DOI:
10.1039/C6RA18898B
(Paper)
RSC Adv., 2016,
6, 106297-106307
Demulsification of heavy oil-in-water emulsions by reduced graphene oxide nanosheets
Received
25th July 2016
, Accepted 23rd October 2016
First published on 24th October 2016
Abstract
A series of reduced graphene oxide (rGO) materials was synthesized by simple, clean, and controlled hydrothermal reduction of graphene oxide (GO). The chemical composition and properties of the obtained rGO materials were characterized by FT-IR, UV-visible absorption spectroscopy, XPS, AFM, zeta potential measurement, and interfacial tension analysis. As two-dimensional surfactants, various samples of rGO were employed to demulsify an oil-in-water emulsion. The demulsification performance of various demulsifiers was found to be considerably improved with increasing the reduction degree of the GO. In particular, the demulsification efficiency could reach about 99.97% for the rGO-110 sample. Quantum chemical calculations with a high quantum level of density functional theory with the empirical dispersion corrections approach (DFT-D) were performed to understand the mechanism of demulsification. The results revealed that rGO has a stronger adsorbability for asphaltene molecules than GO does by π–π interaction. Due to the strong π–π interaction between the rGO nanosheets and the asphaltenes, the protective film stabilizing the oil-in-water emulsion was more easily destroyed, thus promoting the oil droplets to coalesce to realize the separation of oil from water.
1. Introduction
Nowadays, with the gradual ageing of oil fields, the yield of conventional petroleum has not been able to support the daily requirements. Unconventional oils such as heavy oil and oil sands are becoming increasingly important.1–3 For exploiting heavy oil and oil sands, water blending is one of the most important methods to enhance their recovery; however, this often brings a large amount of o/w or w/o emulsions in their production processes.4–7 Compared with conventional oil, heavy oil and bitumen contain more nature emulsifiers, such as asphaltenes, which possess complex molecular structures with saturated substituents and amine, hydroxyl and carboxyl polar groups on the polycyclic aromatic rings.8,9 As amphiphiles, asphaltene molecules can readily adsorb on the oil–water interface to form a rigid film and make emulsions extremely stable.10–12 Therefore, treatment of various heavy oil and bitumen emulsions has become one of the most serious challenges in the petroleum industries. Although various approaches have been developed to destabilize these emulsions, it remains a challenge to separate the oil from the emulsions.
Generally, traditional methods to separate oil from oil–water emulsions include gravity separation, flotation, coagulation, ultra-centrifugation, and membrane filtration.13 However, chemical demulsification is the most widely used method for treating oil-in-water emulsions. Chemical demulsifiers are amphiphilic compounds, which contain hydrophilic and hydrophobic groups. The hydrophilic part usually contains oxyethylene, hydroxyl, carboxyl, or amine groups, while the hydrophobic part includes mainly groups of alkyls, alkylphenols, or oxypropylenes.14–18 A lot of research has shown that the demulsification efficiency depends not only on the solution conditions but, more importantly, upon the molecular structure and composition of the demulsifier itself.2,4,11,13,19–21 To ensure a good performance, the demulsifier should have a good dispersibility in the continuous phase and have a strong interaction with the natural emulsifiers to destroy the protective film stabilizing the emulsion, thus promoting the oil droplets to coalesce to realize the separation of oil from water.22 New methods of treating the emulsions include the use of aerogels,23 nanomaterials,24 and many other materials with special wettability.25 For example, Xu et al.26 prepared magnetically responsive composite absorbent particles coated with interfacially active materials and used them to dewater a water-in-dilute bitumen emulsion. It was found that the particles could rapidly absorb the emulsified water from the stable water-in-heavy naphtha diluted bitumen emulsion and possessed a good dewatering performance at ambient conditions.
Recently, we reported a two-dimensional carbon material, a graphene oxide (GO) sheet, which was found to be a highly efficient, rapid, and universal demulsifier to break up crude/heavy oil-in-water emulsions and to emulsify oily wastewater at ambient conditions.27 GO is the product of chemical oxidation and exfoliation of graphite powders, and contains domains of sp2 carbon atoms as well as randomly distributed sp3-hybridized carbons bearing oxygen functional groups. Thus, it can be viewed as an amphiphile with various hydrophilic oxygen-containing functional groups and hydrophobic sp2-conjugated regions.28 As a novel surfactant, GO can be used as a dispersing agent to disperse π-conjugated materials of graphite and carbon nanotubes in water, as a adsorbent to adsorb drug or dye molecules, and as a stabilizer to prepare Pickering emulsions.29–31 The π-conjugated aromatic hydrocarbons in its basal plane contribute to all those characteristics of GO, which allow GO to adsorb onto interfaces to reduce the surface and interfacial tension or even wrap insoluble particles.32–34
GO, as a two-dimensional surfactant,32 has various useful properties including amphiphilicity, adsorbability, and dispersibility in water, and can be well tuned by changing the solution environment or the functional groups on the basal plane of the graphene sheets. For example, low pH values could promote the protonation of the –COOH groups, resulting in decreased edge charges and hydrophobicity of the sheet. In this study, to investigate the effect of the functional groups in GO on its demulsification efficiency, a series of reduced graphene oxide (rGO) materials was synthesized as demulsifiers by a simple, clean, and controlled hydrothermal dehydration route.35 By changing the content of various oxygen-containing groups on the GO sheet surface, the amphiphilicity of various rGO samples was controlled and its effect on the demulsification performance was investigated. The purpose of this work is to investigate the relationship between the chemical structure of the GO materials and the demulsification performance. In addition, the effects of the type of crude oil, pH, and salinity of the system on the demulsification process were also studied. It is expected to be a novel and highly efficient nanomaterial demulsifier for use in separating oil-in-water emulsions.
2. Experimental and computational details
2.1. Materials
Three crude oil samples were used for the preparation of the emulsions, which were provided by Tahe oil field (Xinjiang Province, China), Changqing oil field (Shanxi Province, China), and Shengli oil field (Shangdong Province, China), respectively. The physicochemical properties of the crude oil samples were characterized and are listed in Table 1. The SARA fraction (saturates, aromatics, resins, and asphaltenes) of the crude oil samples was analyzed by a classical chromatography separation method.36 All the chemicals were of analytical grade purity and directly used without further treatment. Ultrapure water (18.25 MΩ cm) was used throughout all experiment processes.
