DOI:
10.1039/C6RA18928H
(Paper)
RSC Adv., 2016,
6, 105644-105652
Structure evolution of Prussian blue analogues to CoFe@C core–shell nanocomposites with good microwave absorbing performances
Received
26th July 2016
, Accepted 22nd October 2016
First published on 24th October 2016
Abstract
Novel uniform CoFe@C core–shell composite nanoparticles with good distribution have been fabricated through the combined self-assembly and controlled thermal decomposition of Co-based Prussian blue (PB) nanocubes. Good intrinsic magnetic properties including a high saturation magnetization of 90.9–182.5 A m2 kg−1 and a high coercivity of 192–510 Oe are achieved for these CoFe@C nanocomposites. Excellent wave absorbing properties, including a minimum reflection loss value of −43.5 dB at 9.92 GHz with a sample thickness of 2.5 mm and an effective absorption bandwidth of 4.3 GHz (below −10 dB), are obtained for the CoFe@C nanocomposites, which can be ascribed to the good magnetic properties originating from CoFe as the core and the good electrical conductivity from the graphite carbon layers. These CoFe@C nanocomposites also show good structural stability and low density, which further increases their potential for application as high-performance electromagnetic wave-absorbing materials.
1. Introduction
Electromagnetic (EM) wave absorption materials have attracted much attention due to their wide use in various fields such as the EM industry, information security and biological systems, as an efficient solution for EM interference, stealth, and environmental and health problems.1,2 In general, high-efficiency EM wave absorption materials need to satisfy the requirements of having a low weight, thin thickness, strong absorption, high thermal stability and a wide frequency band. Over the past decade, many kinds of materials such as metals (Fe, Co and Ni),3,4 magnetic particles (Fe2O3, Co3O4 and CoFe2O4),5–7 carbon materials (porous carbon, CNTs, CNF and graphene)8–11 and conducting polymers (PANI and PPY)12,13 have been developed to be effective EM wave absorption materials. However, these materials show one major demerit in actual application, their poor impedance matching of dielectric and magnetic loss,14 which is also a major problem to be resolved for high-performance EM wave absorption materials.
Carbon-based magnetic composites can achieve outstanding wave absorbing properties by combining their magnetic and dielectric components, which gives the advantages of increasing the magnetic and dielectric loss, broadening the absorption bandwidth and significantly reducing the weight for these materials.15,16 It is worth noting that these carbon-based composites also exhibit porous or core–shell microstructures, and possess better physical and chemical properties, such as good dielectric properties and a high large specific surface area compared with their single component counterparts,17,18 which are promising for a potential high-performance EM wave absorption material. Thus it is necessary to develop simple and effective preparation methods for fabricating novel carbon-based magnetic composites with controlled composite structures.
Recently, metal–organic frameworks (MOFs) exhibiting regular morphologies, high surface area and good dielectric properties have been assembled by the combination of metal ions with bridging organic ligands, which have proven to be the ideal templates for fabricating various functional materials.19,20 The most attractive aspect is that the materials derived from MOFs possess many different kinds of compositions such as metals, metal oxides, carbon or their hybrids, and it is possible to balance their dielectric permittivity and magnetic permeability through adjusting their compositions to a suitable proportion. As a typical class of MOFs, Prussian blue (PB) nanocubes can be unexpectedly used as the precursors or templates to develop new functional nanomaterials with porous or core–shell nanostructures for EM wave absorption.21 To the best of our knowledge, CoFe@C magnetic composites derived from PB nanocubes and used for EM wave absorption materials have not previously been reported, which may be mainly due to the difficulty in finding appropriate templates or effective preparation methods.
In this work, we have reported a facile template-engaged approach to synthesize CoFe@C core–shell nanocomposites through the combined self-assembly and controlled thermal decomposition of Co-based PB nanocubes. The as-synthesized CoFe@C core–shell nanocomposites exhibit uniformly dispersed nanoparticles with CoFe as the core and graphite carbon as the shell, which leads to their good intrinsic magnetic properties and excellent wave absorbing performances. The microstructures, formation mechanisms and wave absorbing properties of these CoFe@C core–shell nanocomposites are also systematically studied.
2. Experimental section
2.1 Materials
Cobalt chloride hexahydrate (CoCl2·6H2O), sodium citrate, potassium ferricyanide (K3[Fe(CN)6]) and ethanol were used as raw materials and purchased from Sinopharm Chemical Reagent Co., Ltd. All of the reagents in the experiment were of analytical grade and used directly without further purification.
