DOI:
10.1039/C6RA18803F
(Paper)
RSC Adv., 2016,
6, 96121-96137
Design, synthesis, characterization, cytotoxicity, molecular docking and analysis of binding interactions of novel acetylacetonatopalladium(II) alanine and valine complexes with CT-DNA and BSA†
Received
24th July 2016
, Accepted 4th October 2016
First published on 4th October 2016
Abstract
To enhance the development of potential anti-tumor agents, a pair of new, water-soluble and neutral acetylacetonatopalladium(II) complexes with alanine and valine amino acids have been synthesized. They were characterized by elemental analysis (C, H, N), FT-IR, UV-Vis, resonance signals in the 1H-NMR, ESI-MS and conductivity measurements. The molecular geometry, the energy level of the frontier orbitals and the NMR chemical shift values of the Pd(II) complexes have been calculated by using B3LYP/LANL2DZ method and 6-311g* basis set. Cytotoxicity effects of the complexes towards cancer cell line of K562 were measured using MTT assay. The interactions of these complexes with calf thymus DNA (CT-DNA) and Bovine Serum Albumin (BSA) were investigated under physiological condition in Tris–HCl buffer pH = 7 containing 0.02 M sodium chloride using spectroscopic methods including UV-Vis absorption spectroscopy, ethidium bromide displacement, fluorescence titration spectra and viscosity measurements. The results obtained from these analyses indicated that both complexes effectively interact with CT-DNA and BSA at low concentrations. Fluorescence titration revealed that the complexes strongly quench DNA bound ethidium bromide (EB) as well as the intrinsic fluorescence of BSA through static quenching procedures. Binding constant (Kb), apparent bimolecular quenching constant (Kq) and number of binding sites (n) for CT-DNA and BSA were calculated using Stern–Volmer equation. Also, thermodynamic parameters data suggested that hydrogen binding and van der Waals force play a major role in the association of CT-DNA–Pd(II) and BSA–Pd(II) complex formation and follow the binding affinity order of valine complex > alanine complex. The complexes exhibited groove binding with CT-DNA and interact with main binding pocket of BSA. In both cases the binding forces are spontaneous owing to −ΔG°. Finally, the results of molecular docking calculations of [Pd(acac)(Ala)] and [Pd(acac)(Val)] complexes for CT-DNA and BSA clarify the binding modes and binding sites which are in good accordance with experimental results.
Introduction
Cancer is certainly one of the major health concerns faced by our society and serves as a primary target in the field of medicinal chemistry. The landmark finding of cisplatin by Rosenberg in 1965 leading to a revolution of anticancer drug research based on metallopharmaceuticals.1,2 Owing to the dose limiting toxic side effects of cisplatin, the majority of recent research work is aimed at the discovery of platinum complexes which are (i) not cross-resistant with cisplatin, (ii) have fewer toxic side-effects, and (iii) active against a broad range of types of cancer.3,4 Also, non-platinum metal based anticancer compounds mainly includes Au, Ru, Ti, Cu, Zn, Ga and palladium complexes.5 Among them, palladium(II) complexes become alternative candidate of Pt(II) complexes for treating cancers. Over the last decade, a number of contributions on the synthesis and anticancer activity of palladium(II) complexes were reported.3,6–8 This is because of the similarity in chemistry, structural and thermodynamic analogy between Pd(II) and Pt(II) complexes.9–11 Nevertheless, because of higher lability of palladium to platinum analogs, bidentate ligands such as amino acids that are found in biological systems have been used to synthesize palladium anticancer complexes.12,13 These complexes do not dissociate easily in aqueous solution, which may reduce their toxic side effects or increase the concentration of drug inside the cell and thus improve the antitumor activities of these agents.14,15
It is well known that DNA and protein are potential biological targets of metal complexes. Therefore interactions of metal complexes with DNA and protein are very important to determining the mode and extent of binding in both theory and application.16 Depending on the exact nature of the metal and the ligand, the complexes can bind to DNA as: (i) covalent binding with purine and pyrimidine bases, (ii) intercalation between base pairs, (iii) in the minor groove, (iv) in the major groove, (v) hydrogen binding with different groups, (vi) ionic binding mainly with phosphate groups, (vii) hydrophobic and hydrophilic interactions etc.17 Binding of metal complexes and DNA result to inhibit the biosynthesis of DNA, which may hinder or block base replication.18 Thus, the study on the interaction of the transition metal complexes with DNA is of great importance for the design of new drugs and their application.
A stable drug–protein complex formation can be demonstrating important effect on the distribution, free concentration and metabolism of the drug in the bloodstream. Thus the drug–albumin complex may be considered as a model for gaining fundamental insights into the drug–protein interactions.19,20 Among the serum albumins, due to structural homology with human serum albumin, and unusual ligand-binding properties, bovine serum albumin is an attractive macromolecule mostly used in biophysical and biochemical studies.21,22
Recent new synthesized Pd(II) complexes revealed that the amino acid containing Pd(II) compounds had more growth inhibitory activity against cancer cells and less denaturant effect or damaging the BSA molecules.2,3,23,24 According to the results of cytotoxic studies, it is suggested that, addition of amino acid moiety to structure of Pt(II) or Pd(II) antitumor complexes probably has important role to improve the anti-proliferative properties, increased drug concentration in cancer cells and decrease structural inducing harmful effects on the carrier proteins.2,3,23 In view of the above attention, in this study, we have synthesized and characterized of two new water soluble Pd(II) complexes [Pd(acac)(Ala)] and [Pd(acac)(Val)] from amino acids (alanine and valine) and acac. The interaction between Pd(II) complexes with CT-DNA and BSA were investigated by various spectroscopic and molecular docking methods. The fluorescence quenching mechanism of CT-DNA–EB and BSA by each Pd(II) complex was explored at different temperatures (293, 300 and 310 K) and the binding constants (Kb), and binding site number (n), corresponding thermodynamic parameters (ΔG°, ΔH° and ΔS°) were calculated. As well as, the cytotoxicity of the complexes against human tumor cell line K562 was investigated by the MTT assay.
Experimental
Material and methods
Palladium chloride (PdCl2), NaHCO3, NaOH, NaCl, ethidium bromide (EB), acetylacetone (acac), DL-alanine (Ala), DL-valine (Val), highly polymerized calf thymus DNA (CT-DNA) type-1 and bovine serum albumin (BSA) were purchased from Aldrich Chemical Company. Solvent used were commercial grade and distilled before use. The UV-Vis spectra were recorded by UV-Vis spectrophotometer model Rayleigh-1601 at 200–700 nm. FT-IR spectra were recorded with FT-IR spectrometer model JASCO-460 plus at 400–4000 cm−1 using KBr pellets. 1H NMR spectra were recorded in FT-NMR spectrometer model Bruker DRX-250 Avance at 0–12 ppm in DMSO-d6 solvent and TMS were used as internal reference. Electrospray ionization mass spectrometry (ESI-MS) was processed on Finnigan™ LCQ™ DECA instrument equipped with ion trap mass spectrometer and electrospray ionization (ESI) source. Electrospray ionization in the positive mode was used. The electro spray capillary was set at 4.5 kV and the cone at 20 V. The ion source temperature was set at 250 °C and the flow rates for nitrogen bath and spray were 500 l h−1 and 50 l h−1, respectively. Conductivity measurements were carried out on a Systronics conductivity bridge, model 305, with cell (cell constant of 0.59) using conductivity water as a solvent. Fluorescence spectra were carried out using a PERKIN ELMER, LS-3 spectrofluorimeter. Viscosity measurements were done using on SCHOT AVS 450 viscometer.