Table 1 Composition and physical properties of the crude oil samples
Crude oils |
Shengli |
Changqing |
Tahe |
Specific gravity |
0.98 |
0.88 |
0.86 |
API gravity |
12.88 |
28.21 |
33.03 |
Asphaltenes (wt%) |
9.8 |
4.7 |
3.4 |
Resins (wt%) |
34.9 |
25.5 |
22.3 |
Aromatics (wt%) |
23.3 |
15.2 |
15.6 |
Saturates (wt%) |
21.2 |
48.4 |
49.3 |
2.2. Synthetic approaches
GO was prepared using a modified Hummers method from graphite powders (Scheme 1).37–39 Briefly, graphite (12 g) was put into a solution of concentrated H2SO4 (50 mL), K2S2O6 (10 g), and P2O5 (10 g) and kept at 80 °C for 8 h. The mixture was washed with ultrapure water to remove the residual acid and then dried under ambient conditions overnight. The pretreated graphite (5 g) was put into cold 98% H2SO4 (200 mL), followed by addition of KMnO4 (25 g) with stirring at 0 °C. The as-obtained mixture was stirred at 35 °C for 4 h, and then diluted with 250 mL of ultrapure water in an ice bath to keep the temperature below 50 °C. After addition of the 250 mL of ultrapure water, the mixture was stirred for 2 h, and then an additional 750 mL ultrapure water was added. The mixture was treated with 50% H2O2 until the color of the solution changed to brilliant yellow. The mixture was then centrifuged and washed with 10% hydrochloric acid aqueous solution (2 L) and then water until the pH of the solution became neutral. The resulting solid was dispersed in water by ultrasonication for 90 min to get a GO aqueous dispersion (0.5 wt%). The obtained brown dispersion was centrifugated at 4000 rpm for 10 min to remove any aggregates and then purified by dialysis for 1 week to remove the remaining salt impurities. Finally, the resultant mixture was dried by vacuum freeze-drying for the following experiments.
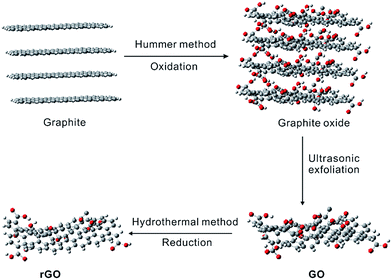 |
| Scheme 1 Preparation of rGO using graphite as the starting material. | |
To prepare an aqueous rGO suspension, 40 mL of 1 mg mL−1 GO aqueous solution was transferred to a Teflon-lined autoclave and heated for 2 h at various temperatures (80–110 °C). Then the autoclave was naturally cooled to room temperature. As shown in Fig. 1, the original yellow-brown suspension darkened after it was heated for 2 h at the desired temperature, and the rGO products obtained at various temperatures (80–110 °C) were defined as rGO-80, rGO-90, rGO-100, and rGO-110, respectively. When the GO aqueous solution was heated at 120 °C for 2 h, a homogeneous black dispersion with a small amount of black precipitate was obtained. For this reason, we didn’t use it as a study object in this work. The precipitation of the reduced sheets presumably occurred as the surface wettability became less hydrophilic as a result of the removal of epoxy and hydroxyl groups and the recovery of the conjugated domains, which increased the incompatibility with the aqueous medium. These results suggest the existence of π–π stacking between rGO sheets in the aqueous solution and also the presence of residual oxygenated functional groups on the rGO sheets.
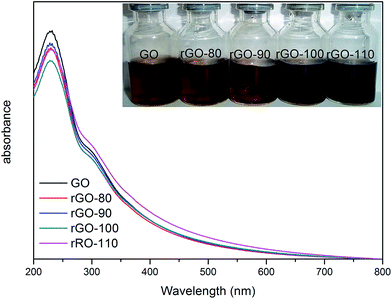 |
| Fig. 1 UV-vis absorption spectra for the GO and rGO samples. Inset shows the color change of the GO solution (0.5 mg mL−1) before and after hydrothermal treatment at 80–110 °C for 2 h. | |
2.3. Characterization
The oxygen-containing groups on GO and rGO were identified with Fourier transform infrared spectroscopy (FT-IR, Nexus870). The UV-visible absorption spectra of GO and rGO were recorded on a Shimadzu UV 2600PC spectrophotometer. The chemical composition and state of the various elements present on the outermost region of the GO and rGO nanosheets were investigated by XPS measurements using an X-ray photoelectron spectrometer (XPS, ESCALAB 250Xi). The surface morphology of the samples was analyzed by atomic force microscopy (AFM, Bruker Multimode-8) with tapping mode operating at a scan rate of 2 Hz. The zeta potentials of the samples were measured with a Zeta PALS instrument (Brookhaven Instruments Co., New York). The measurement was repeated 10 times to ensure the accuracy of the results. The interfacial tension of the GO-based demulsifier solution with different concentrations was measured using a pendant drop method by a contact angle analyzer (JC2000D3, Shanghai, China). Pure water was first used to calibrate the instrument at room temperature. Heptanes were employed as the oil phase, and the solution containing GO with desired concentrations was used as the water phase. Measurements were performed by adding a water drop into the oil phase.
2.4. Demulsification test
The heavy crude oil was first kept at 60 °C for 1 h to increase its flowability. For emulsion preparation, ultrapure water was used for the continuous phase and the provided heavy oil was used for the dispersed phase. Briefly, 20 g heavy oil and 380 g ultrapure water were mixed together and stirred at 28
000 rpm for 5 min using a homogenizer (Fluko, FA25) to obtain an o/w emulsion (5 wt%). The freshly prepared emulsion was found to be stable and was used for the demulsification tests. To perform the demulsification, a GO-based demulsifier dispersion (1 mg mL−1) was added to the oil-in-water emulsion (40 mL) contained in a colorimeter tube. For the blank test, deionized water was used to replace the GO suspension as the demulsifier. Then, the colorimeter tube was shaken 200 times to ensure that the GO and the emulsion can be uniformly mixed. After thoroughly shaking, the mixture was then placed under ambient conditions to observe the oil/water separation. To quantitatively determine the demulsification efficiency, the oil–water mixture was settled by gravitation for 30 min, and then the separated water at the bottom of the test bottle was separated carefully using a separatory funnel. Successively, the oil in the separated water samples was extracted and diluted with ligroin. The residual oil content in the separated water was measured using an ultraviolet spectrophotometer (Unico, UV-2000) by monitoring the absorbance at 256 nm. By comparing with a standard curve obtained from a series of standard solutions with different oil content, the oil concentration was calculated using the following equation: |
 | (1) |
where c0 (mg L−1) is the oil concentration, m0 (mg) is the corresponding mass of oil in the standard sample, and Vw (mL) is the water volume. The demulsification efficiency (ED) was calculated from the measured residual oil content using the equation: |
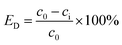 | (2) |
where c0 and ci are the initial and residual oil content in the water, respectively.40 Each sample was tested three times, and the reported ED value is the average of the three test results.
