J. Yang, Angew. Chem., Int. Ed., 2014, 53, 9544–9549 CrossRef CAS PubMed.
- X. Cheng, H. Yan, X. Jia and Z. Zhang, J. Drug Targeting, 2016, 24, 161–168 CAS.
- R. Palao-Suay, M. Rosa Aguilar, F. J. Parra-Ruiz, M. Fernandez-Gutierrez, J. Parra, C. Sanchez-Rodriguez, R. Sanz-Fernandez, L. Rodriganez and J. San Roman, Biomacromolecules, 2015, 16, 1566–1581 CrossRef CAS PubMed.
- K. Berg, P. K. Selbo, A. Weyergang, A. Dietze, L. Prasmickaite, A. Bonsted, B. O. Engesaeter, E. Angell-Petersen, T. Warloe, N. Frandsen and A. Hogset, J. Microsc., 2005, 218, 133–147 CrossRef CAS PubMed.
- G. Gu, X. Gao, Q. Hu, T. Kang, Z. Liu, M. Jiang, D. Miao, Q. Song, L. Yao, Y. Tu, Z. Pang, H. Chen, X. Jiang and J. Chen, Biomaterials, 2013, 34, 5138–5148 CrossRef CAS PubMed.
Footnote |
† Electronic supplementary information (ESI) available. See DOI: 10.1039/c6ra19152e |
|
This journal is © The Royal Society of Chemistry 2016 |