Synthesis of acetylacetonato-DL-alaninopalladium(II), [Pd(acac)(Ala)]
Sodium salt of alanine was prepared by suspending 1 mmol (89 mg) DL-alanine and 1 mmol (84 mg) NaHCO3 in 10 ml of double distilled water and stirring for 20 min at 35–40 °C to a clear solution. In another beaker 2 mmol (117 mg) NaCl and 1 mmol (177 mg) PdCl2 in 20 ml of double distilled water was stirred at 45–50 °C for 2 h to a brown solution containing Na2[PdCl4]. The solution of sodium salt of alanine was dropletly added to a stirred solution of Na2[PdCl4]. Then the mixture was stirred for 1 h at 45–50 °C during which the color of the reaction mixture changed from brown to orange. It was then cooled and filtered. To the filtrate, a solution of 1 mmol (0.1 ml) acac and 1 mmol (40 mg) NaOH, in 10 ml water–ethanol mixture (1
:
1 v/v) was added with constant stirring. The color of reaction mixture changed from orange to yellow. It was stirred for 30 min and then filtered and concentrated to 5 ml on a water bath at 40 °C. Diffusion of acetone into the filtrate results in yellow microcrystal's after three days. The desired product was collected by filtration, washed with acetone and dried at 45 °C. Yield: 116 mg (55%) and decomposes at 214–218 °C. Elemental analysis calculated for C8H13NO4Pd (293): C, 32.76; H, 4.44; N, 4.78%. Found: C, 32.70; H, 4.39; N, 4.70%. 1H NMR (250 MHz, DMSO-d6, ppm, s = singlet, d = doublet, sb = singlet broad and m = multiplet): 1.194 (d, 3H), 2.198 (s, 6H), 3.233 (m, 1H), 4.582 (sb, 1H), 5.198 (sb, 1H) and 5.588 (s, 1H) (Fig. 1a and S1†). Solid state FT-IR spectroscopy have shown three characteristic bands at 3233, 1639 and 1549 cm−1 assigned to νN–H, νC–O (of amino acid) and νC–O (of acac)25 stretching modes respectively. Electronic spectra exhibit two bands at 233 nm (log
ε = 3.33) and 311 nm (log
ε = 3.2). These bands may be assigned to intraligand π → π* and n → π* transitions.8,26 Molar conductivity, ΛM (1 × 10−3 M, H2O) 16 (nonelectrolyte).27 ESI-MS (m/z, H2O): 294.13, [M − H]+.
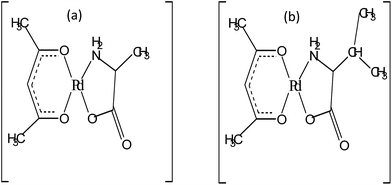 |
| Fig. 1 Proposed structures of (a) [Pd(acac)(Ala)] and (b) [Pd(acac)(Val)]. | |
Synthesis of acetylacetonato-DL-valinopalladium(II), [Pd(acac)(Val)]
This complex was synthesized and recrystallized by following the method as described for [Pd(acac)(Ala)] complex except that valine (Val) (117 mg, 1 mmol) was used instead of alanine. The yield of the complex was 183 mg (57%) and decomposes at 218–222 °C. Elemental analysis calculated for C10H17NO4Pd (321): C, 37.38; H, 5.29; N, 4.36%. Found: C, 37.33; H, 5.20; N, 4.40%. 1H NMR (250 MHz, DMSO-d6, ppm, s = singlet, d = doublet, sb = singlet broad and m = multiplet): 1.370 (m, 6H), 1.909 (d, 1H), 2.112 (s, 6H), 3.075 (m, 1H), 4.374 (sb, 1H), 5.130 (sb, 1H) and 5.519 (s, 1H) (Fig. 1b and S2†). Solid state FT-IR spectroscopy have shown three characteristic bands at 3223, 1619 and 1550 cm−1 assigned to νN–H, νC–O (of amino acid) and νC–O (of acac)25 stretching modes respectively. Electronic spectra exhibit two bands at 225 (log
ε = 3.46) and 320 (log
ε = 2.57) which may be assigned to intraligand π → π* and n → π* transitions.2,26 Molar conductivity, ΛM (1 × 10−3 M, H2O) 17 (nonelectrolyte).27 ESI-MS (m/z, H2O): 322.53, [M − H]+.
Spectrophotometry studies
The stock solutions of DNA (4 mg ml−1) or BSA (2 mg ml−1) were freshly prepared in Tris–HCl buffer by gentle stirring at 4 °C until homogenous. Also, stock solution of each of the Pd(II) complexes (10−4 M) were prepared in the same buffer by gentle stirring and heating at 35 °C. The concentration of DNA and BSA solutions was determined from its absorption intensities using reported molar extinction coefficient of DNA, 6600 M−1 cm−1 at 260 nm and BSA, 43
800 M−1 cm−1 at 280 nm.2,28,29 A solution of DNA in Tris–HCl buffer gave a ratio of UV-Vis absorbance at 260 nm and 280 nm (A260/A280) of 1.7–1.9, indicating that DNA was sufficiently free of protein.30 All experiments were performed separately at 300 K and repeated three times for each metal complex. Two experiments were carried out to investigate the interactions of the Pd(II) complexes with DNA and or BSA using electronic absorption spectroscopy in the UV-Vis region.
First. A fixed concentration of DNA (25 μl stock solution + 1.975 ml Tris–HCl buffer, 0.18 mM) and or BSA (100 μl stock solution + 1.9 ml Tris–HCl buffer, 0.006 mM) were titrated with each of the Pd(II) complexes (0.000–0.047 mM for DNA and 0.000–0.042 mM for BSA) at 300 K. In order to equilibrate, solutions of DNA–Pd or BSA–Pd(II) complexes were incubated for 5 min before spectral measurements. The absorption spectra were recorded between 220 and 350 nm after addition of each Pd(II) complex fractions to DNA or BSA solutions. Addition of the Pd(II) complex were continued until no changes were observed in the absorption spectra. The absorption data were analyzed using eqn (1).31 |
1/A − A0 = 1/A∞ − A0 + 1/K[A∞ − A0] × 1/[metal complex]
| (1) |
where A0 is initial absorption of DNA or BSA at 260 nm and 280 nm respectively in the absence of metal complex. A∞ is the final absorption of the ligated DNA or BSA (i.e. when all binding sites on DNA or BSA were occupied by the desired metal complex or while increasing the concentration of metal complex, no further changes in the intensity of absorption spectra were observed), and A is the recorded absorption at various concentrations of metal complex. The plot of 1/(A − A0) vs. 1/[metal complex] is linear and the binding constant (Kb) can be calculated from the ratio of the intercept to the slope.In the above experiments, selected spectra were overlaid to see if there is any isosbestic points and or hypo-, hyper-, hypso- and batho-chromic shifts.