2.5. DFT-based quantum mechanical calculations
To reveal the demulsification mechanism, quantum mechanical calculation was employed to study the interaction between the rGO and asphaltenes. Geometry optimization of the asphaltenes and rGO was performed with the ORCA-3.0.3 program package41 using the BLYP functional with Grimme’s long-range dispersion correction (BLYP-D3)42 and the double-ζ Def2-SVP basis sets.43 This combination could be efficient for large systems where dispersion forces are of general importance. The geometrical counterpoise (gCP) correction scheme44 was used to semi-empirically treat the basis set superposition error (BSSE) effects. The ability of GO or rGO to adsorb the asphaltenes was evaluated through the binding energy (Ebind) as shown in eqn (3): |
Ebind = Eaggregate − (ΣEcomponents)
| (3) |
The non-covalent interaction (NCI) technique introduced by Johnson et al.45 was also employed to localize and identify the non-covalent interactions. The NCI analysis was carried out with the Multiwfn 3.3 program46 and the NCI regions were then visualized using the Visual Molecular Dynamics software version 1.9.1.47
3. Results and discussion
3.1. Characterization of the GO nanosheets
Fig. 1 shows the UV-vis absorption spectra for the stable GO and rGO dispersions in water. A maximum absorption peak is observed at ∼231 nm and a weak shoulder at ∼300 nm, which correspond to the π–π* transitions of aromatic C
C and n–π* transitions of C
O bonds, respectively. Generally, the intensity of the absorption peak is highest suggesting the best dispersion ability of the GO.48 As shown in Fig. 1, the maximum absorption peaks of the rGOs decrease through the hydrothermal reaction. In comparison, a weak hump is observed at 260 nm in the case of rGO-110, which is assigned to the excitation of π electrons, suggesting the restoration of the π-conjugated network within the GO nanosheets by reduction. The inset shows that the GO solution color changes from yellow-brown to black after hydrothermal treatment, which is a result of an increase in the hydrophobicity of the sheets caused by a decrease of polar groups on the surface of the sheets.
In order to study the changes of functional groups in the GO caused by reduction at different temperature, various samples of the GO and rGO were characterized by FT-IR. As shown in Fig. 2, various characteristic vibration peaks are observed in the FT-IR spectra. For example, the broad and intense peak centered at 3420 cm−1 is assigned to the O–H group, while a peak for C
O appears at 1735 cm−1. The peak at 1405 cm−1 is attributed to the bending vibration of the O–H bond. The peak for the C–OH stretching vibration appears at 1220 cm−1 and the peak for C–O–C is observed at 1060 cm−1. The peak at 1620 cm−1 is assigned to the vibration of the adsorbed water molecules and is also from the contribution of the skeletal vibration of unoxidized graphitic domains.48–50 The peaks appearing in the range of 2330–2350 cm−1 are attributed to the stretching vibration of CO2.51 The results of the FT-IR spectra suggested that the hydrothermal reduction changed only the quantities of the various functional groups on the graphitic skeleton but not the group species.
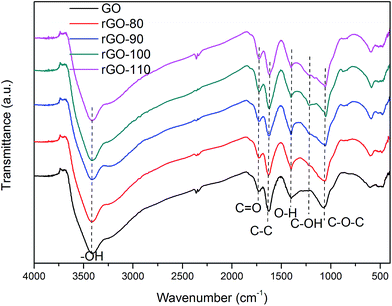 |
| Fig. 2 FT-IR spectra of GO, rGO-80, rGO-90, rGO-100, and rGO-110. | |
XPS analysis was further used to investigate the components and surface chemical state of the C and O elements in GO and rGO. Fig. 3a shows the high-resolution XPS spectra of C 1s, which could be fitted into five components: C–C/C–H (∼284.6 eV), C–OH (∼285.5 eV), C–O–C (∼286.9 eV), C
O (∼288 eV),44 and O
C–O (∼289.0 eV).40 As shown in the C 1s spectra of the samples, the peak position of various functional groups was not changed with increasing the hydrothermal temperature. However, the peak intensity was varied, which implied that the content of the various functional groups in rGO was changed. The relative content of the various components is summarized in Table 2. The molar ratios of sp2/sp3 carbon obtained from the spectra of GO, rGO-80, rGO-90, rGO-100, and rGO-110 were found to be 0.99, 1.08, 1.15, 1.26, and 1.59, respectively, indicating that the amount of sp2 carbon increased with increasing the hydrothermal temperature. For the high-resolution XPS spectra of O 1s, the peak of O(a) at ∼531.4 eV was assigned to the oxygen atoms in the carboxyl or carbonyl groups (C
O), while the peak of O(b) at ∼532.5 eV was assigned to epoxy, hydroxyl, or carboxyl groups (C–O), and the peak of O(c) at ∼533.6 eV was assigned to oxygen atoms in water and chemisorbed oxygen species. As shown in Table 2, the relative content of the oxygen atoms in the epoxy, hydroxyl, and carboxyl groups for these four rGO samples decreased slightly, which agrees well with the results obtained from the C 1s spectra. The C/O atomic ratios for GO, rGO-80, rGO-90, rGO-100, and rGO-110 were determined as 2.08, 2.15, 2.19, 2.23, and 2.51, respectively. These results demonstrate that the amount of oxygen-containing groups was partially reduced and the total area of the sp2 domains slightly increased after reduction.
