DOI:
10.1039/C6RA18945H
(Paper)
RSC Adv., 2016,
6, 79978-79986
Aryl-fused tetrathianaphthalene (TTN): synthesis, structures, properties, and cocrystals with fullerenes†
Received
26th July 2016
, Accepted 15th August 2016
First published on 16th August 2016
Abstract
1,4,5,8-Tetrathianaphthalene (TTN) is the structural isomer of tetrathiafulvalene (TTF). Herein, the title aryl-fused TTNs (TTN1–TTN9) have been synthesized from a zinc–thiolate complex (TBA)2[Zn(DMIT)2] via a two-fold Cu-catalyzed C–S coupling reaction. These TTNs can be transformed into the corresponding aryl-fused TTF isomers by reaction with FeCl3. The electronic properties of these TTNs were studied by absorption spectroscopy, electrochemistry, and theoretical calculations, which reveal that these TTNs show weak electron donating ability as compared with their TTF isomers. Crystallographic analyses indicate that these TTNs take the chair-like conformation, i.e., having two curved surfaces that are of shape complementary to fullerene molecules. Consequently, these TTNs show strong affinity toward C60/C70 to form cocrystals. In the cocrystals, the fullerene molecules are encapsulated by the curved surfaces of TTN molecules through multiple intermolecular atomic contacts. While the interaction modes between TTNs and fullerene molecules are very similar, the packing patterns of the cocrystals can be finely tuned by varying the solvents. Moreover, the fullerene molecules form tight stacks as column arrays or two-dimensional sheets.
Introduction
Tetrathiafulvalene (TTF) and its derivatives have received tremendous attention in recent decades owing to their wide applications in molecular electronic materials.1 Chemically speaking, the TTF framework is constructed from tetrathia-substituted ethene by connecting its sulfur centers with ethene bridges in an end-on style (Scheme 1). On the other hand, the incorporation of ethene bridges onto tetrathia-substituted ethene via the side-on style results in 1,4,5,8-tetrathianaphthalene (TTN), which is also named as 1,4,5,8-tetrathiatetralin.2 As a structural isomer of TTF, TTN can be converted to TTF under certain conditions.3
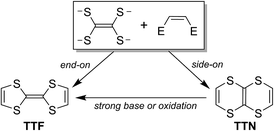 |
| Scheme 1 Schematic depiction for the derivation of TTF and TTN from tetrathia-substituted ethene, along with transformation of TTN into TTF. | |
While TTN and TTF are highly related in chemistry, they show quite different features in both structural and electronic aspects. The TTF framework is of planar shape, particularly in the cation radical state.1 However, the TTN skeleton adopts the chair-like conformation, and the dihedral angle between terminal ethene and central tetratia-enthene is about 137°.2b,c TTF possesses two reversible redox potentials, and can form stable cation radical specie under the accessible potential. The charge-transfer complexes and/or cation radical salts of TTF and its derivatives shows various electronic states including metallic, charge-ordering, Mott insulating, superconducting, and so on.4 On the contrary, TTN has a first redox potential much higher than TTF, and its second redox potential is irreversible. Consequently, the TTN series have drawn less attention because of the weak electron-donating ability that limit the formation of stable and conducting cation radical salts.2c,3a
Recently, the aryl substituted or fused TTF derivatives have received tremendous attentions owing to their applications in organic electronic materials, such as OFET,5 OPV,6 molecular switch,7 conducting MOFs/COFs.8 However, the aryl-fused TTNs are less developed, that limit the further understanding of this system. Concerning the facile transformation of TTN into TTF, the aryl-fused TTNs can be used to create new types of aryl-fused TTFs. Meanwhile, TTN is of chair conformation, thus the aryl-fused TTNs possess two curved π-surfaces that have advantages to encapsulate molecules with spherical surface, for example, fullerene molecules. Herein, we report the synthesis of aryl-fused TTN via two-fold Cu-catalyzed C–S coupling reaction. The crystal structures, optical and electrochemical properties, and the complexation with fullerene are also evaluated. The present work reveals that the aryl-fused TTN show strong tendency to form the cocrystals with fullerene molecules, and the fullerenes form the tight columns and/or two-dimensional sheets in the cocrystals.
Results and discussion
Synthesis
The aryl-fused TTNs (TTN1–TTN9) were synthesized via the two-fold C–S coupling reactions as shown in Scheme 2. As a represent example, the synthesis of TTN3 is reported herein. The Cu2O-catalyzed C–S coupling of (TBA)2[Zn(DMIT)2] and 3,4-diiodo-2,5-dimethylthiophene resulted in 2,5-dimethylthiophene-fused 1,3-dithiole-2-thione, which was then converted to the corresponding 1,3-dithiole-2-one (1c) by reaction with Hg(OAc)2.9 Compound 1c was hydrolyzed by CH3ONa to afford [1,4]dithiine-2,3-dithiolate sodium salt,10 which reacted with TBA·Br and ZnCl2 to form a zinc–thiolate complex 2c. Complex 2c was directly used in the next step without purification. The Cu2O-catalyzed C–S coupling reaction between 3,4-diiodo-2,5-dimethylthiophene and 2c afforded TTN3 with isolated yield of 86%. It should be noted that compound 1e shows poor solubility in the solvent of CH3OH–THF (1
:
1, v/v), which makes the preparation of complex 2e quite difficult. Consequently, the isolated yield of TTN9 is much lower than the others. Needless to say, this is the first synthesis of aryl-fused TTNs and most of them are created in good yields.
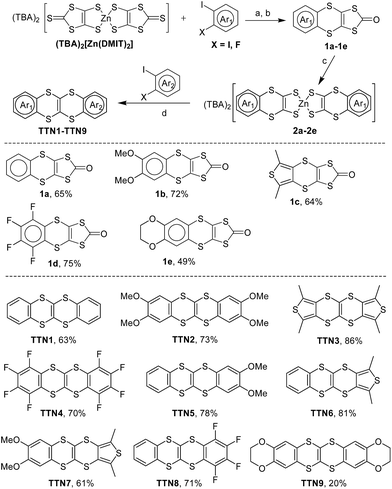 |
| Scheme 2 Synthetic approach with isolated yields for intermediates (1a–1e) and final products (TTN1–TTN9) shown. Reaction conditions: (a) Cu2O (mol 20%), ethyl acetoacetate (mol 40%), DMF, 80 °C, 10 h; (b) Hg(OAc)2 (3 equiv.), CHCl3, r.t., 5 h; (c) NaOCH3 (2.5 equiv.), THF–CH3OH (1 : 1, v/v), r.t., 1 h; then added the mixture of ZnCl2 (1 equiv.) and TBA·Br (1.5 equiv.) in CH3OH solution, 30 min; (d) Cu2O (mol 20%), ethyl acetoacetate (mol 40%), DMF, 120 °C, 10 h. | |
It has been revealed that TTN can be transformed into TTF under the electrochemical oxidation and/or chemical oxidation by Pb(OAc)4.3a However, we found that the electrochemical oxidation of the present TTNs didn't afford the corresponding TTF isomers. For example, the repeated cyclic voltammetry (CV) of TTN3 only shows the redox waves of TTN3 without the typical CV signals of TTF derivatives (see Fig. S1 in the ESI†). Moreover, the oxidation of TTN3 by Pb(OAc)4 resulted in its TTF isomer in very low yield (<5%). Instead, we found that these TTNs can be converted to the TTF isomers in high yield by reaction with FeCl3. As a typical example, TTN3 was almost quantitatively converted to TTF3 by reaction with FeCl3 in CHCl3 solution under reflux, as shown in Scheme 3. The synthesis of TTF from TTNs and the elucidation of their properties is undergoing.