2.2 Synthesis
A schematic preparation process for the Co-based PB nanocubes and the CoFe@C nanocomposites derived from them is shown in Fig. 1. Firstly, the Co-based PB precursors were synthesized by a facile precipitation of CoCl2 and K3[Fe(CN)6]. Typically, a suitable amount of aqueous solution (20 mL) containing CoCl2·6H2O (143 mg) and sodium citrate (387 mg) was mixed with another aqueous solution (20 mL) containing K3[Fe(CN)6] (132 mg) under magnetic stirring for 10 min. Then, the above solution mixture was sealed and left to stand for 24 h at room temperature. The resulting precipitates were collected by centrifugation, washed with distilled water and ethanol several times and dried in a vacuum oven at 40 °C for 24 h. The CoFe@C nanocomposites were fabricated by controlled thermal decomposition using the above freshly prepared Co-based PB precursors as starting materials. Briefly, a certain amount of the as-synthesized Co-based PB precursors was loaded into a combustion boat which was then put in a tubular furnace. Next, the Co-based PB precursors were firstly heated from room temperature to 200 °C at a heating rate of 2 °C min−1 in the tubular furnace under a high-purity N2 atmosphere and then heated from 200 °C to the final temperature (550 °C, 650 °C or 750 °C) at a heating rate of 1 °C min−1. The final product CoFe@C nanocomposites were collected after naturally cooling the decomposed precursors.
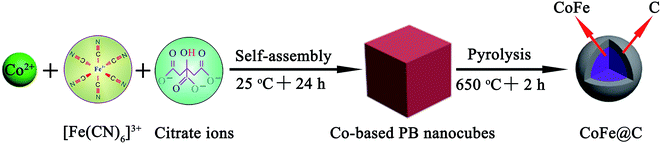 |
| Fig. 1 Schematic formation process for the CoFe@C nanocomposites. | |
2.3 Characterization
The crystal structure of the samples was analyzed using an X-ray diffractometer (XRD, Rigaku D/max 2500PC) with Cu Kα radiation (λ = 1.54 Å, 40 kV, 150 mA). A field emission scanning electron microscope (FESEM, JSM-7500F, JEOL) and a transmission electron microscope (TEM, JEM-2100, JEOL) equipped with an energy dispersive X-ray spectroscope (EDS) were used to investigate the morphologies, microstructures and compositions of the samples. The surface states of the samples were analysed using an X-ray photoelectron spectroscope (XPS, ESCALAB 250 Xi) with an Al Kα excitation source. The room-temperature intrinsic magnetic properties of these samples were measured with a vibrating sample magnetometer (VSM, Lakeshore 7307) under a maximum magnetic field of 10 kOe.
2.4 EM wave absorption measurements
The EM wave absorption measurements were conducted with an Agilent N5230C network analyzer in the range of 2–18 GHz. The measured samples were prepared as follows. Firstly, a mixture consisting of 50 wt% of the final products and 50 wt% of paraffin wax was mixed well to form a uniform composite. The above mixture was then compressed into toroidal shapes (Φin = 3 mm and Φout = 7 mm) with thicknesses of 2.5–3.5 mm. The reflection loss (RL) values are calculated from the measured εr and μr at the given frequency and the absorber thicknesses according to the transmission line theory using the following equations:22 |
Zin = Z0(μr/εr)1/2 tanh[j(2πft/c)(εrμr)1/2]
| (1) |
|
RL = 20 log(Zin − Z0)/(Zin + Z0)
| (2) |
where Zin is the input impedance of the absorber, Z0 is the free-space impedance, εr and μr are the relative complex permittivity and permeability, respectively, f is the frequency of the EM wave, t is the absorber thickness, and c is the velocity of light.
3. Results and discussion
3.1 Characterization of the samples
The XRD patterns of the Co-based PB nanocubes and the annealed ones at the different temperatures of 550 °C, 650 °C and 750 °C are shown in Fig. 2a. The sharp diffraction peaks for the Co-based PB nanocubes show that the precursors are well crystallized and all of the peaks can be indexed to the Co3[Fe(CN)6]2·H2O phase, indicating the high purity of the precursor. However, all the annealed samples exhibit a very different XRD pattern with three sharp peaks at 2θ values of about 44.8°, 65.2° and 82.6°, which can be indexed to the crystal planes of (110), (200) and (211) for the body-centered-cubic CoFe phase (JCPDS 44-1433). In addition, an obvious broad peak at a 2θ value of about 26° for the three annealed samples can be assigned to graphite carbon.23,24 Furthermore, the XPS spectrum of C 1s for the CoFe@C nanocomposites prepared at 650 °C shown in Fig. 2b shows a characteristic peak at 284.5 eV, which corresponds to the sp2-hybridisation state of graphite carbon.25 As discussed later, this graphite carbon acts as a shell coating on the surface of the CoFe nanoparticles to form a CoFe@C core–shell composite structure, which can be confirmed by the following HRTEM measurements. These conductive carbon layers would lead to good electrical conductivity for CoFe@C nanocomposites and thus result in their enhanced dielectric properties. The appearance of both magnetic and dielectric components in these CoFe@C nanocomposites may lead to their high dielectric and magnetic losses so as to result in their good wave absorbing properties, which are discussed later.