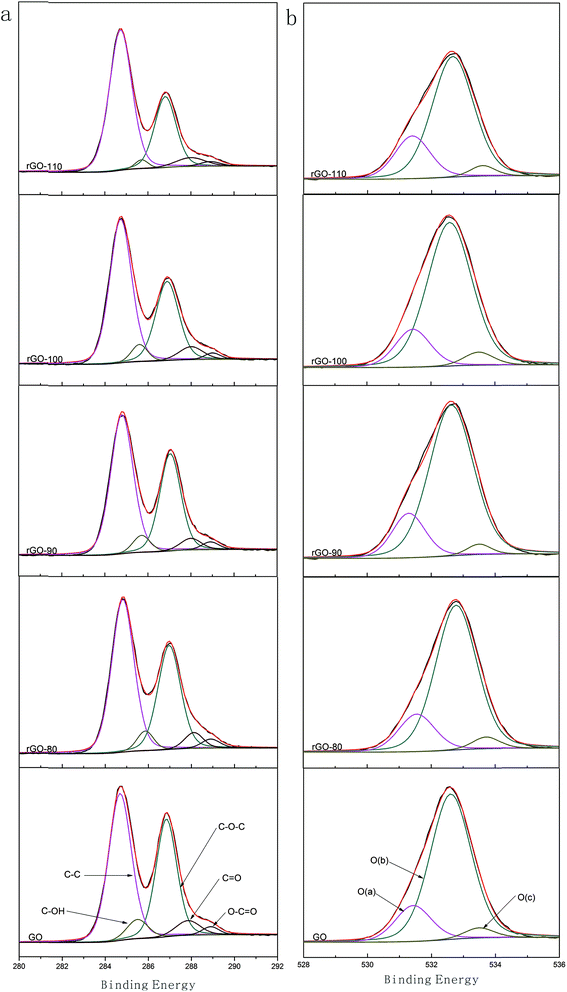 |
| Fig. 3 X-ray photoelectron spectra of GO, rGO-80, rGO-90, rGO-100, and rGO-110: (a) C 1s and (b) O 1s. | |
Table 2 Relative atomic concentration (%) of various carbon and oxygen groups in the samples of GO, rGO-80, rGO-90, rGO-100, and rGO-110
Samples |
C–C/C–H |
C–OH |
C–O–C |
C O |
O C–O |
O(a) |
O(b) |
O(c) |
GO |
49.91 |
6.08 |
36.59 |
5.35 |
2.08 |
17.94 |
82.06 |
4.05 |
rGO-80 |
51.98 |
5.02 |
35.41 |
5.61 |
1.99 |
18.47 |
81.53 |
4.33 |
rGO-90 |
53.45 |
5.05 |
34.42 |
5.35 |
1.73 |
18.33 |
81.67 |
6.42 |
rGO-100 |
55.66 |
4.84 |
32.21 |
5.51 |
1.78 |
18.76 |
81.24 |
5.06 |
rGO-110 |
58.52 |
4.85 |
29.82 |
5.11 |
1.69 |
28.12 |
70.29 |
7.89 |
The morphology of the various samples was observed by atomic force microscopy (AFM). It is shown that the thickness of the rGO-100 and rGO-110 sheets is about ∼1 nm, matching well with that of the reports for a single sheet of rGO (Fig. 4).52,53 Such a thickness is less than the ∼1.5 nm for the GO sample before reduction. However, it is still somewhat larger than the theoretical value for a perfectly flat sp2 carbon atom network, which is attributed to the intrinsic out-of-plane deformation of graphene as well as to the instrumental offset.54,55
 |
| Fig. 4 AFM images of GO, rGO-80, rGO-90, rGO-100, and rGO-110 on silicon substrate. | |
It is known that exfoliated graphite oxide (or GO) can form well-dispersed aqueous colloids.56 The zeta potential of the samples was characterized and the result shows that these rGO sheets are highly negatively charged when dispersed in water at pH = 5.7 (Fig. 5), which is attributed to the oxygen-containing groups on the GO sheet surfaces. It is also noticed that the zeta potential became less negative when the GO materials were reduced by hydrothermal dehydration. Such a result comes from the fact that some oxygen-containing groups were partially eliminated by the hydrothermal reduction.
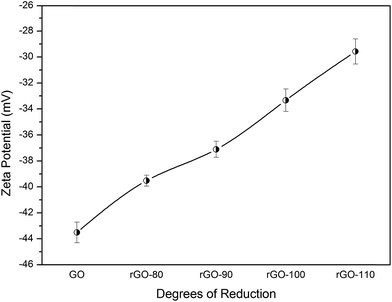 |
| Fig. 5 The zeta potentials of various rGO samples with different reduction levels. | |
3.2. The demulsification performance of GO-based demulsifiers
To study the demulsification performance of the GO-based demulsifiers, oil-in-water emulsions prepared with heavy oil at 5 wt% were demulsified using the synthesized demulsifiers. Once the demulsifiers were added to the heavy oil-in-water emulsion and after shaking, the color of the emulsion changed immediately from dark brown to black. Oil floccules aggregated rapidly to form a suspended oil phase floating on the water surface within 2 minutes. As a control, no phase separation was observed for the sample with only water added. Fig. 6a shows the oil content in the separated water as a function of the demulsifier dosage. The demulsification performance of the GO demulsifiers was found to be considerably improved with increasing the degree of reduction of the GO materials, which might be a result of an increase in the hydrophobicity of the material caused by a decrease in polar functionality on the surface of the GO sheets. Therefore, it can be concluded that the demulsification performance of the various GO demulsifiers is significantly dependent on the molar ratio between the hydrophobic and hydrophilic region of the GO sheets. In addition, it was found that the best demulsification performance was obtained at a dosage of about 30–40 mg L−1 for various rGO samples. In particular, the demulsification efficiency could reach about 99.97% for the rGO-110 sample (Fig. 6b), which is better than that in our previous report.27 The results indicate that the rGO nanosheets could be used as an effective demulsifier for the o/w emulsion with a higher demulsification efficiency than the commercial demulsifiers and the Janus magnetic submicronic particles reported recently. For example, Rajak et al. investigated the demulsification performance of eight commercial demulsifiers for o/w emulsions (1 wt%).13 It was observed that 98% oil could be separated by some demulsifiers under optimum operating conditions. Ali et al. prepared Janus magnetic submicronic particles and compared their demulsification performance with different commercial demulsifiers for demulsifying heavy crude oil emulsion.24 It was found that the demulsification efficiency of the prepared particles is about 95%.