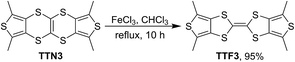 |
| Scheme 3 Transformation of TTN3 into TTF3. | |
Crystal structures
By recrystallization or slow evaporation of solvents, TTN1–TTN4, TTN6, and TTN9 afforded the single crystals suitable for the X-ray diffraction analysis. To gain insight into the difference of molecular and packing structures between TTN and TTF, the crystal structure of TTF3 is also reported. The selected crystallographic data are provided in ESI (Table S2†) and the detailed data have been submitted to CCDC.11 It is wordy to discuss the crystal structure for each TTN, herein we report the crystal structures of TTN3 as a representative example; those of the other TTNs can be found in ESI (Fig. S20–S24†).
The colourless thin platelet single crystals of TTN3 and TTF3 were obtained by slow evaporation of their solution in carbon disulfide (CS2) and dichloromethane (CH2Cl2), respectively. TTN3 crystallizes in P21/c space group with half of TTN3 molecule and half of solvent molecule CS2 crystallographically unique. TTF3 crystallizes in I2/c space group with half of TTF3 molecule crystallographically unique. Fig. 1 depicts the crystal structure of TTN3, along with that of TTF3 for comparison. Referring Fig. 1a, the TTN3 adopts a chair conformation. The dihedral angle between the central tetrathiaethene moiety (C2S4) and terminal 2,5-dimethyl-3,4-dithiathiophene is 132°. On the contrary, TTF3 takes a nearly planar conformation (Fig. 1b), similar to that of thiophene-fused TTF (DT-TTF).5a,c,12 While TTN3 and TTF3 have the different molecular geometries, the bond lengths on them are almost identical (see Fig. 1c and d). For example, the C
C bond lengths of tetrathiaethene moiety on TTN3 and TTF3 are 1.34 Å and 1.35 Å, respectively.
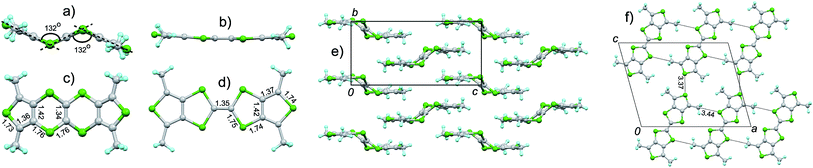 |
| Fig. 1 Crystal structure of TTN3 and TTF3. (a) Top view and (b) side view of TTN3 with the selected bond lengths and dihedral angle shown; (c) top view and (d) side view of TTF3 with the selected bond lengths shown; (e) packing structure of TTN3 viewed along the a-axis with CS2 molecules omitted for clarity; (f) packing structure of TTF3 viewed along the b-axis with the selected intermolecular short contacts shown. | |
The packing structure of TTN3 is shown in Fig. 1e. The main driven forces arise from the C–H⋯π interactions between the 2,5-dimethylthiophenes of the neighbouring TTN3 molecules. The central C2S4 moieties of the neighbouring TTN3 molecules form a void space which accommodates the solvent CS2 molecule. In the crystal structure of TTF3, various intermolecular interactions are observed. There are C–H⋯π interactions between the 2,5-dimethylthiophenes of neighbouring TTF3 molecules. Moreover, there are C–S atomic short contacts (3.44 Å)13 between the methyl group and the central TTF skeleton, and S–S atomic short contacts (3.37 Å) between the 2,5-dimethylthiophenes as well. As compared with TTF3, TTN3 form the relatively loose pack structure, which is ascribable to the non-planar conjugated system. For the other TTNs so far obtained, the molecular geometries are very similar to that of TTN3. The dihedral angle between the central C2S4 moiety and the terminal aryl is in the range of 132–138°, which depends on the aryl groups (Fig. S20–S24†).
Electronic structures
The electronic properties of the present TTNs are elucidated by means of theoretical calculation, and electrochemical and optical investigations. Herein, we report the electronic properties of TTN3 as a representative example, and those of the others are provided in ESI (Fig. S2–S18†).
Fig. 2 depicts the frontier molecular orbitals and the energy diagram of TTN3 and TTF3, which is calculated on the basis crystal structure by the means of B3LYP/6-31G(d,p) level of theory. The highest occupied molecular orbital (HOMO) of TTN3 is distributed on the whole conjugated skeleton, but the central tetrathia-ethene moiety (C2S4) shows the largest contribution. Meanwhile, the lowest unoccupied molecular orbital (LUMO) is mainly located on thiophene rings. This means the intramolecular charge-transfer, most plausibly between the thiophene ring and the central C2S4 moiety, would play the significant contribution on the low-energy electronic transition of TTN3. On the other hand, both HOMO and LUMO orbitals of TTF3 are delocalized on the whole molecular skeleton, indicating the extension of the conjugated π-electron system would be the key factor for the electronic transition. The HOMO energy level of TTN3 (EHOMO = −5.264 eV) is lower than that of TTF3 (EHOMO = −4.811 eV), and the LUMO energy level of TTN3 (ELUMO = −0.868 eV) is higher than that of TTF3 (ELUMO = −1.000 eV). Consequently, TTN3 has a HOMO–LUMO energy gap (E = 4.40 eV) larger than that of TTF3 (E = 3.81 eV). The discrepancy between the molecular orbitals of TTN3 and TTF3 would be ascribable to their distinct molecular geometry, the former is in deep chair and the latter is planar as reported in the crystal structure section.