Second. Unfolding or conformational changes of DNA or BSA incubated with each of the above mentioned metal complexes allowed us to observe modifications in the structure of DNA or protein and binding affinities of these complexes.32,33 In these experiments, a constant parameter, i.e. the concentration of each metal complex at midpoint of transition of DNA or BSA from native to unfolded, L1/2, can be determine.3,34 It was done by looking at the changes in the UV-Vis absorption spectrum of DNA solution at 260 nm or BSA solution at 280 nm upon addition of each Pd(II) complex. In these studies, the sample cell was filed with 1.8 ml of 0.2 mM DNA solution (1.775 ml Tris-buffer + 0.025 ml stock solution of DNA) or 1.8 ml of 0.007 mM BSA solution (1.7 ml Tris-buffer + 0.1 ml stock solution of BSA). However reference cell was filled with 1.8 ml of Tris-buffer only. Then, both cells were kept at constant temperature of 300 K or 310 K and 10 μl stock solution of any Pd(II) complex was added to both cells. After 5 min incubation, the UV-Vis absorption was recorded at 260 nm for DNA or 280 nm for BSA (denoted Am) also at 640 nm (denoted A640).35 Addition of Pd(II) complex to both cells was continued until no further changes in the absorption readings were observed. Thus the data obtained from this experiment are: Am, A640, [L]t, a = V2/V1 (where, a is dilution coefficient, V2 is total volume of solution in the sample cell after each addition of Pd(II) complex, V1 is initial volume i.e. 1.8 ml) and [L]t is total concentration of Pd(II) complex in each time of addition. On plotting a(Am − A640) against [L]t, the concentration of each metal complex at midpoint of transition, L1/2, could be deduced for the two temperatures 300 K and 310 K separately.
Spectrofluorimetry studies
The modes of interaction between DNA or BSA and the above synthesized palladium(II) complexes were investigated by taking help of fluorescence technique. In these experiments, excitation wave lengths of 471 nm for DNA and 280 nm for BSA were selected. The emission spectra were recorded between 525 to 725 nm for DNA and 300 to 500 nm for BSA. The band slits were set at 5 nm for DNA or BSA in both excitation and emission. Measurements were made using a 1 cm path length fluorescence cuvette. The maximum fluorescence intensity was used in order to calculate the thermodynamic parameters. In the interaction studies between above Pd(II) complexes and DNA or BSA, following procedures were followed.
DNA interaction studies
The fluorescence intensities of the solutions of either [Pd(acac)(Ala)] or [Pd(acac)(Val)] complexes and calf thymus DNA have been checked and their emission intensities found to be very small and negligible. Thus ethidium bromide (EB) was used to probe the course of interaction. In these experiment, in a quartz cell containing 1 ml Tris–HCl buffer of pH = 7, 25 μl of DNA (stock solution) and 68 μl aqueous EB (0.023 mg ml−1) were added and the volume adjusted to 2.5 ml by the same buffer. In this solution mixture, the fluorescence of EB enhanced about 50 fold at ∼600 nm as it intercalates between base pairs of DNA.34,36 Then effects of each Pd(II) complex on the emission intensity of interacted DNA–EB were studied by adding increasing concentrations of each metal complex (10, 20, 30, 40, and 50 μl of the stock solution). After each addition step and equilibration for 5 min, an emission spectrum was recorded between 525 to 725 nm. At the end of experiment, all spectra overlaid to observe the changes in their emission intensities. This experiment was performed separately for each sample at three different temperatures of 293, 300 and 310 K. Using the data pertaining to each metal complex and eqn (2), the Stern–Volmer quenching constant, Ksv (a measure of the effectiveness of the metal complex as quencher) was determined.37,38 |
F0/F = 1 + Kqτ0[Q] = 1 + Ksv[Q]
| (2) |
where, F0 and F are the fluorescence intensities of DNA–EB in the absence and presence of various concentration of quencher i.e. metal complex, [Q] is total concentration of Pd(II) complex, τ0 is average fluorescence lifetime of fluorophore (in this case EB) in the absence of quencher and Kq is the apparent bimolecular quenching constant which equals to Ksv/τ0.39
Thus the slope of the plot F0/F versus [Q] gives the value of Ksv for each sample. Also, the binding constant (Kb) and the number of binding sites (n) on DNA were calculated according to the eqn (3).
|
log(F0 − F/F) = log Kb + n log[Q]
| (3) |
here, from the plot of log(
F0 −
F/
F)
versus log[Q], the value of
n and
Kb could be calculated.
36 Knowing
Kb, thermodynamic parameters can be evaluated using
eqn (4), Van't Hoff equation.
37 |
−ln Kb = −ΔH°/RT + ΔS°/R
| (4) |
where
Kb is binding constant analogous to effective quenching constant (
Kq) at corresponding temperature,
R represents the gas constant,
T is the absolute temperature (K) and Δ
H° and Δ
S° are enthalpy and entropy changes respectively. These two later parameters were obtained separately at 293, 300 and 310 K by plotting ln
Kb versus 1/
T. From these plots, the values of Δ
H° and Δ
S° were obtained, from the slope (−Δ
H°/
R) and intercept on
y axis (Δ
S°/
R) respectively. Finally, the Gibbs free energy changes (Δ
G°) in the interaction between each Pd(
II) complex and DNA–EtBr can be calculated according to the
eqn (5).
40 |
ln Kb = −ΔG°/RT
| (5) |
Viscometric measurements
The viscosity of the CT-DNA solution (200 μM) were measured using SCHOT AVS 450 viscometer in the absence and presence of the metal complexes ([complex]/[DNA] = 0, 0.05, 0.1, 0.15, 0.2, 0.25, 0.3, 0.35, 0.4, 0.45 and 0.5) in Tris–HCl buffer (pH = 7) in the thermostated water bath at 27 °C. Flow time was measured with a digital stopwatch and each sample was measured three times, then an average flow time was calculated. Data were presented as (η/η0)1/3 versus [complex]/[DNA], where η0 and η represent the specific viscosity of CT-DNA solution in the absence and presence of the complexes, respectively. Viscosity values were calculated from the observed flow time of DNA containing complexes (t) corrected for the flow time of buffer alone (t0), according to the relation η = (t − t0)/t0.
BSA interaction studies
Procedures followed to study interaction between BSA and each metal complex was the same as above procedures for DNA interaction. The only difference is, no need of using fluorophore (EB) as in the case of DNA interaction studies. This is because BSA is intrinsically fluorescence active which comes from tryptophan, tyrosine and phenylalanine residues. This activity is very sensitive to its microenvironment, and variety of molecular interactions can diminish the fluorescence intensity of proteins, which is called quenching.29,33,39,41
Cytotoxic studies
Cell culture. The chronic myelogenous leukemia cell line, K562 (obtained from National Cell Bank of Iran [NCBI]-Pasteur Institute of Iran), was selected for study of Pd(II) complexes cytotoxicity. Cells were grown in the RPMI medium (RPMI, Sigma). The medium was supplemented with L-glutamine (2 mM), streptomycin and penicillin (5 μg ml−1) and 10% heat-inactivated fetal calf serum, at 37 °C under a 5% CO2/95% air atmosphere.