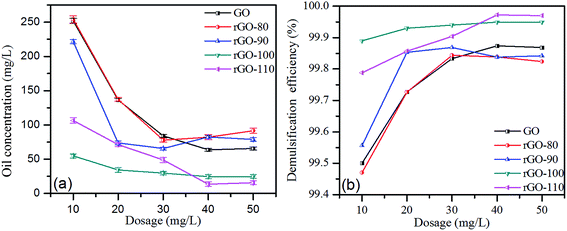 |
| Fig. 6 (a) Residual oil content in the separated water as a function of demulsifier dosage. (b) The corresponding demulsification efficiency as a function of demulsifier dosage for the GO and rGO samples. | |
It was noticed that the color of the separated water after demulsification by various GO demulsifiers was significantly different. Based on our previous report,27 it is believed that the color of the separated water originates from the GO nanosheets. As shown in Fig. 7, the color of the separated water treated by GO is pale yellow, while it became much lighter with the reduction of GO. In particular, the water treated by rGO-110 is nearly colorless. This demonstrates that on increasing the degrees of reduction, the rGOs preferentially transfer from the water to the oil phase after demulsification. A similar phenomenon has also been found in demulsification by changing the solution pH value. i.e. the separated water derived from the demulsification at pH = 5.7 is yellow, whereas it is nearly colorless at pH = 2, which is attributed to the increased hydrophobicity of GO in a lower pH solution. In this work, with hydrothermal reduction, the rGO becomes less hydrophilic and prefers to migrate into the oil phase after demulsification. As a result, the color of the separated water treated by rGO-110 was colorless.
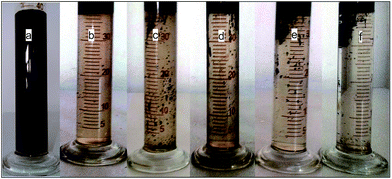 |
| Fig. 7 Demulsification process after addition of water and various demulsifiers at optimal dosage after settling for 30 min: (a) water, (b) GO, (c) rGO-80, (d) rGO-90, (e) rGO-100, and (f) rGO-110. | |
GO as a two-dimensional surfactant, with amphiphilicity and dispersability characteristics, can be tuned by changing the pH of the environment or by varying the size or reduction degree of the GO itself.57 To understand the effect of a different reduction degree of the GO on its interfacial activity and demulsification efficiency, the heptanes/water interfacial tension with addition of the GO-based demulsifiers in the solution was measured and compared. As shown in Fig. 8, the interfacial tension of heptanes/water gradually decreased with the increase of the concentration of various demulsifiers in the solution, which is consistent with a previous report.58 In particular, the interfacial tension reduced much more with the increasing GO reduction degree. On comparison of the reduction of the interfacial tension with the demulsification efficiency, a close relationship between them was found (Fig. 8b). For example, samples of rGO-100 and rGO-110 are more effective at reducing the interfacial tension, corresponding to a more efficient removal of emulsified water. In contrast, GO, rGO-80, and rGO-90 are less effective at reducing the interfacial tension, which corresponds to a relatively lower demulsification efficiency. Therefore, it is believed that reducing the interfacial tension of oil/water is an important factor contributing to the demulsification process.
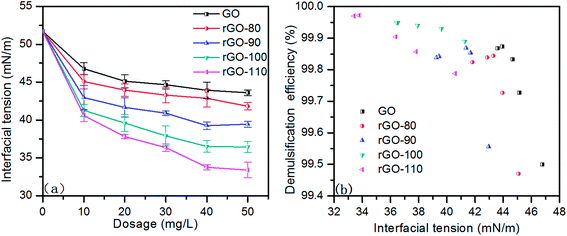 |
| Fig. 8 (a) Effect of various GO demulsifiers on the interfacial tension of heptanes/water at 25 °C. (b) Relationship between the interfacial tension and the demulsification efficiency. | |
3.3. Factors affecting the demulsification efficiency
The effects of the various factors of pH, salinity, and type of crude oil on the demulsification efficiency were investigated. Taking the demulsifiers of GO and rGO-110 as examples, as shown in Table 3, it was found that the oil separation efficiency in the acidic and neutral condition oil varied very little. However, the demulsification efficiency for both of GO and rGO-110 was decreased in the alkaline solution, especially for a strong alkaline condition of pH = 10. Such a result might be attributed to the fact that the carboxyl and hydroxyl groups could be deprotonated in the alkaline solution, leading to an electrostatic repulsion between the demulsifiers and oil droplets. As a result, the demulsification efficiency of GO and rGO-110 in alkaline solution was notably reduced. These findings are in good consistency with our previous reports.27
Table 3 Effects of the aqueous pH, solution salinity, and the crude oil type on the demulsification performance
Crude oil sample |
Dosage (mg L−1) |
Aqueous phase properties |
Residual oil content in the separated water (mg L−1) |
Demulsification efficiency (%) |
Continuous phase |
pH |
GO |
rGO-110 |
GO |
rGO-110 |
Shengli |
40 |
Ultrapure water |
2 |
68.5 ± 3.5 |
19.3 ± 1.8 |
99.87 |
99.97 |
5.7 |
64.4 ± 1.9 |
13.5 + 1.3 |
99.87 |
99.97 |
8 |
171.2 ± 6.3 |
69.2 ± 3.1 |
99.66 |
99.86 |
10 |
966.7 ± 40.4 |
813.3 ± 35.2 |
98.07 |
98.38 |
40 |
Formation water |
8.9 |
840 ± 37.2 |
544 ± 20.8 |
98.32 |
98.91 |
120 |
8.9 |
415 ± 18.4 |
210.5 ± 8.3 |
99.17 |
99.58 |
200 |
8.9 |
340.3 ± 8.0 |
170.2 ± 4.0 |
99.32 |
99.66 |
Changqing |
40 |
Ultrapure water |
5.7 |
64.7 ± 2.5 |
13.9 ± 1.4 |
99.87 |
99.97 |
Tahe |
5.7 |
68.1 ± 2.4 |
14.3 ± 1.4 |
99.87 |
99.97 |
It is well known that the salt content in aqueous solution plays an important role on the interfacial properties of the emulsion and its stability.59,60 Therefore, the oil-in-water emulsions were prepared by simulating the Daqing formation water to study the influence of the solution salinity on the demulsification performance of GO and rGO. The composition of the synthetic formation water is given in Table 4. It was found that the demulsification efficiency for rGO-110 at a dosage of 40 mg L−1 was about 98.91% (Table 3). As the dosage increased to 120 and 200 mg L−1, the demulsification efficiency correspondingly increased to 99.58% and 99.66%, respectively. A similar tendency was observed for GO; the demulsification efficiency increased from 98.32 to 99.32% with the dosage increasing from 40 to 200 mg L−1. These results indicate that the solution salinity does have some adverse influence on the demulsification performance of GO and rGO. The possible reasons might come from two aspects. On one hand, the solution salinity changed the interfacial properties of the emulsion. On the other hand, the prepared formation water had a pH value of 8.9, which decreased the demulsification efficiency as discussed above.