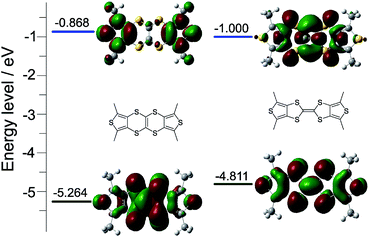 |
| Fig. 2 Calculated HOMO and LUMO orbitals of TTN3 and TTF3 by means of the B3LYP/6-31G(d,p) level of theory. | |
The electrochemical property of TTN3 was investigated by cyclic voltammetry (CV) and differential pulse voltammetry (DPV) as shown in Fig. 3. The DPV study indicates that TTN3 has two oxidation peaks at 1.09 V (E1ox) and 1.57 V (E2ox), which are clearly higher than those of TTF3 (E1ox = 0.73 V, E2ox = 1.18 V). This result is consistent with the theoretical investigation, i.e., the HOMO energy level of TTN3 is lower than that of TTF3. Moreover, the CV investigation shows that the first redox wave (E11/2) of TTN3 is reversible and the second redox wave (E21/2) is irreversible. This feature is quite different from that of TTF3, which has two reversible redox waves. As summarized in Table 1, most of the TTNs possess two oxidation potentials and the first oxidation potential (E1ox) increases as the electron-withdrawing ability of the aryls increases. Among the present TTNs, TTN4 doesn't show clear oxidation peaks, which would be attributed to the sufficiently low π-electron density on TTN core caused by the inductive effect of the electropositive peripheral C6F4 ring.14 The similar situation also observed in for TTN8, which doesn't show the second oxidation peak.
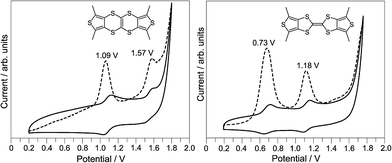 |
| Fig. 3 Cyclic (solid line) and DPV (dashed line) voltammograms of TTN3, along with those of TTF3 for comparison. | |
Table 1 Electrochemical properties of TTNsa
|
E1ox [V] |
E1red [V] |
E11/2 [V] |
E2ox [V] |
E2red [V] |
E21/2 [V] |
ΔEb [V] |
Measurement conditions: solvent CH2Cl2, concentration 5 × 10−4 mol L−1, supporting electrolyte (n-Bu)4NPF6, working electrode Pt/C, counter electrode Pt, reference electrode SCE, scan speed 50 mV s−1, temperature 20 °C. ΔE = E21/2 − E11/2. |
TTN1 |
1.16 |
1.08 |
1.12 |
1.58 |
— |
— |
— |
TTN2 |
0.98 |
0.90 |
0.94 |
1.40 |
1.32 |
1.36 |
0.42 |
TTN3 |
1.09 |
1.01 |
1.05 |
1.57 |
1.48 |
1.52 |
0.47 |
TTN4 |
— |
— |
— |
— |
— |
— |
— |
TTN5 |
1.01 |
0.95 |
0.98 |
1.47 |
1.41 |
1.44 |
0.46 |
TTN6 |
1.14 |
1.04 |
1.09 |
1.56 |
— |
— |
— |
TTN7 |
1.06 |
0.96 |
1.01 |
1.49 |
1.42 |
1.45 |
0.44 |
TTN8 |
1.38 |
1.30 |
1.34 |
— |
— |
— |
— |
TTN9 |
1.02 |
0.94 |
0.98 |
1.48 |
1.38 |
1.43 |
0.45 |
TTF3 |
0.73 |
0.66 |
0.70 |
1.18 |
1.10 |
1.14 |
0.44 |
The UV/Vis absorption spectra of TTN1–TTN9 were recorded in CH2Cl2 solution and solid state (dispersing in KBr pellet) at 25 °C. These TTNs show the almost identical absorption spectra. Fig. 4 depicts the absorption spectra of TTN3. This compound displays a sharp absorption peak at λmax = 278 nm, and a broad absorption band at 325–375 nm with moderate absorbance in the CH2Cl2 solution (Fig. 4a). On the other hand, TTF3 shows a maximum and relatively broad absorbance band at λmax = 310 nm, and a very weak and much broad absorbance band at 365–450 nm in the CH2Cl2 solution (Fig. 4b). This is consistent with the theoretical calculation, which shows that TTN3 has a HOMO–LUMO energy gap larger than that of TTF3. For both compounds, the absorption band show red-shift in solid state, reflecting the intermolecular interaction in solid state as reported in crystal structure section.
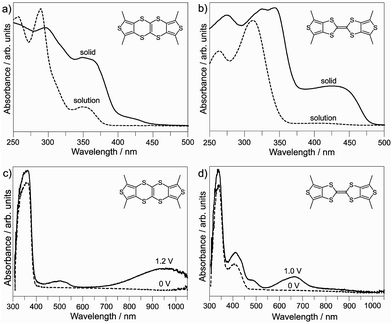 |
| Fig. 4 UV-Vis absorption spectra of TTN3 and TTF3 under the different condition: absorption spectra of (a) TTN3 and (b) TTF3 in CH2Cl2 solution (c = 10−5 mol L−1) and solid state (dispersed in the KBr pellet); spectroelectrochemistry of (c) TTN3 and (d) TTF3 in the CH2Cl2 solution (c = 10−5 mol L−1). | |
To further understand the difference between TTN3 and TTF3, the absorption spectra of them at the different oxidation state were studied by means of spectroelectrochemistry. Upon electrochemical oxidation of TTN3 at 1.2 V in CH2Cl2 solution, which convert TTN3 to its cation radical state [TTN3]+˙, two new broad bands were observed as centered at 500 nm and 950 nm (Fig. 4c). It should be noted that [TTN]+˙ displays the similar absorption bands centered at 450 nm and 900 nm.15 As reported by Kirketerp et al.,15 both bands of [TTN]+˙ correspond to the π–π* transition, whereas the former (450 nm) and the latter (900 nm) originate from the SOMO → (SOMO+1) and (SOMO−1) → SOMO transitions, respectively. In comparison with [TTN]+˙, the absorption bands of [TTN3]+˙ show the clear red-shifts, which would be ascribable to the extension of π-electron system by introducing the peripheral aryls. The oxidation of TTF3 also resulted in two new broad absorption bands centered at 475 nm and 650 nm. The former and the latter correspond to the π–π* transitions of SOMO → (SOMO+2) and (SOMO−1) → SOMO, respectively.15
Cocrystals with fullerenes
The complexes of TTF derivatives and fullerene molecules show various electronic properties.16,17 Recently, we have reported that the arylthio-substituted TTF derivatives (Ar–S–TTF) are promising receptors for fullerene molecules (C60 and C70).18 For example, tetra(3-methoxybenzene-2-thio)-TTF (TMBT-TTF) can encapsulate C60 to form 1
:
1 cocrystal by evaporating their mixed solution in carbon disulfide (CS2).18a As shown in the crystal structure section, the present TTNs adopt the chair-like conformation with two curved surfaces formed by the central C2S4 core and the terminal aryls. Concerning the round surface of fullerene molecules, the present TTNs would show strong propensity to encapsulate fullerene molecules.