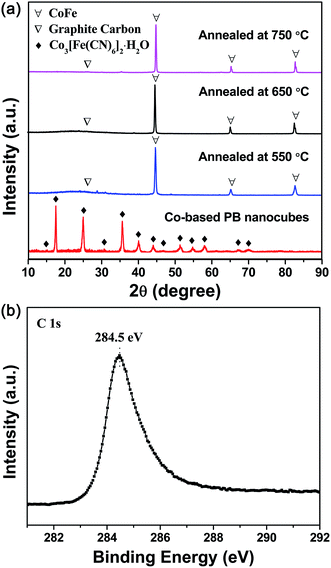 |
| Fig. 2 The XRD patterns of the products (a) and the C 1s XPS spectrum of the CoFe@C nanocomposites (b). | |
Fig. 3 shows the representative SEM images of the Co-based PB precursors and the CoFe@C nanocomposites prepared at 550 °C, 650 °C and 750 °C. It can be seen that the precursors show uniform cube-shaped nanoparticles with a smooth surface and a particle size of about 150 nm (Fig. 3a), which indicates that Co-based PB nanocubes can be self-assembled by reacting Co2+ ions with cyanide ions through a controlled precipitation method. As shown in Fig. 3b and c, these well-structured Co-based PB nanocubes can be converted into homogeneously dispersed CoFe@C composite nanoparticles with a nearly spherical morphology after suitable thermal decomposition. Furthermore, the particle size of the CoFe@C nanocomposites increases from 100 nm to 700 nm with increasing the annealing temperature from 650 °C to 750 °C. Moreover, asymmetric agglomerated particles are also obtained in the CoFe@C nanocomposites prepared at 750 °C, which would bring about their lower wave absorbing properties, which are discussed below.
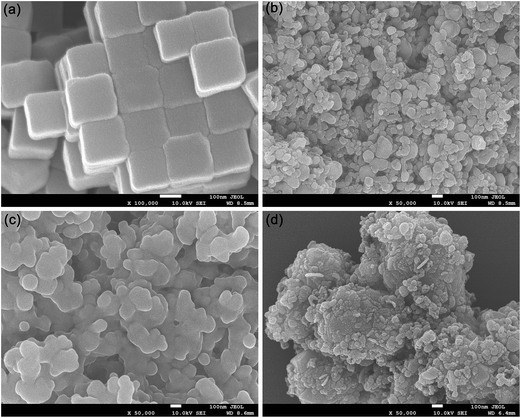 |
| Fig. 3 The SEM images of the Co-based PB nanocubes (a) and the CoFe@C nanocomposites prepared at 550 °C (b), 650 °C (c) and 750 °C (d). | |
TEM measurement has been used to further investigate the core–shell structure of the CoFe@C nanocomposites. Fig. 4 shows the representative TEM images of the Co-based PB nanocubes and the CoFe@C nanocomposites prepared at 550 °C and 650 °C. The uniform cube-shaped morphologies for the precursors in Fig. 4a are consistent with the above SEM observation. It can be clearly seen from Fig. 4b and c that the CoFe@C nanocomposites exhibit a core–shell composite structure with CoFe nanoparticles as the core and graphite carbon layers as the shell. The thickness of the graphite carbon layers coated on the surface of the CoFe nanoparticles is estimated to be 20–50 nm. Furthermore, the thickness of the graphite carbon layers for the CoFe@C nanocomposites will be slightly increased with increasing the thermal-decomposition temperature from 550 °C to 650 °C. The formation of the graphite carbon deposited on the surface of the CoFe nanoparticles should be attributed to the catalytic effects of metals.26 The mass fraction ratio of CoFe and the carbon phase, calculated by EDS measurement, in the CoFe@C nanocomposites prepared at 550 °C and 650 °C is 74.3
:
25.7 and 68.4
:
31.6, respectively. It can be found that the microstructures of the CoFe@C nanocomposites, including the particle size and the carbon shell thickness, can be tuned effectively by varying the thermal-decomposition temperature, which may facilitate the occurrence of good impedance matching in these novel carbon-based nanocomposites so as to obtain excellent wave absorbing properties. The representative HRTEM image shown in Fig. 4d for the CoFe@C nanocomposites presents an obvious interfacial structure of the graphite carbon and CoFe phases, the lattice interplanar spacing of which can be measured to be 0.34 nm and 0.2 nm, respectively, and matches very well with the distances of the (002) and (110) planes for these two phases, respectively.27,28 Furthermore, the SAED patterns shown in Fig. 4h reveal three obvious diffractions, which accord well with those for the (002), (100) and (110) crystal planes of the graphite phase, respectively.27 Some regularly scattered diffraction dots can be indexed to the single-crystal CoFe phase. Fig. 4e–g show the representative elemental mapping of the spatial distribution of the Co and Fe elements in the CoFe@C nanocomposites prepared at 650 °C. There is a uniform distribution of the Co and Fe elements for the CoFe phase as the core part, which further confirms the perfect core–shell structure in the CoFe nanocomposites.