Table 4 Composition of the synthetic formation water
Composition |
NaCl |
KCl |
CaCl2 |
MgCl2·H2O |
Na2SO4 |
NaHCO3 |
Concentration (mg L−1) |
2294 |
13 |
42 |
17.2 |
75 |
1860 |
To study the crude oil composition and American Petroleum Institute (API) gravity on the demulsification performance of the demulsifiers, two medium crude oil samples produced from Changqing and Tahe (Table 1) were used to prepare the emulsions. As shown in Table 3, it was found that the demulsification efficiency of GO and rGO-110 for the different crude oil-in-water emulsion varied very little. For example, the demulsification efficiency of rGO-110 for the two medium crude oil emulsions also reached as high as 99.97%, which was same as that of the heavy crude oil sample from Shengli. The results indicate that the rGO materials are universal and highly efficient demulsifiers, which could be used to separate the oil from various types of crude oil emulsions.
3.4. The possible demulsification mechanism
To understand the demulsification processes of the crude oil-in-water emulsion driven by the rGO, the morphologies of the emulsion before and after demulsification were observed with a polarizing microscope. The optical microscopic images show that the oil droplets in the original emulsion generally have a size smaller than 10 μm in diameter (Fig. 9a). Once the demulsifier of the rGO-110 nanosheet suspension was added into the emulsion, coalescence of the fine oil droplets occurred to form irregular oil floccules (Fig. 9b). After thorough shaking, the oil floccules aggregated and floated rapidly to the water surface forming the suspended oil phase. Fig. 9c shows the optical image of the newly produced oil phase. It can be seen that some water droplets were wrapped in the oil phase, which could be mostly precipitated given a settling time of 2 hours at 60 °C. For the separated water phase, there were no oil droplets suspended in the water after settling for 30 min (Fig. 9d). These findings are in good consistency with our previous observations.27
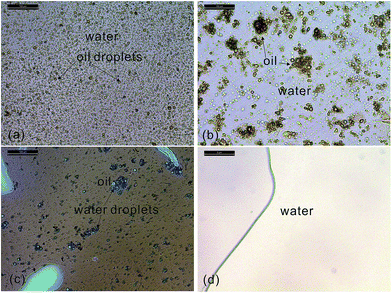 |
| Fig. 9 Micrographs of the oil–water mixture before and after demulsification: (a) the original oil-in-water emulsion, (b) coagulation of the oil droplets after addition of rGO-110 (50 mg L−1) without shaking, (c) the newly obtained oil phase after demulsification, and (d) the separated water phase after demulsification. | |
It is well known that the oil-in-water emulsion is mainly stabilized by a rigid film composed of asphaltenes at the oil–water interface.61 As a two-dimensional surfactant, the GO sheet has a larger lateral dimension of π-conjugated hydrocarbons on the basal plane, which allows GO to adsorb at the oil–water interface and interact with the asphaltenes.57 How the GO interacts with the asphaltenes plays a significant role in the demulsification process. Therefore, quantum chemical calculations were carried out to reveal the interactions between GO and asphaltenes. For the calculation, the molecular structures of GO, rGO, and asphaltenes are shown in Fig. 10. The rGO molecular model (Fig. 10c) was obtained by removing two epoxy and hydroxyl groups from the GO molecule (Fig. 10b). From the geometry optimization, it was found that the asphaltene molecule prefers to be adsorbed on the π-conjugated hydrocarbons on the basal plane of the GO sheet. To further identify the interaction characteristics between the GO/rGO and the asphaltene molecules, the reduced density gradient (RDG) was used. As shown in Fig. 11, a partially continuous isosurface appears in the overlapping region between the GO/rGO and the asphaltenes, which corresponds to the π–π interaction, indicating that the adsorption of asphaltenes on the GO/rGO sheets is realized mainly by π–π interaction. The interaction strength between the GO/rGO and asphaltenes could be reflected by their binding energy. It was shown that the binding energies are −63.24 kcal mol−1 for GO and −68.04 kcal mol−1 for rGO, indicating that the rGO has stronger adsorbability for asphaltene molecules than GO does. In other words, the π–π interaction between the GO/rGO and asphaltenes could be enhanced by the increase of the π-conjugated hydrocarbons on the basal plane of the GO sheets. Therefore, the demulsification efficiency of GO was increased by hydrothermal reduction of the oxygen-containing groups on the GO sheets. However, it should be pointed out that the oxygen-containing groups could not be fully removed from the GO sheets. It is necessary to keep some of them enabling the rGO to be well dispersed in the water phase. Due to the strong π–π interaction between the rGO nanosheets and the asphaltenes, the protective film enwrapping the oil droplets was destroyed, thus promoting the oil droplets to coalesce to realize the separation of oil from water.