We compared the affinity of TTNs and Ar–S–TTFs toward fullerenes. As a representative example, the complexation of C60 with TTN3 and TMBT-TTF was investigated. C60 (1 mmol) was introduced to a mixed solution of TTN3 and TMBT-TTF (1 mmol
:
1 mmol) in CS2, and the resulting solution was kept under a nitrogen gas flow to evaporate CS2 slowly. After 5 days, the red crystals of TMBT-TTF were isolated and the black block-like complex of TTN3 and C60 was formed, as confirmed by the thin layer chromatography. We also investigated the affinity of TTN3 and TTF3 toward C60. When C60 was introduced to a mixed solution of TTN3 and TTF3 (molar ratio, 1
:
1) in CS2, only TTN3 formed the complex with C60 and TTF3 was recovered. These results reveal that TTN3 show much stronger affinity toward C60 than TMBT-TTF and TTF3. Regarding the electron-donating ability, TTF3 (E11/2 = 0.70 V) and TMBT-TTF (E11/2 = 0.57 V) are better than TTN3 (E11/2 = 1.05 V). Therefore, the strong affinity of TTN3 toward C60 is not originated from the so-called donor–acceptor type interaction, but ascribable to the intrinsically curved skeleton, i.e., the shape complementary with fullerene molecules.
To gain further insight on the structures of TTN–fullerene complexes, the single crystalline complexes of the present TTNs with C60/C70 were prepared by slow evaporation of their mixed solution. All of the present TTNs can form crystalline complexes with C60 and/or C70, whereas only several of them afforded the crystals suitable for X-ray single crystal diffraction measurement.19 The compositions of the complexes were determined on the basis of single crystal structure analyses. We found that the solvent played the pivotal role on the compositions and packing structures of the resulting complexes. As representative examples, herein we report the structures of the complexes of TTN3 with C60 and C70, and those of the others are shown in ESI (Fig. S26–S27†). The complexes of TTN3 with C60/C70 were prepared in the different solvents, CS2 and chlorobenzene (PhCl). The complexes formed in CS2 solution have the compositions of (TTN3)·(C60)·(CS2) and (TTN3)·(C70)·(CS2), and those cropped in PhCl solution are (TTN3)·(C60) and (TTN3)·(C70). In the following, the structure of each complex is reported.
(TTN3)·(C60)·(CS2)
This complex crystallizes in the triclinic P
space group with half of TTN3, half of C60, and half of CS2 crystallographically unique. Fig. 5 shows the crystal structure of this complex. In the complex, TTN3 molecule takes the chair conformation similar to that observed in its crystal structure, whereas the dihedral angle (137°) between the C2S4 moiety and 2,5-dimethyl-3,4-dithiathiophene is slightly enlarged. As we expected, both curved surfaces of TTN3 encapsulate a C60 molecule through multiple intermolecular van der Waals interactions: five C–C contacts of 3.20–3.34 Å and two C–S contacts of 3.42 Å (Fig. 5a). This would be ascribable to the shape complementary between TTN3 and C60. A C60 molecule is sandwiched by two TTN3 molecules, and they form the chain-like stacks along the crystallographic (b–c) direction (Fig. 5b). On the other hand, the C60 molecules form the columnar stacks along the a-axis direction. Within stack column, the neighboring C60 molecules are connected through four C–C short contacts (3.35–3.36 Å).
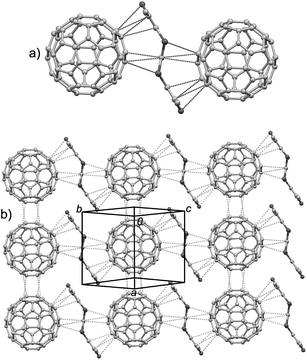 |
| Fig. 5 Crystal structure of (TTN3)·(C60)·(CS2): (a) intermolecular interactions between TTN3 and C60; (b) packing structure. The dashed lines represent the intermolecular short contacts. The hydrogen atoms and solvent molecule CS2 are omitted for clarity. | |
(TTN3)·(C60)
This complex is in the orthorhombic Pnma space group with half of TTN3 and half of C60 crystallographically unique. Fig. 6 depicts the crystal structure of this complex. Similar to (TTN3)·(C60)·(CS2), the C60 molecule locates at the curved surface of TTN3, and there are multiple intermolecular van der Waals interactions between the TTN3 and C60: five C–C contacts of 3.07–3.39 Å and three C–S contacts of 3.35–3.47 Å (Fig. 6a). Consequently, the sandwich type combination of C60 and TTN3 grows along the b-axis direction to form the chain-like stacks (Fig. 6b). Unlike (TTN3)·(C60)·(CS2), the C60 molecules in this complex form two dimensional sheets in the crystallographic ac-plane through multiple intermolecular C–C short contacts (3.16–3.24 Å).
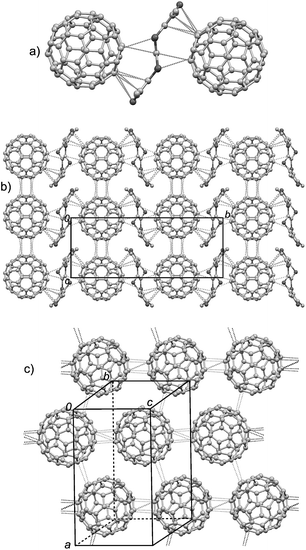 |
| Fig. 6 Crystal structure of (TTN3)·(C60): (a) intermolecular interactions between TTN3 and C60; (b) packing structures projected along the a-axis; (c) packing structure of C60 molecules. The dashed lines shows the intermolecular interactions and the hydrogen atoms are omitted for clarity. | |
(TTN3)·(C70)·(CS2)
This complex crystallizes in the triclinic P
space group, and the asymmetric unit contains one TTN3, one C70, and two halves of CS2. The crystal structure of this complex is shown in Fig. 7. In the complex, one C70 molecule is sandwiched by two TTN3 molecules, and the long axis of C70 is almost parallel to the central C
C bond of TTN3 (Fig. 7a). There are multiple intermolecular van der Waals interactions between the TTN3 and C70: four C–C contacts of 3.29–3.36 Å and three C–S contacts of 3.37–3.48 Å. Consequently, the –TTN3–C70–TTN3–C70– stacking chain is formed along the crystallographic b-axis direction (Fig. 7b). The C70 molecules form the columnar stacks along the a-axis direction. Within a stack column, there are four C–C atomic short contacts (3.19–3.33 Å) between the neighboring C70 molecules.
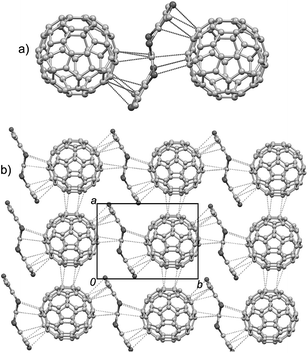 |
| Fig. 7 Crystal structure of (TTN3)·(C70)·(CS2): (a) the intermolecular interactions between TTN3 and C70; (b) packing structure viewed along the c-axis. The dashed lines represent the intermolecular interactions and the hydrogen atoms are omitted for clarity. | |
(TTN3)·(C70)
This complex is in the triclinic P
space group with two halves of TTN3 (molecules A and B) and one C70 molecule crystallographically unique. Fig. 8 depicts the crystal structure of this complex. The TTN3 molecules A and B show the different interaction modes with C70 molecule (Fig. 8a). Molecule A is surrounded by four C70 molecules: two C70 molecules locate at the curved surfaces of molecule A (one C–C contact, 3.39 Å; two C–S contacts, 3.43 Å and 3.44 Å), and two C70 molecules have the interactions with molecule A along its lateral axis with two C–S contacts (3.29 Å and 3.47 Å). In the case of molecule B, two C70 molecules are located at its curved surfaces with three C–C contacts (3.22 Å, 3.27 Å, and 3.30 Å). Consequently, TTN3 and C70 molecules form the columnar arrays in manner of –A–C70–B–C70– along the crystallographic (b + c) direction, which is different from those observed in the above three complexes. As shown in Fig. 8b, the C70 molecules in this complex form the two-dimensional sheets in the crystallographic ac-plane, and there are multiple intermolecular C–C atomic contacts (3.02–3.39 Å) between the neighboring C70 molecules.