Cell proliferation assay. The above Pd(II) complexes inhibited the growth of chronic myelogenous leukemia cell line, K562. This growth inhibition was measured by means of MTT (3-(4,5-dimethylthiazol-2-yl)-2,5-diphenyltetrazolium bromide) assay.23 The cleavage and conversion of the soluble yellowish MTT to the insoluble purple formazan by active mitochondrial dehydrogenase of living cells has been used to develop an assay system alternative to other assays for measurement of cell proliferation. Harvested cells were seeded into a 96-well plate (1 × 104 cells per ml) with varying concentrations of sterilized Pd(II) complexes (0–250 μM) and incubated for 24 h. Four hours to the end of incubations, 25 μl of MTT solution (5 mg ml−1 in PBS) was added to each well containing fresh and cultured medium. At the end, the insoluble formazan produced was dissolved in the solution containing 10% SDS and 50% DMF (left in darkness for 2 h at 37 °C) and optical density (OD) was read against reagent blank with multi-well scanning spectrophotometer (ELISA reader, Model Expert 96, Asys Hitech, Austria) at the wavelength of 570 nm. Absorbance is a function of concentration of the converted dye. The OD value of study groups was divided by the OD value of the untreated control and presented as the percentage of control (as 100%).7,24
Computational details
In the present study, all the calculations were performed with the Gaussian 03 software.42 The geometry optimizations for all structures were carried out using density function theory B3LYP.43 H, C, O, and N atoms were described with 6-311G* pople's basis set. For a description of the Pd atom LANL2DZ basis set were utilized. Frequency calculations were done to verify that the geometries were minima on the potential energy surface. Also, the energy gaps have been computed as the energy difference between the LUMO and the HOMO.
Molecular docking
The 3D X-ray structures of BSA (encoded 3V03) and DNA (encoded 453D) were obtained from the Brookhaven Protein Data Bank (http://www.pdb.org). The optimized complexes were considered for docking study. So, the Auto Dock 4.2 suite molecular-docking tool was applied and the methodology was follow out (Gowthaman et al., 2008).44,45 Bonding site was chosen suitable enough for BSA or DNA to allow the ligands to rotate freely. The binding position and bound conformation of the macromolecules with complexes, and the rough estimate of their interactions were examined with the Auto Dock results. To analyze the mode of binding docked conformation at lowest binding energy was selected.3,46,47
Results and discussion
Neutral complexes [Pd(acac)(Ala)] and [Pd(acac)(Val)] (where, acac, Ala and Val are anions of acetylacetone, alanine and valine respectively) were synthesized in water by sequential addition of sodium salt of Ala or Val and acac to Na2[PdCl4] in molar ratio of 1
:
1
:
1. These complexes are sufficiently soluble in water which is an important first step for possible applications in biological systems. Satisfactory results of elemental analysis and spectral studies revealed that the complexes were of good purity. The analytical data of complexes are given in the Experimental section. From the spectral (FT-IR, UV-Vis, 1H NMR and ESI-MS) and non-spectral (conductivity measurements and elemental analysis) characterization data, the proposed structures of the complexes are shown in Fig. 1. Here, a bidentate coordination of the ligands can be proposed for this type compounds. Both complexes are air-stable at room temperature. The stability of the complexes in aqueous medium (5 × 10−4 M) was analyzed by comparing their UV-Vis spectra of 0-, 24- and 48 h intervals. The spectra of the complexes were found to be similar, indicating that they are stable in solution, retaining their original structures. Furthermore, the ESI-MS studies confirmed the stability of alanine and valine complexes in aqueous medium. The complexes were isolated as yellow solids and our attempts to make single crystal suitable for X-ray crystallography were unsuccessful.
Detailed binding (n and Kapp) and thermodynamic (ΔG°, ΔH° and ΔS°) parameters, unfolding abilities (L1/2), cytotoxic activities (Cc50) and molecular docking of the two complexes to CT-DNA and BSA are discussed here.
Cytotoxicity tests of the Pd(II) amino acid complexes
The in vitro anti-tumor property of the acetylacetonatopalladium(II) amino acid complexes were studied by testing against human tumor cell line K562. In these studies, different concentrations of the Pd(II) complexes ranging from 0 to 100 μM of stock solutions were used in the culture medium of the cell line for 24 h (Fig. 2). The 50% cytotoxic concentration (Cc50) of [Pd(acac)(Ala)] and [Pd(acac)(Val)] complexes after 24 h incubation time are 38.59 μM and 29.09 μM respectively. As shown in Fig. 2, cells growing after 24 h was significantly reduced in the presence of various concentrations of the Pd(II) complexes and the Pd(II) complexes produce a dose–response suppression on growing of K562 leukemia cell line. Fig. 2 also showed that [Pd(acac)(Val)] is more cytotoxic as compared to that of [Pd(acac)(Ala)] complex. Furthermore, Cc50 value of cis-diamminedichloroplatinum(II), the well-known anti-cancer drug under the above same experimental conditions was measured. This value (154 μM) is much higher as compared to the Cc50 values of the above two Pd(II) complexes. It can be noticed that the presence of amino acids in the structure of our complexes as well as their higher water solubility (∼60 mg ml−1) as compared to cisplatin (∼2.53 mg ml−1)48 might have influence on anti-tumor activities of these complexes on K562 cell line.
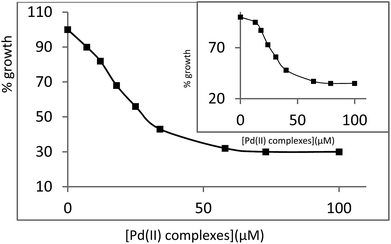 |
| Fig. 2 The growth suppression activity of the [Pd(acac)(Val)] and inset [Pd(acac)(Ala)] complexes on K562 cell line were assessed using MTT assay. The tumour cells were incubated with varying concentrations of the complexes ranging from 0–100 μM for 24 h. | |
The cytotoxicity results showed the possibility of antitumor properties of the alanine and valine complexes. However, numerous experiments have demonstrated DNA is the primary intracellular target of anticancer drugs17 and most drugs are carried by proteins like HSA or BSA in the body fluids.18 Thus DNA binding studies help us to understand the mechanism by which these agents may interact with DNA, causing its damage and blocking the division of cancer cells. However, interaction of the Pd(II) complexes with proteins such as BSA that is found in biological systems, may reflect the side effects of these agents. Thus our discussions are followed by detailed DNA-/BSA-interaction studies to predict their possible interaction mechanisms.
Electronic absorption experiments
Ultraviolet visible spectroscopy has been universally employed to determine binding strength and binding modes of small molecules to DNA double helix or proteins.49 A fixed amount of CT-DNA (0.18 mM) or BSA (0.006 mM) was titrated with increasing concentrations of [Pd(acac)(Ala)] and [Pd(acac)(Val)] complexes (0.000–0.047 mM for DNA and 0.000–0.042 mM for BSA) at 300 K. The decrease in the absorption maxima with some blue shift (2–4 nm) of DNA (260 nm) and BSA (280 nm) are shown in Fig. 3. These observations indicate the interaction between both Pd(II) complexes with CT-DNA and BSA molecules.50 There were no changes in the absorption spectra if more metal complexes were injected to the systems, suggesting saturation of CT-DNA and BSA binding sites and no further interaction occur. However, it is well known that dynamic quenching only affects the excited state of the fluorophore and does not change the absorption spectrum, while the formation of non-fluorescence ground-state complex between fluorophore and quencher, induced the change in the absorption spectrum of the fluorophore (Fig. 3c and d). Thus the possible quenching mechanism of BSA by Pd(II) complex was a static quenching process.51 Also each system show two isosbestic points or points of constant absorbance which are given in Table 1.