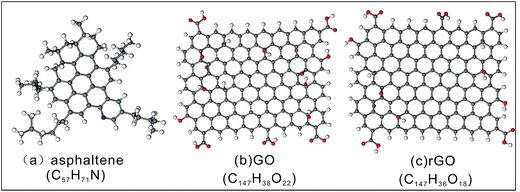 |
| Fig. 10 Molecular structures of asphaltene, GO, and rGO molecules used in the quantum chemical calculations. | |
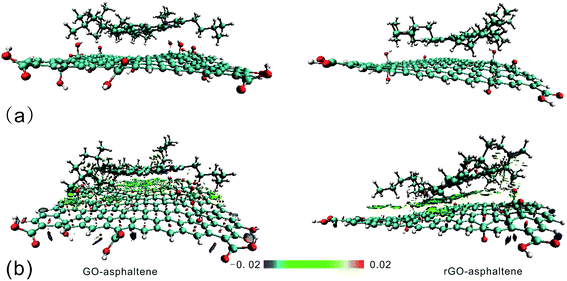 |
| Fig. 11 (a) The optimized structures of the GO/rGO and asphaltenes by BLYP-D3/Def2-SVP. (b) The color-filled reduced density gradient (RDG) map between GO/rGO and asphaltenes. The gradient isosurfaces (s = 0.6 a.u.) are colored on a blue-green-red (BGR) scale according to the sign(λ2)ρ over the range of −0.02 to 0.02 a.u. | |
4. Conclusions
In this work, a water-only reduced method was employed to prepare rGO from GO materials. It allows better control of the amphiphilicity and dispersibility of the GO compounds. The demulsification tests indicated that the demulsification performance of the rGO demulsifiers considerably improved with increasing the degree of reduction of the GO. In particular, rGO-110 was the most effective at demulsification and could recover 99.97% of the oil from the emulsions only by gravity settling at room temperature for 30 min. The separated water after demulsification was nearly colorless. It was believed that with hydrothermal reduction, the π-conjugated aromatic network area on the basal plane of the GO sheet was increased, which significantly strengthened its adsorbability and amphiphilicity. On the one hand, an increase of the adsorbability enables the rGO compounds to more easily interact with the asphaltenes through π–π interaction, which was demonstrated by the quantum chemical calculations. On the other hand, the improvement of the hydrophobicity makes the rGO tend to enter in the oil phase and obtain colorless separated water after demulsification. The results obtained in this work demonstrated that the properties and demulsification efficiency of the GO materials could be tuned by hydrothermal reduction. Therefore, the rGO materials might have a good prospect of application in recovering oil from crude oil-in-water emulsions and/or oily wastewater. In addition, the findings in this work also provide a new perspective to develop novel demulsifiers for water-in-crude oil emulsions.
Acknowledgements
The authors thank the fund support from the National Science Foundation of China (Grant No. 51574217; 51374195).
References
- S. L. Ren, T. Dang-Vu, H. Y. Zhao, J. Long, Z. H. Xu and J. Masliyah, Energy Fuels, 2009, 23, 334–341 CrossRef CAS.
- X. Feng and J. A. Behles, Energy Fuels, 2015, 29, 4616–4623 CrossRef CAS.
- Y. M. Xu, J. Y. Wu, T. Dabros, H. Hamza, S. Y. Wang, M. Bidal, J. Venter and T. Tran, Can. J. Chem. Eng., 2004, 82, 829–835 CrossRef CAS.
- R. Martínez-Palou and J. Aburto, Ionic Liquids as Surfactants – Applications as Demulsifiers of Petroleum Emulsions, ed. H. Scott, InTech, Rijeka, 1, 2015, vol. 11, pp. 306–326 Search PubMed.
- J. Tao, P. Shi, S. Fang, K. Li, M. Duan and P. Liu, RSC Adv., 2015, 5, 24591–24598 RSC.
- L. Kai, Chemical, Biological and Environmental Engineering-Proceedings of the International Conference on Cbee 2009, World Scientific, Singpore, 2010 Search PubMed.
- A. Mandal, A. Samanta, A. Bera and K. Ojha, Ind. Eng. Chem. Res., 2010, 49, 12756–12761 CrossRef CAS.
- H. Groenzin and O. C. Mullins, J. Phys. Chem. A, 1999, 103, 11237–11245 CrossRef CAS.
- T. Takanohashi, S. Sato and R. Tanaka, J. Jpn. Pet. Inst., 2013, 56, 61–68 CrossRef CAS.
- W. Kang, L. Guo, H. Fan, L. Meng and Y. Li, J. Pet. Sci. Eng., 2012, 81, 177–181 CrossRef CAS.
- J. Liu, Y. Zhao and S. Ren, Energy Fuels, 2015, 29(2), 1233–1242 CrossRef CAS.
- Z. Zhang, G. Xu, F. Wang, S. Dong and Y. Chen, J. Colloid Interface Sci., 2005, 282, 1–4 CrossRef CAS PubMed.
- V. K. Rajak, I. Singh, A. Kumar and A. Mandal, Pet. Sci. Technol., 2016, 34, 1026–1032 CrossRef CAS.
- C. W. Angle, Encyclopedic Handbook of Emulsion Technology, 2001, pp. 541–594 Search PubMed.
- N. Ali, B. Zhang, H. Zhang, W. Zaman, X. Li, W. Li and Q. Zhang, Colloids Surf., A, 2015, 472, 38–49 CrossRef CAS.
- R. Aveyard, B. P. Binks, P. D. I. Fletcher and J. R. Lu, J. Colloid Interface Sci., 1990, 139, 128–138 CrossRef CAS.
- J. Wang, F.-L. Hu, C.-Q. Li, J. Li and Y. Yang, Sep. Purif. Technol., 2010, 73, 349–354 CrossRef CAS.
- J. Wu, Y. Xu, T. Dabros and H. Hamza, Colloids Surf., A, 2005, 252, 79–85 CrossRef CAS.
- S. Li, N. Li, S. Yang, F. Liu and J. Zhou, J. Mater. Chem. A, 2014, 2, 94–99 CAS.
- M. A. Krawczyk, D. T. Wasan and C. Shetty, Ind. Eng. Chem. Res., 1991, 30, 367–375 CrossRef CAS.
- L. Hao, B. Jiang, L. Zhang, H. Yang, Y. Sun, B. Wang and N. Yang, Ind. Eng. Chem. Res., 2016, 55, 1748–1759 CrossRef CAS.
- M. Rondon, P. Bouriat, J. Lachaise and J. L. Salager, Energy Fuels, 2006, 20, 1600–1604 CrossRef CAS.
- Y. Si, Q. Fu, X. Wang, J. Zhu, J. Yu, G. Sun and B. Ding, ACS Nano, 2015, 9, 3791–3799 CrossRef CAS PubMed.
- N. Ali, B. Zhang, H. Zhang, W. Li, W. Zaman, L. Tian and Q. Zhang, Fuel, 2015, 141, 258–267 CrossRef CAS.
- Q. Ma, H. Cheng, A. G. Fane, R. Wang and H. Zhang, Small, 2016, 12, 2186–2202 CrossRef CAS PubMed.
- C. Liang, Q. Liu and Z. Xu, Energy Fuels, 2016, 30, 5253–5258 CrossRef CAS.