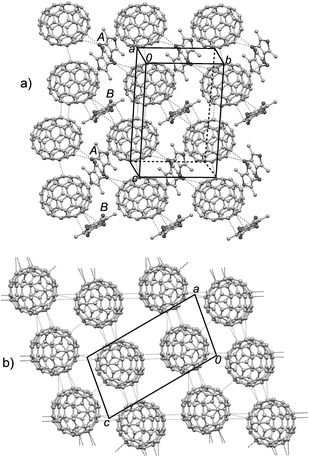 |
| Fig. 8 Crystal structure of (TTN3)·(C70): (a) packing structure; (b) packing structures of C70 molecules viewed along the b-axis. The dashed lines represent the intermolecular atomic short contacts and the hydrogen atoms are omitted for clarity. | |
The above results demonstrate that the aryl-fused TTNs are much better receptors for fullerene molecules, as compared with their corresponding TTF isomers and Ar–S–TTFs as well. The strong affinity of the aryl-fused TTNs toward fullerene molecules arises from their intrinsic non-planar skeleton, i.e., the chair-like conformation has two curved surfaces that show the shape complementary with fullerene molecules. Moreover, the structures of “TTN·fullerene” cocrystals strongly depend on the solvents, particularly the packing motifs of fullerene molecules. Therefore, the “TTN·fullerene” cocrystals could be finely tuned in varying the experimental conditions, and lead to tight columnar arrays or two-dimensional sheets of fullerene molecules.
Previously, we have reported that the cocrystals of Ar–S–TTF and fullerenes have neutral ground state and charge-transfer excited state to display long wavelength absorbance centred at 800 nm.18a The “TTN·fullerene” cocrystals also show neutral ground state, since their IR spectra are interpreted as the superpositions of TTN and fullerene (Fig. S28 and S30†). Meanwhile, “TTN·fullerene” cocrystals do not exhibit low energy absorbance (Fig. S29 and S31†). Hence, there is no electronic communication between TTN and fullerene molecules in both the ground and excited states of “TTN·fullerene” cocrystals. The difference between the electronic structures of “TTN·fullerene” and “Ar–S–TTF·fullerene” cocrystals would be ascribable to the electron-donating issue, as the first oxidation potential of Ar–S–TTF (E1ox = 0.60 ± 0.05 V) is much lower than that of the present TTNs (E1ox > 0.98 V). Concerning the tuneable packing of fullerene molecules which are neutral in both ground and excited states, the “TTN·fullerene” cocrystals are of interest to elucidate the intrinsic transport behaviours of fullerene molecules in the one- and two-dimensional stacks.
Conclusions
A library of aryl-fused tetrathianaphthalenes (TTN1–TTN9) have been synthesized from a readily available zinc–thiolate complex (TBA)2[Zn(DMIT)2] through two-fold C–S coupling reaction. The spectroscopic, electrochemical, and theoretical investigations reveals that the electron-donating ability of the present TTNs are much weaker than the corresponding TTF isomers. Unlike their planar TTF isomers, these TTNs adopt the chair conformation, and the curved surfaces on them show shape complementary with fullerene molecules. Consequently, they can play as receptors for fullerene molecules to form the “TTN·fullerene” cocrystals. In the resulting cocrystal, the fullerene molecule is sandwiched by TTN molecules through multiple intermolecular interactions, and the stacks of fullerene molecules can be finely tuned as two-dimensional sheets or columnar arrays in varying experimental conditions. Apart from their application in the supramolecular chemistry, these TTNs can be facilely transformed into their TTF isomers. Therefore, they would be employed to create novel aryl-fused TTFs that have potential applications in fabricating organic electronics.5
Experimental
General methods
Melting points (Mp) were determined on WRS-2 melting point apparatus. All of the new compounds in this report were identified by 1H NMR, 13C NMR, 19F NMR (for TTN4 and TTN9), and high-resolution mass spectra (HRMS), and/or X-ray single crystal diffraction. 1H, 19F and 13C NMR spectra were recorded on a Bruker Avance III 400 MHz (376 MHz and 100 MHz for 19F and 13C, respectively) spectrometer. The chemical shifts of 1H and 13C NMR were recorded using TMS as internal standard. 19F NMR chemical shifts were determined relative to CFCl3 at δ = 0.0. The HRMS was carried out on a maXis 4G mass spectrometer (Bruker Daltonic Inc.).
The UV-Vis spectra were recorded on Lambda 35 spectrometer (Varian). The in situ investigation of absorption spectra for TTN3 and TTF3 under constant potential electrochemical-oxidation was performed on a Zahner CIMPS type photo-electrochemical workstation. The IR spectra were record on the PerkinElmer Spectrum 400 spectrometer with the resolution of 2 cm−1. The CV and DPV were recorded on a RST 5000 electrochemical workstation at a scan rate of 50 mV s−1, with glassy carbon discs as the working electrode, Pt wire as the counter electrode, and SCE electrode as the reference electrode. The concentration was 1 × 10−4 mol L−1 in CH2Cl2, and the supporting electrolyte was (n-Bu)4N·PF6 (0.1 mol L−1). The measurement was performed at 20 °C after bubbling the solution with N2 gas for 15 min.
The X-ray single crystal diffraction measurement was carried out on a SuperNova (Agilent) type diffractometer at room temperature for TTN1–TTN4, TTN6, and TTN9, and at 110 K for (TTN3)·(C60)·(CS2), (TTN3)·(C70)·(CS2), and (TTN3)·(C60). The single crystal diffraction measurement of cocrystal (TTN3)·(C70) was conducted by means of synchrotron radiation at 77 K. The crystal structures of TTNs and cocrystals were solved by a direct method SIR2004 (ref. 20a) and refined by full-matrix least-square method on F2 by means of SHELXL-97.20b Calculated positions of the hydrogen atoms (d(C–H) = 0.95 Å) were included in the final refinement.
All calculations were carried out with the Gaussian 09 programs.21a For DFT calculations, we used the hybrid gradient corrected exchange functional of Lee, Yang, and Parr.21b,c A standardized 6-31G basis set21d was used together with polarization (d) and (p) functions.