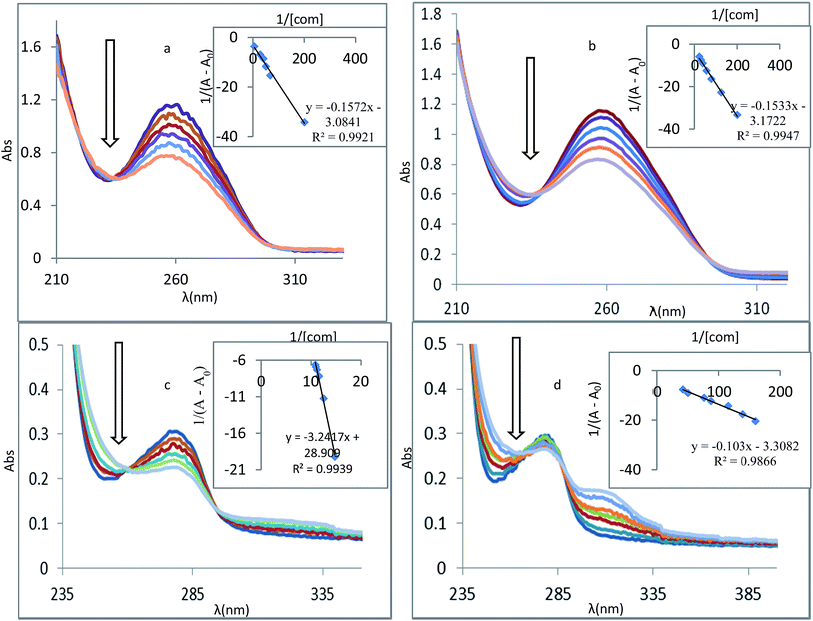 |
| Fig. 3 Effect of [Pd(acac)(Ala)] and [Pd(acac)(Val)] on the absorption spectrum of CT-DNA (a & b) and BSA (c & d) respectively. The inset plots give their corresponding Kapp at 300 K. | |
Table 1 Apparent binding constants, positions of the isosbestic points and midpoint transition constants for the interaction between [Pd(acac)(Ala)] and [Pd(acac)(Val)] complexes with calf thymus DNA and bovine serum albumin (BSA)
Compound |
Kappa (M−1) at 300 K |
Isosbestic points (λ, nm) at 300 K |
T (K) |
L1/2b (mM) |
CT-DNA |
BSA |
CT-DNA |
BSA |
CT-DNA |
BSA |
Kapp is apparent association constant. Concentration of Pd(II) complex needed to induce 50% conformational changes in native CT-DNA or BSA. |
[Pd(acac)(Ala)] |
1.96 × 104 |
8.91 × 103 |
240 & 295 |
255 & 295 |
300 K |
0.018 |
0.021 |
0.019 |
0.023 |
[Pd(acac)(Val)] |
2.07 × 104 |
3.21 × 104 |
240 & 295 |
255 & 295 |
310 K |
0.012 |
0.02 |
0.016 |
0.022 |
The presence of these isosbestic points suggest that:52 (i) there is an equilibrium between DNA/BSA bound and free forms of metal complexes and these two forms have same absorptions at the isosbestic points (ii) good indicating of there being only two absorbing species in solutions, and final spectrum corresponding to that of interacted CT-DNA–Pd or BSA–Pd complexes and (iii) two isosbestic points indicates that reaction proceeds without side reactions (such as hydrolysis, etc.). This later point is supported by considering structure of Pd(II) complexes (Fig. 1) in which both ligands (acac and amino acids) coordinated to Pd(II) center as bidentate chelates and do not detached easily. Similar observations have been seen in the interaction of amino acid metal complexes with calf thymus DNA.53 To determine quantitatively the CT-DNA or BSA binding strengths of the Pd(II) complexes, binding constant were calculated by eqn (1). In plot of 1/(A − A0) vs. 1/[metal complex], apparent binding constant, Kapp, is given from the ratio of y intercept to slope which are given in Table 1. This Kapp in the interaction of these complexes with CT-DNA is lower than that of classical intercalator ethidium bromide, (EB), (1.4 × 106 M−1).54 These results suggest the binding modes of these complexes to CT-DNA, should be non-intercalation and differs from that of EB (intercalation). Moreover, binding with DNA via intercalation usually results in hypochromism and bathochromism of the absorption. This is due to a consequence of strong π–π* stacking interaction between planar aromatic moiety of the complexes and DNA base pairs.54 These results are in agreement with the proposed structures of the complexes (Fig. 1) having no planar aromatic moieties to act as intercalator.
Determination of midpoint transition, L1/2, as an important constant
The profiles of conformational changes in the structure of native CT-DNA or BSA induced by [Pd(acac)(Ala)] and [Pd(acac)(Val)] complexes are shown in Fig. 4 and 5. These conformational changes occurred in such a manner that the absorbing groups of DNA(purine and pyrimidine bases) and BSA (mainly tryptophan residues) get hidden from UV light, results a decrease in the intensity of the absorption peak with increase in the concentration of Pd(II) complexes at the same wavelength.3,40 Thus unloaded (native) and loaded (interacted) BSA or CT-DNA by any of Pd(II) complexes have different conformations and transition between these two conformations is discussed under the above title.
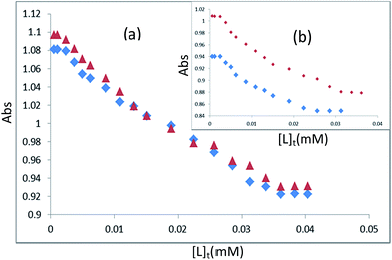 |
| Fig. 4 The changes of absorbance of DNA at λmax = 260 nm due to increasing the concentration of [Pd(acac)(Ala)] (a), and [Pd(acac)(Vla)] (b), complexes, at temperatures of 300 K ( ) and 310 K ( ). | |
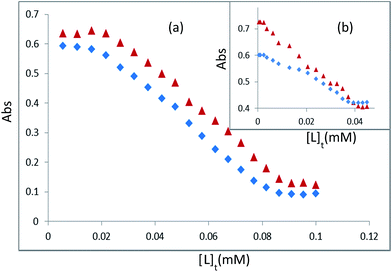 |
| Fig. 5 The changes of absorbance of BSA at λmax = 280 nm due to increasing the concentration of [Pd(acac)(Ala)] (a), and [Pd(acac)(Vla)] (b), complexes, at temperatures of 300 K ( ) and 310 K ( ). | |
As Fig. 4 and 5 shows, the concentration of both metal complexes needed to interact with native CT-DNA or BSA causing 50% conformational changes (L1/2) are very low (see Table 1). It means that, complexes can interact with CT-DNA or BSA at their low concentrations and if used as anti-cancer agent, lower doses will be needed. In other words, patients treated with lower doses drugs may suffer fewer side effects in comparison with those exposed to higher doses.55 Looking at L1/2 values given in Table 1, following points could be deduced:
(i) L1/2 values of CT-DNA are lower than those of BSA indicating that complexes show more interaction affinities towards DNA in comparison with BSA. This is expected because there may be more available binding sites on DNA as compared with those of BSA. (ii) L1/2 values of CT-DNA at 300 K is lower than those of 310 K. The results indicated that reaction between the Pd(II) complexes with CT-DNA may be exothermic and (iii) in the interaction between valine complex with CT-DNA, L1/2 values in both temperatures of 300 and 310 K, are lower than those for alanine complex. These suggest that interaction affinity of valine complex is more than that of alanine complex.