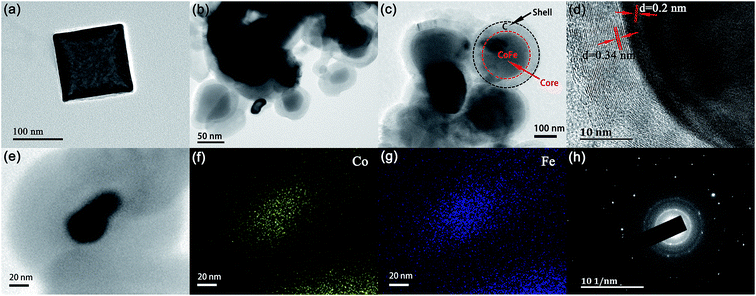 |
| Fig. 4 The TEM images of the Co-based PB nanocubes (a) and the CoFe@C nanocomposites prepared at 550 °C (b) and 650 °C (c), HRTEM images of the CoFe@C nanocomposites (d), and the elemental mapping of Co (f) and Fe (g) elements from the TEM image (e) and the SAED pattern for the CoFe@C nanocomposites (h). | |
3.2 Formation mechanism of the CoFe@C nanocomposites
Based on the morphologies and microstructures of the CoFe@C nanocomposites, the main reactions for the formation process of the samples can be expressed as follows. |
3Co2+ + 2[Fe(CN)6]3− → Co3[Fe(CN)6]2
| (3) |
|
Co3[Fe(CN)6]2 → CoFe + C + N2
| (4) |
As can be seen from eqn (3), the [Fe(CN)6]3− ions show a strong tendency to coordinate with Co2+ to form stable Co3[Fe(CN)6]2 nuclei. It can be found in the experimental section of the work of Hu et al. (ref. 28) that sodium citrate addition would decrease and postpone the nucleation rate of Co3[Fe(CN)6]2 so as to control the crystal growth, which may result in the formation of monodispersed Co3[Fe(CN)6]2 nanocubes. Then, the Co-based PB nanocubes were decomposed at high temperature under N2 atmosphere. In this thermal decomposition process, the organic components of the precursors can be directly carbonized and the metal ions Co2+ and Fe2+ are simultaneously reduced in situ to become high-purity CoFe alloys (eqn (4)) resulting in the formation of CoFe@C hybrids, a similar process to which can be observed in previous work.18,29 It should be noted that the formation of the graphite carbon layers can be attributed to the catalytic effects of metal ions.30 It has been reported that the metal/carbon hybrid as a unique heterostructure may exhibit a good EM matching through adjusting its composite structure in terms of its nanoscale geometry.31 The higher decomposition temperature of the CoFe@C nanocomposites leads to their larger particle size, which can be ascribed to the quicker grain growth of the CoFe@C nanoparticles resulting from the rapid decomposition of the precursor at high temperature.
3.3 Intrinsic magnetic properties of the CoFe@C nanocomposites
Fig. 5 shows the room-temperature hysteresis loops of the Co-based PB nanocubes and the CoFe@C nanocomposites prepared at 550 °C, 650 °C and 750 °C. It can be seen from Fig. 5a that the Co-based PB nanocube samples exhibit almost nonmagnetism due to their MOF-based materials. However, the CoFe@C nanocomposites converted from the Co-based PB nanocubes show typical ferromagnetic behavior mainly due to the existence of the magnetic CoFe phase. The CoFe@C nanocomposites prepared at 550 °C exhibit a very high coercivity (iHc) of 510 Oe along with a saturation magnetization (Ms) of 90.9 A m2 kg−1. Moreover, much higher Ms values of 116.6 A m2 kg−1 and 182.5 A m2 kg−1 and relatively lower iHc values of 296.6 Oe and 192 Oe are obtained in the CoFe@C nanocomposites prepared at 650 °C and 750 °C, respectively, which can probably be ascribed to their larger CoFe grains due to the higher thermal-decomposition temperature. Compared with the intrinsic magnetic properties of bulk bcc-CoFe alloys (iHc of about 10 Oe and Ms of about 235 A m2 kg−1),32 the higher iHc values and relatively lower Ms for these CoFe@C nanocomposites may be ascribed to their nanoparticles and the nonmagnetism of the graphite carbon layers along with the possible surface spin effects for ferromagnetic CoFe phases in the composites.33,34 The good intrinsic magnetic properties, including a very high iHc value and a high Ms value, for the CoFe@C nanocomposites would result in their high magnetic loss, which is favorable for enhancing their EM wave absorption performances, which are discussed below.