Synthesis
Compounds 1a–1e are synthesized according to the literature.9 The TTN1–TTN9 are synthesized in the same strategies. As a representative example, the synthesis of TTN3 as well as its transformation into TTF3 is shown as followings.
2c. The compound 1c (1.79 g, 7.0 mmol) and anhydrous THF (30 mL) were placed in a 100 mL three-necked flask under the inert atmosphere, then the freshly prepared CH3ONa solution in CH3OH (Na, 0.40 g, 17.5 mmol; CH3OH, 15 mL) was added dropwisely. The resulting mixture was stirred at room temperature for 1 h, and the solution changed from colorless to dark red. To this solution were added a mixed solution of ZnCl2 (0.95 g, 7.0 mmol) and TBA·Br (3.48 g, 10.5 mmol) in CH3OH (15.0 mL). After 0.5 h, the solvent was removed by evaporation under reduced pressure to afford compound 2c as red solid, which was directly used in the next step without purification.
TTN3. The crude compound 2c, 3,4-diiodo-2,5-dimethylthiophene (2.55 g, 7 mmol), Cu2O (0.10 g, 0.70 mmol), ethyl acetoacetate (0.18 g, 1.40 mmol), and DMF (20.0 mL) were placed in a 50 mL flask and heated to 120 °C under inert atmosphere. After stirred at this temperature for 10 h, the reaction mixture was cooled down to room temperature and poured into the distilled water (30 mL). The mixture was extracted by CS2 (3 × 15 mL), and the organic layer was combined and dried with anhydrous Na2SO4. The crude product was purified by column chromatography on silica gel (eluent: CS2) to afford TTN3 as white solid (2.24 g, yield 86%). Mp: 294.3–295.2 °C; 1H NMR (400 MHz, CDCl3) δ 2.30 (s, 12H); 13C NMR (100 MHz, CDCl3) δ 129.69, 127.96, 125.45, 13.62; HRMS (C14H12S6): calc. 371.9258, found 371.9258.
TTF3. Compound TTN3 (0.93 g, 2.5 mmol), FeCl3 (0.81 g, 5 mmol), and CHCl3 (50 mL) were placed in a 100 mL flask and heated to reflux under inert atmosphere. After stirred at this temperature for 5 h, the reaction mixture was cooled down to room temperature and poured into the distilled water (30 mL). The organic layer was separated and washed with distilled water (3 × 10 mL), then dried with anhydrous Na2SO4. The crude product was purified by column chromatography on silica gel (eluent: CS2) to afford TTF3 as yellow solid (0.88 g, yield 95%). Mp: 298.8–300.1 °C; 1H NMR (400 MHz, CDCl3) δ 2.29 (s, 1H); 13C NMR (100 MHz, CDCl3) δ 131.13, 122.49, 119.60, 14.48; HRMS (C14H12S6): calc. 371.9258, found 371.9256.
Acknowledgements
This work was financially supported by National Nature Scientific Foundation of China (21522203, 21372111, and 21190034). The authors are grateful to Dr Yongliang Shao for the X-ray single crystal diffraction measurement. The synchrotron radiation experiment was conducted at 3W1A beam line of Beijing Synchrotron Radiation Facility (BSRF) at Institute of High Energy Physics, Chinese Academy of Science, Beijing.
Notes and references
-
(a) TTF Chemistry: Fundamentals and Applications of Tetrathiafulvalenes, ed. J. Yamada and T. Sugimoto, Kodansha and Springer, Tokyo, Berlin, Heidelberg, New York, 2004 Search PubMed;
(b) P. Batail, Chem. Rev., 2004, 104, 4887 CrossRef CAS;
(c) P. J. Skabara and M. Sallé, Beilstein J. Org. Chem., 2015, 11, 1528 CrossRef CAS PubMed.
-
(a) M. Mizuno, M. P. Cava and A. F. Garito, J. Org. Chem., 1976, 41, 1484 CrossRef CAS;
(b) H. Bock, B. Roth, M. V. Lakshmikantham and M. P. Cava, Phosphorus Sulfur Relat. Elem., 1984, 21, 67 CrossRef CAS;
(c) K. S. Varma, N. Sasaki, R. A. Clark, A. E. Underhill, O. Simonsen, J. Becher and S. Bøwadt, J. Heterocycl. Chem., 1988, 25, 783 CrossRef CAS;
(d) S. Nakatsuji, Y. Amano, H. Kawamura and H. Anzai, J. Chem. Soc., Chem. Commun., 1994, 841 RSC;
(e) S. Nakatsuji, Y. Amano, H. Kawamura and H. Anzai, Liebigs Ann., 1997, 729 CrossRef.
-
(a) T. Sugimoto, I. Sugimoto, A. Kawashima, Y. Yamamoto, Y. Misaki and Z. Yoshida, Heterocycles, 1987, 25, 83 CrossRef CAS;
(b) R. L. Meline and R. L. Elsenbaumer, J. Chem. Soc., Perkin Trans. 1, 1997, 3575 RSC;
(c) R. L. Meline and R. L. Elsenbaumer, Synthesis, 1997, 617 CrossRef CAS;
(d) R. L. Meline and R. L. Elsenbaumer, Synth. Met., 1997, 86, 1845 CrossRef CAS;
(e) R. L. Meline and R. L. Elsenbaumer, J. Chem. Soc., Perkin Trans. 1, 1998, 2467 RSC;
(f) R. L. Meline and R. L. Elsenbaumer, Synth. Met., 1999, 102, 1658 CrossRef CAS;
(g) J. Garín, R. Andreu, J. Orduna and J. M. Royo, Synth. Met., 2001, 120, 749 CrossRef.
-
(a) T. Ishiguro, K. Yamaji and G. Saito, Organic Superconductors, Springer, Berlin, 2nd edn, 1998 Search PubMed;
(b) J. M. Williams, J. R. Ferraro, R. J. Thorn, K. D. Carlson, U. Geiser, H. H. Wang, A. M. Kini and M. H. Whangbo, Organic Superconductors (including Fullerenes), Prentice Hall, Englewood Cliffs, NJ, 1992 Search PubMed;
(c) S. Kagoshima, K. Kanoda and T. Mori, J. Phys. Soc. Jpn., 2006, 75, 051001 CrossRef;
(d) A. Lebed, The Physics of Organic Superconductors and Conductors, Springer, New York, 2008 Search PubMed.