- J. Liu, X. Li, W. Jia, Z. Li, Y. Zhao and S. Ren, Energy Fuels, 2015, 29, 4644–4653 CrossRef CAS.
- L. J. Cote, J. Kim, V. C. Tung, J. Luo, F. Kim and J. Huang, Pure Appl. Chem., 2010, 83, 95–110 CrossRef.
- Z. Liu, J. T. Robinson, X. Sun and H. Dai, J. Am. Chem. Soc., 2008, 130, 10876–10877 CrossRef CAS PubMed.
- X. Y. Yang, X. Y. Zhang, Z. F. Liu, Y. F. Ma, Y. Huang and Y. Chen, J. Phys. Chem. C, 2008, 112, 17554–17558 CAS.
- B. Zhang, F. Li, T. Wu, D. Sun and Y. Li, Colloids Surf., A, 2015, 464, 78–88 CrossRef CAS.
- A. R. Koltonow, J. Kim, L. J. Cote, J. Luo and J. Huang, Mater. Res. Soc. Symp. Proc., 2011, 1344, 93–100 CrossRef.
- S. Stankovich, D. A. Dikin, R. D. Piner, K. A. Kohlhaas, A. Kleinhammes, Y. Jia, Y. Wu, S. T. Nguyen and R. S. Ruoff, Carbon, 2007, 45, 1558–1565 CrossRef CAS.
- X. Fan, W. Peng, Y. Li, X. Li, S. Wang, G. Zhang and F. Zhang, Adv. Mater., 2008, 20, 4490–4493 CrossRef CAS.
- Y. Zhou, Q. L. Bao, L. A. L. Tang, Y. L. Zhong and K. P. Loh, Chem. Mater., 2009, 21, 2950–2956 CrossRef CAS.
- M. Ding, Y. Zhang, J. Liu, W. Jia, B. Hu and S. Ren, AIChE J., 2014, 60, 2985–2993 CrossRef CAS.
- W. S. Hummers Jr and R. E. Offeman, J. Am. Chem. Soc., 1958, 80, 1339 CrossRef.
- N. I. Kovtyukhova, P. J. Ollivier, B. R. Martin, T. E. Mallouk, S. A. Chizhik, E. V. Buzaneva and A. D. Gorchinskiy, Chem. Mater., 1999, 11, 771–778 CrossRef CAS.
- Y. Xu, H. Bai, G. Lu, C. Li and G. Shi, J. Am. Chem. Soc., 2008, 130, 5856–5857 CrossRef CAS PubMed.
- J. Liu, X. Li, W. Jia, M. Ding, Y. Zhang and S. Ren, J. Dispersion Sci. Technol., 2015, 37, 1294–1302 CrossRef.
- F. Neese, Wiley Interdiscip. Rev.: Comput. Mol. Sci., 2012, 2, 73–78 CrossRef CAS.
- I. C. Lin, A. P. Seitsonen, I. Tavernelli and U. Rothlisberger, J. Chem. Theory Comput., 2012, 8, 3902–3910 CrossRef CAS PubMed.
- F. Weigend and R. Ahlrichs, Phys. Chem. Chem. Phys., 2005, 7, 3297–3305 RSC.
- H. Kruse and S. Grimme, J. Chem. Phys., 2012, 136, 154101 CrossRef PubMed.
- E. R. Johnson, S. Keinan, P. Mori-Sanchez, J. Contreras-Garcia, A. J. Cohen and W. Yang, J. Am. Chem. Soc., 2010, 132, 6498–6506 CrossRef CAS PubMed.
- T. Lu and F. Chen, J. Comput. Chem., 2012, 33, 580–592 CrossRef CAS PubMed.
- W. Humphrey, A. Dalke and K. Schulten, J. Mol. Graphics, 1996, 14, 33–38 CrossRef CAS PubMed.
- S. K. Srivastava and J. Pionteck, J. Nanosci. Nanotechnol., 2015, 15, 1984–2000 CrossRef CAS PubMed.
- K. Krishnamoorthy, M. Veerapandian, K. Yun and S. J. Kim, Carbon, 2013, 53, 38–49 CrossRef CAS.
- G. Venugopal, K. Krishnamoorthy, R. Mohan and S.-J. Kim, Mater. Chem. Phys., 2012, 132, 29–33 CrossRef CAS.
- M. Acik, R. Guzman and Y. J. Chabal, Mater. Res. Soc. Symp. Proc., 2011, 1205, 1205-L01-05 Search PubMed.
- S. F. Pei and H. M. Cheng, Carbon, 2012, 50, 3210–3228 CrossRef CAS.
- X. Yu, H. Cai, W. Zhang, X. Li, N. Pan, Y. Luo, X. Wang and J. G. Hou, ACS Nano, 2011, 5, 952–958 CrossRef CAS PubMed.
- C. Casiraghi, A. Hartschuh, E. Lidorikis, H. Qian, H. Harutyunyan, T. Gokus, K. S. Novoselov and A. C. Ferrari, Nano Lett., 2007, 7, 2711–2717 CrossRef CAS PubMed.
- A. Fasolino, J. H. Los and M. I. Katsnelson, Nat. Mater., 2007, 6, 858–861 CrossRef CAS PubMed.
- D. Li, M. B. Muller, S. Gilje, R. B. Kaner and G. G. Wallace, Nat. Nanotechnol., 2008, 3, 101–105 CrossRef CAS PubMed.
- K. Erickson, R. Erni, Z. Lee, N. Alem, W. Gannett and A. Zettl, Adv. Mater., 2010, 22, 4467–4472 CrossRef CAS PubMed.
- J. Kim, L. J. Cote, F. Kim, W. Yuan, K. R. Shull and J. Huang, J. Am. Chem. Soc., 2010, 132, 8180–8186 CrossRef CAS PubMed.
- M. Lashkarbolooki, S. Ayatollahi and M. Riazi, Energy Fuels, 2014, 28, 6820–6829 CrossRef CAS.
- C.-D. Yuan, W.-F. Pu, X.-C. Wang, L. Sun, Y.-C. Zhang and S. Cheng, Energy Fuels, 2015, 29, 6165–6176 CrossRef CAS.
- P. Tchoukov, F. Yang, Z. Xu, T. Dabros, J. Czarnecki and J. Sjoblom, Langmuir, 2014, 30, 3024–3033 CrossRef CAS PubMed.
|
This journal is © The Royal Society of Chemistry 2016 |