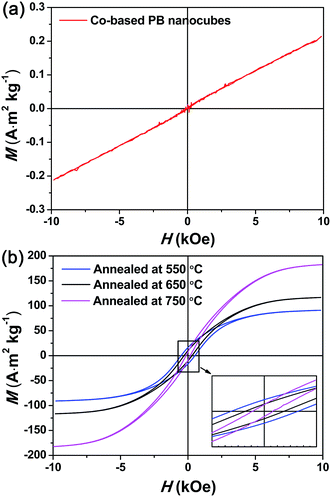 |
| Fig. 5 The hysteresis loops of the Co-based PB nanocubes (a) and the CoFe@C nanocomposites (b) prepared at 550 °C, 650 °C and 750 °C. | |
3.4 Wave absorbing properties of the CoFe@C nanocomposites
The Co-based PB nanocubes show almost no wave absorbing properties just because of their nonmagnetism. However, enhanced wave absorbing properties are observed in the CoFe@C core–shell nanocomposites. Fig. 6 shows the two-dimensional (2D) and three-dimensional (3D) RL of the CoFe@C nanocomposites prepared at 550 °C, 650 °C and 750 °C. As shown in Fig. 6a, the minimum RL value for the CoFe@C nanocomposites prepared at 550 °C is −18 dB with a sample thickness of 2.5 mm. Moreover, from Fig. 6c it can be seen that much better wave absorbing properties, including a minimum RL value of −43.5 dB at 9.92 GHz with a sample thickness of 2.5 mm and an effective absorption bandwidth of 4.3 GHz (below −10 dB), are obtained for the CoFe@C nanocomposites prepared at 650 °C. The greatly enhanced wave absorbing properties are superior to those of most of the carbon-based composites reported in the literature listed in Table 1. The application scope for the samples with good wave absorbing properties including the detailed sample thickness along with the accurate applied frequency can be demonstrated in their 3D RL images shown in Fig. 6b, d and e, which can promote and guide their actual application. It can be seen that RL values exceeding −10 dB can be achieved in a wide frequency range of 10–18 GHz for the CoFe@C nanocomposites prepared at 550 °C (Fig. 6b). However, the corresponding sample thickness is about 4–10 mm (below −10 dB), which may need to be thinned for their practical application. Notably, for the CoFe@C nanocomposites prepared at 650 °C, their RL values exceeding −10 dB in a wide frequency range of 4–18 GHz can be achieved with a sample thickness of 0–10 mm. Moreover, a minimum RL value of −43.5 dB is obtained at 9.92 GHz with a sample thickness of 2.5 mm, which is much better than that of the sample with the same thickness prepared at 550 °C (minimum RL value of −18 dB). Relatively lower wave absorbing properties with a minimum RL value of −30.1 dB at 4.56 GHz with a sample thickness of 5 mm are obtained in the composites prepared at 750 °C due to their agglomerated structure.
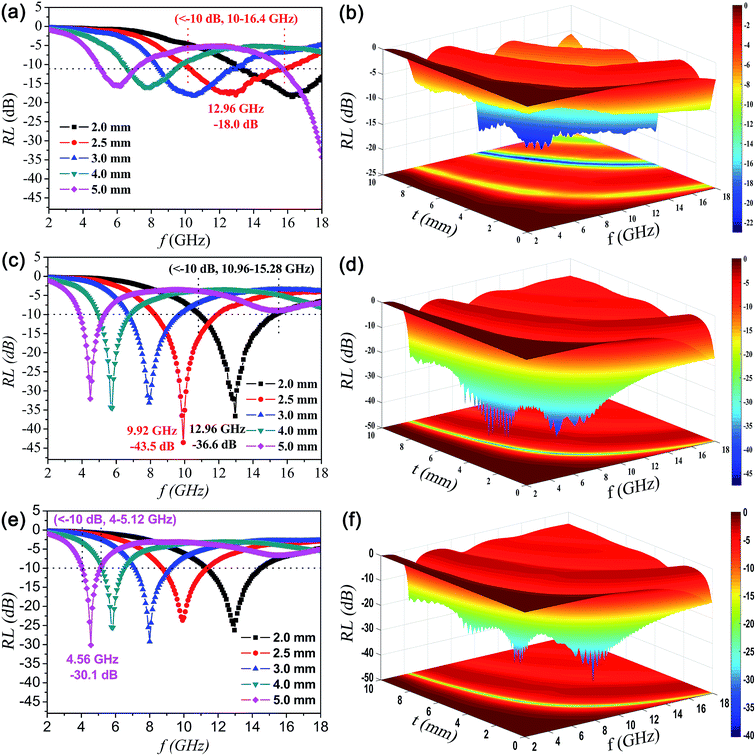 |
| Fig. 6 The two-dimensional and three-dimensional RL values of the CoFe@C nanocomposites prepared at 550 °C (a and b), 650 °C (c and d) and 750 °C (e and f). | |
Table 1 The microwave absorbing properties of some carbon-based composites reported in the literature
Sample |
Weight fraction (wt%) |
RLmin (dB) |
Sample thickness (mm) |
Bandwidth (<−10 dB) (GHz) |
Ref. |
FeNi@C |
40 |
−26.9 |
2.0 |
5.6 (12.4–18.0) |
35 |
CoNi@C |
40 |
−35.0 |
2.0 |
6 (12.0–18.0) |
36 |
Ni@C |
40 |
−32.0 |
2.0 |
4.3 (11.2–15.5) |
37 |
Fe–C |
50 |
−36.0 |
3.0 |
1.2 (3.6–4.8) |
38 |
Co/C |
40 |
−35.3 |
2.5 |
5.8 (8.4–14.2) |
18 |
FeCo/C |
50 |
−40.2 |
2.5 |
3.6 (8.2–11.8) |
39 |
Fe–Co/C |
50 |
−21.7 |
1.2 |
5.8 (12.2–18.0) |
40 |
CoFe@C |
50 |
−43.5 |
2.5 |
4.3 (11.0–15.3) |
This work |