-
(a) M. Mas-Torrent, M. Durkut, P. Hadley, X. Ribas and C. Rovira, J. Am. Chem. Soc., 2004, 126, 984 CrossRef CAS PubMed;
(b) J. Nishida, S. Ando, J. Yamaguchi, K. Itaka, H. Koinuma, H. Tada, S. Tokito and Y. Yamashita, J. Am. Chem. Soc., 2005, 127, 10142 CrossRef PubMed;
(c) J. Nishida, D. Kumaki, S. Tokito and Y. Yamashita, J. Am. Chem. Soc., 2006, 128, 9598 CrossRef PubMed;
(d) X. Gao, Y. Wang, X. Yang, Y. Liu, W. Qiu, W. Wu, J. Zhang, T. Qi, Y. Liu, K. Lu, C. Du, Z. Shuai, G. Yu and D. Zhu, Adv. Mater., 2007, 19, 3037 CrossRef CAS;
(e) X. Gao, W. Wu, Y. Liu, S. Jiao, W. Qiu, L. Wang and D. Zhu, J. Mater. Chem., 2007, 17, 736 RSC;
(f) Y. Yamashita, Sci. Technol. Adv. Mater., 2009, 10, 024313 CrossRef;
(g) W. Wu, Y. Liu and D. Zhu, Chem. Soc. Rev., 2010, 39, 1489 RSC;
(h) M. Mas-Torrent and C. Rovira, Chem. Rev., 2011, 111, 4833 CrossRef CAS PubMed;
(i) C. Wang, H. Dong, W. Hu, Y. Liu and D. Zhu, Chem. Rev., 2012, 112, 2208 CrossRef CAS PubMed;
(j) G. Yang, C. Di, G. Zhang, J. Xiang, D. Zhang and D. Zhu, Adv. Funct. Mater., 2013, 23, 1671 CrossRef CAS.
-
(a) R. Berridge, P. J. Skabara, C. Pozo-Gonzalo, A. Kanibolotsky, J. Lohr, J. J. W. McDouall, E. J. L. McInnes, J. Wolowska, C. Winder, N. S. Sariciftci, R. W. Harrington and W. Clegg, J. Phys. Chem. B, 2006, 110, 3140 CrossRef CAS PubMed;
(b) N. Martín, L. Sánchez, M. A. Herranz, B. Illescas and D. M. Guldi, Acc. Chem. Res., 2007, 40, 1015 CrossRef PubMed , and references therein.
- There are tremendous literatures about the molecular switches as well as the related rotaxanes and catenanes using TTF as key building block, it is hard to cite all of these pioneering works. Alternately, a recent review covers most of the works about this topic, please see: D. Canavet, M. Sallé, G. Zhang, D. Zhang and D. Zhu, Chem. Commun., 2009, 2245 RSC , and references therein.
-
(a) C. F. Leong, B. Chan, T. B. Faust and D. M. D'Alessandro, Chem. Sci., 2014, 5, 4724 RSC;
(b) S. Jin, T. Sakurai, T. Kowalczyk, S. Dalapati, F. Xu, H. Wei, X. Chen, J. Gao, S. Seki, S. Irle and D. Jiang, Chem.–Eur. J., 2014, 20, 14608 CrossRef CAS PubMed;
(c) H. Ding, Y. Li, H. Hu, Y. Sun, J. Wang, C. Wang, C. Wang, G. Zhang, B. Wang, W. Xu and D. Zhang, Chem.–Eur. J., 2014, 20, 14614 CrossRef CAS PubMed;
(d) S. Cai, Y. Zhang, A. Pun, B. He, J. Yang, F. Toma, I. Sharp, O. Yaghi, J. Fan, S. Zheng, W. Zhang and Y. Liu, Chem. Sci., 2014, 5, 4693 RSC;
(e) T. C. Narayan, T. Miyakai, S. Seki and M. Dinca, J. Am. Chem. Soc., 2012, 134, 12932 CrossRef CAS PubMed;
(f) S. Bivaud, J. Y. Balandier, M. Chas, M. Allain, S. Goeb and M. Sallé, J. Am. Chem. Soc., 2012, 134, 11968 CrossRef CAS PubMed;
(g) S. Bivaud, S. Goeb, V. Croue, P. I. Dron, M. Allain and M. Sallé, J. Am. Chem. Soc., 2013, 135, 10018 CrossRef CAS PubMed;
(h) P. Huo, L. Xue, Y. Li, T. Chen, L. Yu, Q. Zhu and J. Dai, CrystEngComm, 2016, 18, 2894 RSC.
-
(a) J. Sun, X. Lu, J. Shao, Z. Cui, Y. Shao, G. Jiang, W. Yu and X. Shao, RSC Adv., 2013, 3, 10193 RSC;
(b) J. Sun, X. Lu, J. Shao, X. Li, S. Zhang, B. Wang, J. Zhao, Y. Shao, R. Fang, Z. Wang, W. Yu and X. Shao, Chem.–Eur. J., 2013, 19, 12517 CrossRef CAS PubMed;
(c) X. Lu, J. Sun, Y. Liu, J. Shao, L. Ma, S. Zhang, J. Zhao, Y. Shao, H. Zhang, Z. Wang and X. Shao, Chem.–Eur. J., 2014, 20, 9650 CrossRef CAS PubMed.
-
(a) R. Andreu, J. Garín, J. Orduna and J. M. Royo, Tetrahedron Lett., 2001, 120, 749 Search PubMed;
(b) D. G. Branzea, F. Pop, P. Auban-Senzier, R. Clerac, P. Alemany, E. Canadell and N. Avarvari, J. Am. Chem. Soc., 2016, 138, 6838 CrossRef CAS PubMed.
- CCDC registration numbers: TTN1, 1492704; TTN2, 1492705; TTN3, 1492706; TTN4, 1492707; TTN6, 1492708; TTN9, 1492710; TTF3, 1492709.
- C. Rovira, J. Veciana, N. Santaló, J. Tarrés, J. Cirujeda, E. Molins, J. Llorca and E. Espinosa, J. Org. Chem., 1994, 59, 3307 CrossRef CAS.
- A. Bondi, J. Phys. Chem., 1964, 68, 411 CrossRef.
-
(a) K. Reichenbächer, H. I. Süss and J. Hulliger, Chem. Soc. Rev., 2005, 34, 22 RSC;
(b) Y. Wang, S. R. Parkin, J. Gierschner and M. D. Watson, Org. Lett., 2008, 10, 3307 CrossRef CAS PubMed;
(c) R. Berger, G. Resnati, P. Metrangolo, E. Weber and J. Hulliger, Chem. Soc. Rev., 2011, 40, 3496 RSC;
(d) D. Chopra and T. N. Guru Row, CrystEngComm, 2011, 13, 2175 RSC.
- M. S. Kirketerp, L. A. E. Leal, D. Varsano, A. Rubio, T. J. D. Jørgensen, K. Kilså, M. B. Nielsen and S. B. Nielsen, Chem. Commun., 2011, 47, 6900 RSC.