Fluorescence experiments with calf thymus DNA
The above electronic absorption spectroscopy study point out that the new Pd(II) complexes efficiently interact with CT-DNA. Further, it is well known that the interaction of transition metal complexes with DNA takes place via both covalent and/or non-covalent interaction.56 In the case of covalent binding, there should be labile ligand (generally monodentate) coordinated to the central metal ion of the complex to be replaced by a nitrogen bases of DNA such as guanine N7.57 This type of binding is not likely to be responsible for biological activity of complexes shown in Fig. 1 owning to presence of two bidentate chelating ligands tightly coordinated to Pd(II) center. Non-covalent DNA interaction includes intercalation, electrostatic and groove binding of metal complexes to DNA helix. Among these main binding interactions, groove binding is the most likely mode, since the alanine and valine complexes neither have planer aromatic moieties nor charge to be interacted with CT-DNA via intercalation or electrostatic binding respectively. The groove binding mode has been confirmed by following experiments:
Competitive studies with ethidium bromide. The binding mode of alanine and valine complexes to CT-DNA has been analyzed by quenching emission intensity of ethidium bromide (EB). It is well known that EB emits intense fluorescence in presence of CT-DNA due to its nonspecific intercalation between the adjacent CT-DNA base pairs. The addition of other compounds competitive with EB will induce displacement of bound EB and a decrease in fluorescence intensity.58 Hence competitive ethidium bromide binding studies can provide indirect evidence for the DNA-binding mode.59,60 Fig. 6 illustrate the fluorescence emission spectra of CT-DNA–EB system with increasing concentration of Pd(II) complexes. The experiment revealed that addition of the alanine or valine complexes to DNA bound EB does not cause releasing EB molecules, while the emission intensity decrease steadily. Because if EB releases, a weak intensity spectrum due to free EB near the base line should appear which has not been observed (see Fig. 6). This implies that the two probes, the complexes and EB, bind with DNA independently and binding of Pd(II) complexes does not affect the binding of the EB. However interaction of Pd(II) complex with DNA bound EB may bring about conformational changes leading to displacement of some EB molecules from hydrophobic to hydrophilic moieties causing diminuendo in emission intensities are possible.50 Thus, the experiment confirmed that interaction between CT-DNA and the neutral alanine and valine complexes is not through intercalation and the possible mode of binding seems to be groove interaction.
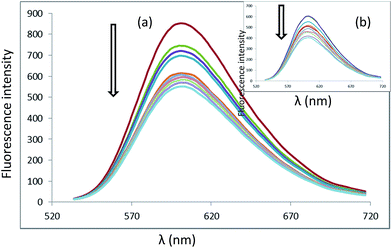 |
| Fig. 6 The emission spectra of DNA–EB due to increasing the concentration of [Pd(acac)(Ala)] (a), and [Pd(acac)(Val)] (b), complexes. | |
Stern–Volmer quenching constants. Titration of CT-DNA–EB with various amount of Pd(II) complexes was quantified by measuring the fluorescence quenching of the DNA–EB solution. In fact, fluorescence quenching refers to any process that decreases the fluorescence intensity of a sample.61 It may cause by a variety of molecular interactions such as excited state reactions, energy transfers, formation of non-fluorescent ground state complex (static quenching) and collision between the fluorophore and quencher (dynamic quenching).62 In dynamic quenching, collision occurs during the lifetime of the excited state, and as a result of this contact; fluorophore returns to the ground state without emission of a photon. The quenching rate constant expected to decrease with increasing temperature for static quenching. In contrast, the reversed effect was observed for the dynamic quenching.61,62 In order to clarify the quenching mechanism of CT-DNA–EB by Pd(II) complexes, the Stern–Volmer eqn (2) is used to analyze the fluorescence quenching data. This equation has been explained in Experimental section. The Ksv values for each Pd(II) complex are obtained from the slope of the plot F0/F versus [Q] at three temperatures. According to the classified Stern–Volmer equation, the quenching plot illustrate that the quenching of EB bound to DNA by complex is in good agreement with the linear Stern–Volmer equation.63 By using Fig. 7, the values of Ksv and Kq at different temperatures were calculated and listed in Table 2. From Table 2, the results show that the values of Ksv and Kq decrease with increasing of the temperature and the values of Kq is greater than 2.0 × 1010 L mol−1 s−1, which indicates that the probable quenching mechanism of DNA–EB solution is a static quenching.64,65
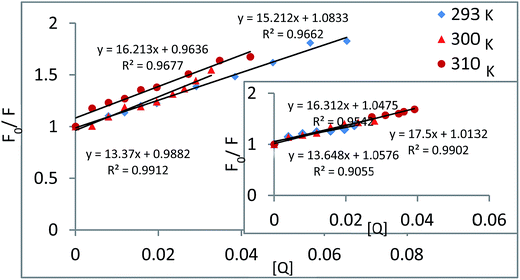 |
| Fig. 7 The Stern–Volmer plots of the fluorescence titration of DNA–EB with [Pd(acac)(Ala)] and inset with [Pd(acac)(Val)], at difference temperatures. | |
Table 2 The Ksv and Kq values of the Pd(II) complexes in the interaction with CT-DNA
Complex |
T (K) |
Ksv (103 M−1) |
Kq (1011 M−1 s−1) |
[Pd(acac)(Ala)] |
293 |
16.231 |
16.231 |
300 |
15.212 |
15.212 |
310 |
13.370 |
13.370 |
[Pd(acac)(Val)] |
293 |
17.500 |
17.500 |
300 |
16.312 |
16.312 |
310 |
13.648 |
13.648 |
Binding constant (Kb) and number of binding sites (n). When a small molecule such as a transition metal complex interact independently to a set of equivalent binding sites on a macromolecule like a DNA, the titration data obtained from the fluorescence experiment can be used to calculate the binding constant and number of binding sites using eqn (3).66 Here Kb and n represent the binding constant and number of binding sites of Pd(II) complex to CT-DNA, respectively. Based on eqn (2), the values of Kb and n were determined from the intercept and slope of (log[(F0 − F)/F] versus log[Q], (Fig. 8)), and the corresponding calculated results are listed in Table 3. Data on Table 3 shows that the values of Kb decrease with increasing of temperature, indicating that the stability of DNA–EB complex reduce with the temperature rising. The number of binding sites n are approximately equal unity, indicating that there is one independent class of binding sites on DNA for Pd(II) complex. Similar results were obtained for reported metal complexes.36,67 However, the values of n and Kb (Fig. 8) decreasing with rise in temperature, which demonstrate that the complex formation between CT-DNA–EB and Pd(II) complexes can be regarded to be exothermic procedure.36,68 Finally the values of Kb has similar trend to the Kapp values (Table 1) in both complexes and values of Kb represent the lower binding affinity of [Pd(acac)(Ala)] to DNA than [Pd(acac)(Val)] complex. This noticeable difference between alanine and valine complexes in binding affinity, suggests that the –CH(CH3)2 group on valine complex play an important role in the Pd(II) complex–DNA interaction.