To further investigate the mechanism of the good wave absorbing properties for these CoFe@C nanocomposites, the frequency-dependent complex permittivity (εr = ε′ − jε′′) and permeability (μr = μ′ − jμ′′) curves at a frequency range of 2–18 GHz for the two representative samples prepared at 550 °C and 650 °C have been analyzed as shown in Fig. 7. As is known, the real parts μ′ and ε′ and the imaginary parts μ′′ and ε′′ for the absorbing materials represent their storage and loss capability of EM wave energy, respectively.41 Therefore, the occurrence of large εr and μr especially with increased μ′′ and ε′′ values for the absorbing materials would increase greatly their EM wave absorbing ability.42 As shown in Fig. 7a and b, several magnetic resonance peaks can be observed in the frequency-dependent μ′ and μ′′ curves for the two samples, indicating the contribution of the magnetic resonance loss to their EM wave absorptions due to ferromagnetic CoFe alloys in the composites. However, the similar magnetic loss tan
δμ shown in Fig. 7e for the samples probably contributes equally to their EM wave attenuation. Thus, the dielectric loss occurring in these CoFe@C nanocomposites discussed below may dominate the total loss in their EM wave absorption measurements.
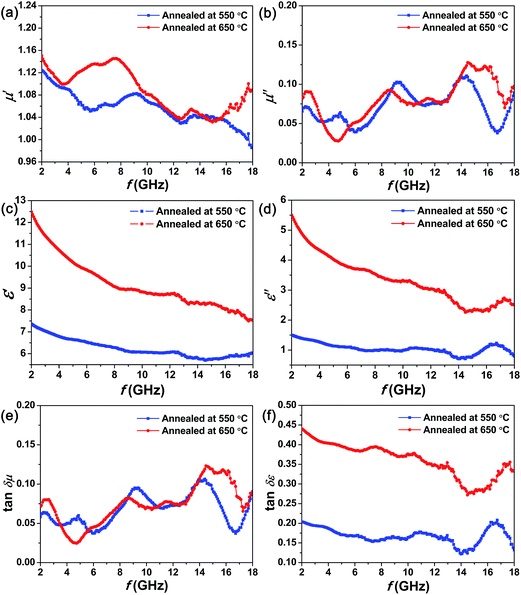 |
| Fig. 7 The relative εr (a and b), relative μr (c and d), magnetic loss tan δμ (e) and dielectric loss tan δε (f) curves of the CoFe@C nanocomposites prepared at 550 °C and 650 °C. | |
As shown in Fig. 7c and d, the two CoFe@C nanocomposites exhibit enhanced dielectric properties, which can be ascribed to the interfacial polarization and associated relaxation in the interfaces between the CoFe cores and carbon layers. Moreover, both the values of ε′ and ε′′ in the εr curves for the two composites decrease rapidly with increasing the applied frequency, which is believed to correlate with the frequency-dependent dielectric dispersion behavior occurring in most carbon-based composites.43 Furthermore, the CoFe@C nanocomposites prepared at 650 °C show larger ε′′ values and higher dielectric loss tan
δε (Fig. 7f), which can be ascribed to their increased electric polarization in the presence of thicker graphite carbon layers with higher conductivity.14 The higher dielectric loss in this sample indicates greater contribution to their EM wave attenuation. As discussed above, the composites prepared at 650 °C possess thicker graphite carbon layers, which is believed to increase their dielectric constant and promote the occurrence of several stronger dielectric polarizations including electronic, ionic and molecular polarization along with interfacial polarization decay.44 Thus, greater dielectric loss will occur in CoFe@C nanocomposites prepared at 650 °C so as to enhance their wave absorbing properties. Besides the inner dielectric loss, the much thicker graphite carbon layer as the shell can buffer the impedance difference between the air and absorbing materials due to its good electrical conductivity, which can help the accession of EM waves into the internal absorbing materials and thus enhance their wave-absorbing properties. In summary, it can be concluded that the excellent wave absorbing properties can be ascribed to the synergistic effects of the good intrinsic magnetic properties arising from the CoFe core and the high electrical conductivity of the graphite carbon layers with suitable thickness.