-
(a) A. Izuoka, T. Tachikawa, T. Sugawara, Y. Suzuki, M. Konno, Y. Saito and H. Shinohara, J. Chem. Soc., Chem. Commun., 1992, 1472 RSC;
(b) A. Izuoka, T. Tachikawa, T. Sugawara, Y. Saito and H. Shinohara, Chem. Lett., 1992, 1049 CrossRef CAS;
(c) D. V. Konarev, V. N. Semkin, A. Graja and R. N. Lyubovskaya, J. Mol. Struct., 1998, 450, 11 CrossRef CAS;
(d) D. V. Konarev, R. N. Lyubovskaya, N. V. Drichko, E. I. Yudanova, Y. M. Shul'ga, A. L. Litvinov, V. N. Semkin and B. P. Tarasov, J. Mater. Chem., 2000, 10, 803 RSC;
(e) G. Saito, H. Sasaki, T. Aoki, Y. Yoshida, A. Otsuka, H. Yamochi, O. O. Drozdova, Y. Yakushi, H. Kitagawa and T. Mitani, J. Mater. Chem., 2002, 12, 1640 RSC;
(f) D. V. Konarev, S. S. Khasanov, G. Saito, A. Otsuka and R. N. Lyubovskaya, J. Mater. Chem., 2007, 17, 4171 RSC.
- For the complexes of the extended-TTF with fullerenes, see:
(a) E. M. Pérez, L. Sánchez, G. Fernández and N. Martín, J. Am. Chem. Soc., 2006, 128, 7172 CrossRef PubMed;
(b) K. A. Nielsen, W.-S. Cho, G. H. Sarova, B. M. Petersen, A. D. Bond, J. Becher, F. Jensen, D. M. Guldi, J. L. Sessler and J. O. Jeppesen, Angew. Chem., Int. Ed., 2006, 45, 6848 CrossRef CAS PubMed;
(c) E. M. Pérez, M. Sierra, L. Sánchez, M. R. Torres, R. Viruela, P. M. Viruela, E. Ortí and N. Martín, Angew. Chem., Int. Ed., 2007, 46, 1847 CrossRef PubMed;
(d) G. Fernández, L. Sánchez, E. M. Pérez and N. Martín, J. Am. Chem. Soc., 2008, 130, 10674 CrossRef PubMed;
(e) H. Isla, M. Gallego, E. M. Pérez, R. Viruela, E. Ortí and N. Martín, J. Am. Chem. Soc., 2010, 132, 1772 CrossRef CAS PubMed;
(f) E. Huerta, H. Isla, E. M. Pérez, C. Bo, N. Martín and J. de Mendoza, J. Am. Chem. Soc., 2010, 132, 5351 CrossRef CAS PubMed;
(g) B. Grimm, J. Santos, B. M. Illescas, A. Muñoz, D. M. Guldi and N. Martín, J. Am. Chem. Soc., 2010, 132, 17387 CrossRef CAS PubMed;
(h) D. Canevet, M. Gallego, H. Isla, A. de Juan, E. M. Pérez and N. Martín, J. Am. Chem. Soc., 2011, 133, 3184 CrossRef CAS PubMed;
(i) S. Fukuzumi, K. Ohkubo, Y. Kawashima, D. S. Kim, J. S. Park, A. Jana, V. M. Lynch, D. Kim and J. L. Sessler, J. Am. Chem. Soc., 2011, 133, 15938 CrossRef CAS PubMed;
(j) Z. Zhou, Y. Qin, W. Xu and D. Zhu, Chem. Commun., 2014, 50, 4082 RSC.
-
(a) J. Sun, X. Lu, M. Ishikawa, Y. Nakano, S. Zhang, J. Zhao, Y. Shao, Z. Wang, H. Yamochi and X. Shao, J. Mater. Chem. C, 2014, 2, 8017 RSC;
(b) X. Lu, J. Sun, S. Zhang, L. Ma, L. Liu, H. Qi, Y. Shao and X. Shao, Beilstein J. Org. Chem., 2015, 11, 1043 CrossRef CAS PubMed;
(c) S. Zhang, X. Lu, J. Sun, Y. Zhao and X. Shao, CrystEngComm, 2015, 17, 4110 RSC.
- CCDC registration numbers: (TTN3)·(C60)·(CS2), 1492711; (TTN3)·(C60), 1492712; (TTN3)·(C70)·(CS2), 1492713; (TTN3)·(C70), 1492714; (TTN2)·(C60), 1492715; (TTN6)·(C60), 1492716.
-
(a) M. C. Burla, R. Caliandro, M. Camalli, B. Carrozzini, G. L. Cascarano, L. D. Caro, C. Giacovazzo, G. Polidori and R. Spagna, J. Appl. Crystallogr., 2005, 38, 381 CrossRef CAS;
(b) G. M. Sheldrick, SHELXL-97, A Program for Crystal Structure Refinement, University of Göttingen, Göttingen, 1997, Release 97–2 Search PubMed.
-
(a) M. J. Frisch, G. W. Trucks, H. B. Schlegel, G. E. Scuseria, M. A. Robb, J. R. Cheeseman, G. Scalmani, V. Barone, B. Mennucci, G. A. Petersson, H. Nakatsuji, M. Caricato, X. Li, H. P. Hratchian, A. F. Izmaylov, J. Bloino, G. Zheng, J. L. Sonnenberg, M. Hada, M. Ehara, K. Toyota, R. Fukuda, J. Hasegawa, M. Ishida, T. Nakajima, Y. Honda, O. Kitao, H. Nakai, T. Vreven, J. A. Montgomery, Jr, J. E. Peralta, F. Ogliaro, M. Bearpark, J. J. Heyd, E. Brothers, K. N. Kudin, V. N. Staroverov, R. Kobayashi, J. Normand, K. Raghavachari, A. Rendell, J. C. Burant, S. S. Iyengar, J. Tomasi, M. Cossi, N. Rega, J. M. Millam, M. Klene, J. E. Knox, J. B. Cross, V. Bakken, C. Adamo, J. Jaramillo, R. Gomperts, R. E. Stratmann, O. Yazyev, A. J. Austin, R. Cammi, C. Pomelli, J. W. Ochterski, R. L. Martin, K. Morokuma, V. G. Zakrzewski, G. A. Voth, P. Salvador, J. J. Dannenberg, S. Dapprich, A. D. Daniels, Ö. Farkas, J. B. Foresman, J. V. Ortiz, J. Cioslowski and D. J. Fox, Gaussian 09, revision A.01, Gaussian, Inc., Wallingford CT, 2009 Search PubMed;
(b) A. D. Becke, J. Chem. Phys., 1993, 98, 5648 CrossRef CAS;
(c) C. Lee, W. Yang and R. G. Parr, Phys. Rev. B: Condens. Matter Mater. Phys., 1988, 37, 785 CrossRef CAS;
(d) V. A. Rassolov, M. A. Ratner, J. A. Pople, P. C. Redfern and L. A. Curtiss, J. Comput. Chem., 2001, 22, 976 CrossRef CAS.
Footnote |
† Electronic supplementary information (ESI) available: Characterization of the compounds, theoretical calculation, additional optical and electrochemical spectra, crystal structures. CCDC 1492704–1492716. For ESI and crystallographic data in CIF or other electronic format see DOI: 10.1039/c6ra18945h |
|
This journal is © The Royal Society of Chemistry 2016 |
Click here to see how this site uses Cookies. View our privacy policy here.