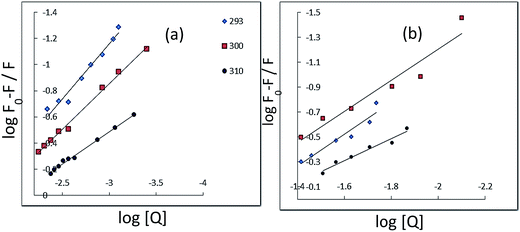 |
| Fig. 8 Plot of log(F0 − F)/F versus log[Q] of CT-DNA interacted with [Pd(acac)(Ala)] (a), and [Pd(acac)(Val)] (b), at difference temperatures. | |
Table 3 The binding constant (Kb) and number of binding sites (n) values of Pd(II) complexes in the interaction with CT-DNA
Complex |
T (K) |
Kb (103 M−1) |
n |
[Pd(acac)(Ala)] |
293 |
22.407 |
1.235 |
300 |
15.642 |
1.116 |
310 |
11.585 |
0.974 |
[Pd(acac)(Val)] |
293 |
27.977 |
1.280 |
300 |
19.485 |
1.248 |
310 |
14.828 |
0.963 |
Thermodynamic parameters and binding mode. When binding constant (Kb) depends on temperature, thermodynamic parameters, free energy change (ΔG), enthalpy change (ΔH), and entropy change (ΔS) of interaction, are essential to interpret the binding mode of CT-DNA with Pd(II) complexes.69 The main force acting between a small molecule and macromolecule may include hydrogen bonds, van der Waals, electrostatic and hydrophobic interactions.70 When enthalpy change does not vary significantly in the temperature rang studied, both ΔH° and ΔS° can be determined from Van't Hoff equation (eqn (4)). In eqn (4), Kb is analogous to the effective quenching constant (Kq) at the corresponding temperature. The temperatures used were 293, 300 and 310 K. Using eqn (4), (see Experimental section) the slope and y-intercept of the plot of ln
K versus 1/T, determine the ΔH° and ΔS° respectively (Fig. 9). Then the Gibbs free energy change (ΔG°) is determined by following eqn (5). Thus according to eqn (4) and (5), we could obtain the values of ΔH°, ΔS° and ΔG° and the results are shown in Table 4. Yang et al.71 correlate the magnitude and sign of thermodynamic parameters with various kinds of interactions that may take place between small molecules with macromolecules such as DNA, protein etc. They concluded: (i) ΔH > 0 and ΔS > 0, hydrophobic forces; (ii) ΔH < 0 and ΔS < 0, van der Waals forces and hydrogen binds; (iii) ΔH < 0 and ΔS > 0, electrostatic interactions. In addition, specific electrostatic interaction between ionic species in aqueous solution is also characterized by a positive ΔS and negative ΔH.
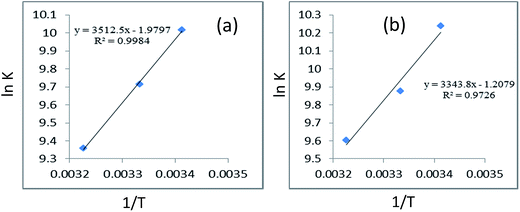 |
| Fig. 9 The Van't Hoff plot of [Pd(acac)(Ala)] (a), and [Pd(acac)(Val)] (b), with DNA–EB at difference temperatures. | |
Table 4 The thermodynamic value of Pd(II) complexes in DNA–EB interaction
Complex |
T |
ΔH (kJ mol−1) |
ΔS (kJ mol−1 K−1) |
ΔG (kJ mol−1) |
[Pd(Ala)(acac)] |
293 |
−29.202 |
−0.016 |
−24.401 |
300 |
−29.202 |
−0.016 |
−24.227 |
310 |
−29.202 |
−0.016 |
−24.117 |
[Pd(Val)(acac)] |
293 |
−27.800 |
−0.010 |
−24.942 |
300 |
−27.800 |
−0.010 |
−24.636 |
310 |
−27.800 |
−0.010 |
−24.753 |
Considering thermodynamic parameters given in Table 4, the binding forces are spontaneous because of the Gibbs free energy change value is negative.16 It is also demonstrated that both enthalpy and entropy changes are negative, suggesting the Pd(II) complexes were holded in DNA grooves via hydrogen and van der Waals binding. It could also be explained that, owing to the binding of complexes to DNA, some conformational changes are induced results to destacking of some base pairs, showed by the decrease in fluorescence emission intensity of DNA–EB upon addition of the Pd(II) complexes. These results are quite in agreement with proposed structures given in Fig. 1. As Fig. 1 shows, the carbonyl and one –NH2 groups of amino acids coordinated to Pd(II) center are susceptible for H-binding and –CH3 group of alanine and –CH(CH3)2 group of valine can interact via van der Waals forces in groove binding. Similar observations were made for [Pd3(C,N-(C6H4C(Cl)
NO)-4)6] complex.50
Viscosity measurements. To further clarify the nature of the interaction between the complexes and CT-DNA, viscosity measurements were carried out. In general, intercalating agents are expected to elongate the double helix of DNA and groove binding by partial and/or non-classical interaction typically cause less pronounced (positive or negative) or no change in DNA solution viscosity.72,73 The values of relative specific viscosity of DNA in absence and presence of complexes are plotted against r = [complex]/[DNA]. The relative viscosities of CT-DNA bound to valine and alanine Pd(II) complexes inapparently decreased with continues addition of the complexes (Fig. 10). These observations are consistent with groove binding suggested for the complexes and similar to the reported metal complexes.74
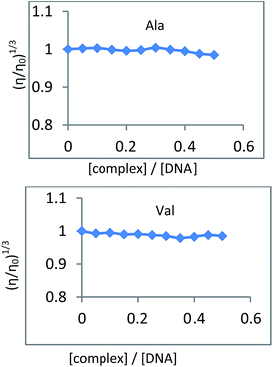 |
| Fig. 10 Effect of increasing amount of the alanine (Ala) and valine (Val) Pd(II) complexes on the relative viscosity of CT-DNA at 27 °C, [DNA] = 200 μM. The results are the mean of three independent experiments carried out under identical conditions. | |
Fluorescence experiments with bovine serum albumin (BSA)
Qualitative analysis of binding of Pd(II) complexes to BSA can be detected using fluorescence experiments. It is well known that among the three fluorophores namely, tryptophan, tyrosine and phenylalanine in BSA, the intrinsic fluorescence is mainly due to tryptophan alone.75 Change in the emission spectra of tryptophan are common in response to protein conformational transitions, subunit associations, substrate binding or denaturation.57 In case of latter, addition of non-fluorescent quencher to BSA solution causing enhancement in emission intensity may be due to denaturation of the protein which is because of exposure of the tryptophan residues to aqueous medium.76 However on increasing the concentration of our Pd(II) complexes, a progressive decrease in the fluorescence intensity of BSA solution was observed, accompanied with slight blue shift (Fig. 11). These observations suggest that the Pd(II) complexes do not denature the BSA and their interactions may perturb the native conformation of BSA leading to changes in tryptophan environments.77