4. Conclusions
The Co-based PB precursors have been self-assembled by mixing Co2+ ions with cyanide ions in aqueous solution through a facile precipitation method and then in situ converted to novel CoFe@C nanocomposites by suitable thermal decomposition. The CoFe@C nanocomposites show a typical core–shell composite structure with CoFe as the core and graphite carbon as the shell, which leads to their high electrical conductivity and good intrinsic magnetic properties including a high coercivity of 192–510 Oe along with a saturation magnetization of 90.9–182.5 A m2 kg−1. The excellent wave absorbing properties including the minimum RL value of −43.5 dB at 9.92 GHz with a sample thickness of 2.5 mm and an effective absorption bandwidth of 4.3 GHz (below −10 dB) are obtained in the CoFe@C nanocomposites prepared at 650 °C, which can be ascribed to the synergistic effects of the good magnetic properties of their CoFe cores and the high electrical conductivity of their graphite carbon shells.
Acknowledgements
This work was supported by Beijing Natural Science Foundation under Grant no. 2132039 and the National Natural Science Foundation of China under Grant no. 51101007, 51171007 and 51271009.
References
- H. Wu, G. Wu, Y. Ren, L. Yang, L. Wang and X. Li, J. Mater. Chem. C, 2015, 3, 7677–7690 RSC.
- S. Zhang, Q. Jiao, Y. Zhao, H. Li and Q. Wu, J. Mater. Chem. A, 2014, 2, 18033–18039 CAS.
- Y. Y. Ren, H. F. Li, G. L. Wu, L. Yang, C. H. Zheng, L. D. Wang, F. Ren, H. Xing and H. J. Wu, Nano, 2016, 11, 1650014–1650024 CrossRef CAS.
- H. Zhao, Z. Zhu, C. Xiong, X. Zheng and Q. Lin, RSC Adv., 2016, 6, 16413–16418 RSC.
- H. C. Yu, L. C. Hsu, T. H. Chang and Y. Y. Li, Dalton Trans., 2012, 41, 723–726 RSC.
- X. B. Li, S. W. Yang, J. Sun, P. He, X. P. Pu and G. Q. Ding, Synth. Met., 2014, 194, 52–58 CrossRef CAS.
- M. Fu, Q. Jiao, Y. Zhao and H. Li, J. Mater. Chem. A, 2014, 2, 735–744 CAS.
- W. L. Song, M. S. Cao, L. Z. Fan, M. M. Lu, Y. Li, C. Y. Wang and H. F. Ju, Carbon, 2014, 77, 130–142 CrossRef CAS.
- H. Hekmatara, M. Seifi, K. Forooraghi and S. Mirzaee, Phys. Chem. Chem. Phys., 2014, 16, 24069–24075 RSC.
- G. Li, T. S. Xie, S. L. Yang, J. H. Jin and J. M. Jiang, J. Phys. Chem. C, 2012, 116, 9196–9201 CAS.
- B. Wen, M. S. Cao, M. M. Lu, W. Q. Cao, H. L. Shi, J. Liu, X. X. Wang, H. B. Jin, X. Y. Fang, W. Z. Wang and J. Yuan, Adv. Mater., 2014, 26, 3484–3489 CrossRef CAS PubMed.
- J. Zhao, J. Lin, J. Xiao and H. Fan, RSC Adv., 2015, 5, 19345–19352 RSC.
- S. Varshney, A. Ohlan, V. K. Jain, V. P. Dutta and S. K. Dhawan, Ind. Eng. Chem. Res., 2014, 53, 14282–14290 CrossRef CAS.
- R. C. Che, L. M. Peng, X. F. Duan, Q. Chen and X. L. Liang, Adv. Mater., 2004, 16, 401–405 CrossRef CAS.
- W. C. Ye, J. J. Fu, Q. Wang, C. M. Wang and D. S. Xue, J. Magn. Magn. Mater., 2015, 395, 147–151 CrossRef CAS.
- L. L. Zhang, X. X. Yu, H. R. Hu, Y. Li, M. Z. Wu, Z. Z. Wang, G. Li, Z. Q. Sun and C. L. Chen, Sci. Rep., 2015 DOI:10.1038/srep09298.
- Y. C. Du, W. W. Liu, R. Qiang, Y. Wang, X. J. Han, J. Ma and P. Xu, ACS Appl. Mater. Interfaces, 2014, 6, 12997–13006 CAS.
- Y. Y. Lu, Y. T. Wang, H. L. Li, Y. Lin, Z. Y. Jiang, Z. X. Xie, Q. Kuang and L. S. Zhe, ACS Appl. Mater. Interfaces, 2015, 7, 13604–13611 CAS.
- X. H. Cao, B. Zheng, X. H. Rui, W. H. Shi, Q. Y. Yan and H. Zhang, Angew. Chem., Int. Ed., 2014, 53, 1404–1409 CrossRef CAS PubMed.
- J. K. Sun and Q. Xu, Energy Environ. Sci., 2014, 7, 2071–2100 CAS.
- R. Qiang, Y. C. Du, H. T. Zhao, Y. Wang, C. H. Tian, Z. G. Li, X. J. Han and P. Xu, J. Mater. Chem. A, 2015, 3, 13426–13434 CAS.
- T. Maeda, S. Sugimoto, T. Kagotani, N. Tezuka and K. Inomata, J. Magn. Magn. Mater., 2004, 281, 195–205 CrossRef CAS.
- N. J. Welham and J. S. Williams, Carbon, 1998, 36, 1309–1315 CrossRef CAS.
- M. A. Vieira, G. R. Goncalves, D. F. Cipriano, M. A. Schettino Jr, E. A. Silva Filho, A. G. Gunha, F. G. Emmerich and J. C. C. Freitas, Carbon, 2016, 98, 496–503 CrossRef CAS.
- L. P. Zheng, H. Zhang, P. F. Cheng, Q. Ma, J. J. Liu, J. Nie, W. F. Feng and Z. B. Zhou, Electrochim. Acta, 2016, 196, 169–188 CrossRef CAS.
- I. Martin-Gullon, J. Vera, J. A. Conesa, J. L. Gonzalez and C. Merino, Carbon, 2006, 44, 1572–1580 CrossRef CAS.
- G. X. Xin, Y. H. Wang, J. B. Zang, S. P. Jia, P. F. Tian and S. Y. Zhou, Carbon, 2016, 98, 221–224 CrossRef CAS.
- M. Hu, S. Ishihara, K. Ariga, M. Imura and Y. Yamauchi, Chem.–Eur. J., 2013, 19, 1882–1885 CrossRef CAS PubMed.
- S. L. Guo, L. D. Wang and H. J. Wu, Adv. Powder Technol., 2015, 26, 1250–1255 CrossRef CAS.
- J. Xiang, J. Li, X. Zhang, Q. Ye, J. Xu and X. Shen, J. Mater. Chem. A, 2014, 2, 16905–16914 CAS.
- R. C. Che, C. Y. Zhi, C. Y. Liang and X. G. Zhou, Appl. Phys. Lett., 2006, 88, 3105 Search PubMed.
- T. Sourmail, Prog. Mater. Sci., 2005, 50, 816–880 CrossRef CAS.
- D. X. Chen, O. Pascu, A. Roig and A. Sanchez, J. Magn. Magn. Mater., 2010, 322, 3834–3840 CrossRef CAS.
- X. H. Li, C. L. Xu, X. H. Han, L. A. Qiao, T. Wang and F. S. Li, Nanoscale Res. Lett., 2010, 5, 1039–1044 CrossRef CAS PubMed.
- X. G. Liu, B. Li, D. Y. Geng, W. B. Cu, F. Yang, Z. G. Xie, D. J. Kang and Z. D. Zhang, Carbon, 2009, 47, 470–474 CrossRef CAS.
- H. Wang, Y. Y. Dai, W. J. Gong, D. Y. Geng, S. Ma, D. Li, W. Liu and Z. D. Zhang, Appl. Phys. Lett., 2013, 102, 223113 CrossRef.
- X. F. Zhang, X. L. Dong, H. Huang, Y. Y. Liu, W. N. Wang, X. G. Zhu, B. Lv, J. P. Lei and C. G. Lee, Appl. Phys. Lett., 2006, 89, 3115 Search PubMed.
- T. Wang, H. D. Wang, X. Chi, R. Li and J. B. Wang, Carbon, 2014, 74, 312–318 CrossRef CAS.
- X. H. Li, J. Feng, Y. P. Du, J. T. Bai, H. M. Fan, H. L. Zhang, Y. Peng and F. S. Li, J. Mater. Chem. A, 2015, 3, 5535–5546 CAS.
- X. M. Zhang, G. B. Ji, W. Liu, B. Quan, X. H. Liang, C. M. Shang, Y. Cheng and Y. W. Du, Nanoscale, 2015, 7, 12932–12942 RSC.
- H. L. Zhu, Y. J. Bai, R. Liu, N. Lun, Y. X. Qi, F. D. Han and J. Q. Bi, J. Mater. Chem., 2011, 21, 13581–13587 RSC.
- Y. Chen, X. Liu, X. Mao, Q. Zhuang, Z. Xie and Z. Han, Nanoscale, 2014, 6, 6440–6447 RSC.
- J. Y. Fang, T. Liu, Z. Chen, Y. Wang, W. Wei, X. G. Yue and Z. H. Jiang, Nanoscale, 2016, 8, 8899–8909 RSC.
- H. Lv, G. Ji, W. Liu, H. Zhang and Y. Du, J. Mater. Chem. C, 2015, 3, 10232–10241 RSC.
|
This journal is © The Royal Society of Chemistry 2016 |
Click here to see how this site uses Cookies. View our privacy policy here.