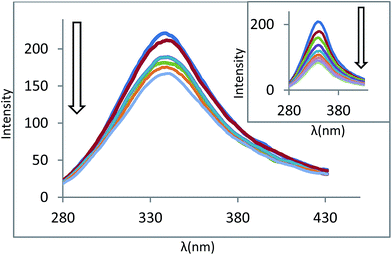 |
| Fig. 11 The emission spectrum of BSA due to increasing the concentration of [Pd(acac)(Ala)], inset [Pd(acac)(Val)], complexes. | |
The decrease in the emission intensity also suggest a shift of tryptophans of BSA into more hydrophobic environment that can be attributed to internalization of fluorophore residue towards the protein hydrophobic core due to the protein refolding or due to hydrophobic amino acid residues around tryptophan such as leucine (Leu), Tyr and phe, leading to a decrease in fluorescence intensity.16,76 In the titration of BSA with Pd(II) complexes, another noticeable point is that, at higher concentration of Pd(II) complexes, intensity of emission reaches a constant value, suggesting no further change in microenvironment of the tryptophan residues corresponding to the protein saturation point and formation of free Pd(II) complex.76 As we shall see in later part of this studies, number of binding sites for each Pd(II) complex per BSA molecule equal to unity. There is only one binding sites for each Pd(II) complex on BSA and once it gets occupied, no further changes in the emission spectrum were observed. It suggests that interaction between each neutral Pd(II) complex and BSA includes occupation of one binding site and probably there is no more interactions with other parts of BSA molecule causing structural damage and bring about side effect in the physiological systems.14 This is in fact the normal function of the protein BSA in the bloodstream. In order to understand quantitatively the magnitude of the complexes to quench the emission intensity of BSA (Fig. 11), Stern–Volmer eqn (2) can be used. A plot of F0/F against the concentration of alanine and or valine complexes resulted in liner plot (Fig. 12) and the Ksv value is obtained from the slope. The observed Ksv values indicate good BSA binding affinity of the two complexes whit valine complex exhibiting the higher protein binding ability (Table 5). The Kq values for alanine and valine complexes are 2.505 × 1012 M−1 s−1 and 2.900 × 1012 M−1 s−1, respectively. From the other side, Kq of various quenchers with biopolymer is 2.0 × 1010 L mol−1 s−1.78 Thus, calculated Kq by the protein quenching procedure is greater than the maximum scatter collision-quenching constant of biopolymers. This indicated that a static quenching mechanism is operating.79,80 Moreover, the curves F0/F versus [Q] are linear which support the static quenching (Fig. 12).
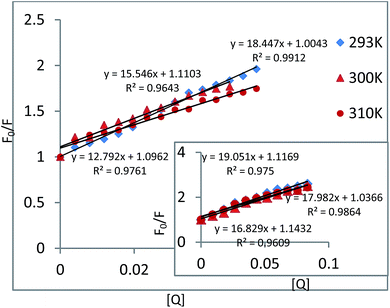 |
| Fig. 12 The Stern–Volmer plots of the fluorescence titration of BSA with [Pd(acac)(Ala)] and inset [Pd(acac)(Val)], at difference temperatures. | |
Table 5 The Ksv values of Pd(II) complexes in the interaction with BSA
Complex |
T (K) |
Ksv (103 M−1) |
Kq (1012 M−1 s−1) |
[Pd(acac)(Ala)] |
293 |
18.447 |
2.97 |
300 |
15.546 |
2.505 |
310 |
12.397 |
2.063 |
[Pd(acac)(Val)] |
293 |
19.051 |
3.072 |
300 |
17.982 |
2.900 |
310 |
16.828 |
2.714 |
The binding constant, Kb of quencher (in the present case alanine and valine complexes) with BSA and the number of binding sites, n, on BSA available for the [Pd(acac)(Ala)] and [Pd(acac)(Val)] complexes were calculated using eqn (3). The values of Kb can be determined from the slope of plot log(F0 − F/F) versus log[Q] (Fig. 13) and Table 6. The results are consistent with greater binding affinity of valine complex as compared to that of alanine complex. Similar observations were made for reported metal complex.81 The value of n at the experimental temperatures approximately equal to 1, which indicates that there is just single binding site in BSA for each Pd(II) complex. As the complexes are natural, they most likely binds to hydrophobic pocket in sub-domain IIA, that is to say, Trp-212 is near or within the binding site.33
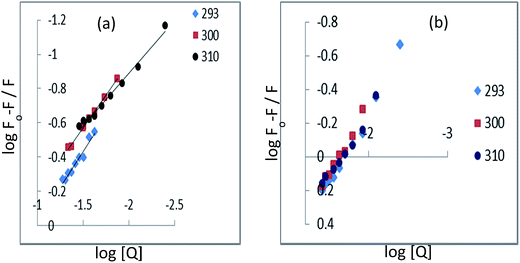 |
| Fig. 13 Plot of log(F0 − F)/F versus log[Q] of BSA interacted with [Pd(acac)(Ala)] (a), and [Pd(acac)(Val)] (b), at difference temperatures. | |
Table 6 The binding constant (Kb) and number of binding sites (n) values of Pd(II) complexes in the interaction with BSA
Complex |
T (K) |
Kb (103 M−1) |
n |
[Pd(acac)(Ala)] |
293 |
23.675 |
1.010 |
300 |
19.462 |
1.007 |
310 |
13.570 |
1.001 |
[Pd(acac)(Val)] |
293 |
28.112 |
1.123 |
300 |
23.604 |
1.016 |
310 |
15.524 |
1.008 |
From the binding constant Kb and eqn (4) and (5), thermodynamic parameters at corresponding temperatures have been evaluated (Table 7) and (Fig. 14). These parameters allow us to conclude the binding mode between Pd(II) complexes and BSA molecule.82 The negative ΔH° and ΔS° values are regarded as an evidence for hydrogen and van der Waals binding. Therefore, these two forces may play main role in holding Pd(II) complexes in hydrophobic pocket located in sub-domain IIA of BSA molecule.83 The negative values of ΔG° also supported the UV-Vis results of free energy changes and indicated the spontaneity of Pd(II) complexes–BSA binding.
Table 7 The thermodynamic values of Pd(II) complexes in BSA interaction
Complex |
T |
ΔH (kJ mol−1) |
ΔS (kJ mol−1 K−1) |
ΔG (kJ mol−1) |
[Pd(Ala)(acac)] |
293 |
−24.902 |
−0.001 |
−24.535 |
300 |
−24.902 |
−0.001 |
−24.633 |
310 |
−24.902 |
−0.001 |
−24.524 |
[Pd(Val)(acac)] |
293 |
−26.718 |
−0.005 |
−24.954 |
300 |
−26.718 |
−0.005 |
−25.114 |
310 |
−26.718 |
−0.005 |
−24.871 |
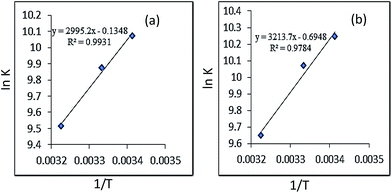 |
| Fig. 14 The Van't Hoff plot of [Pd(acac)(Ala)] (a), and [Pd(acac)(Val)] (b), with BSA at difference temperatures. | |
Geometry optimization and energies of the complexes
In the present work all optimized geometries were performed using the density functional theory method and 6-311G*+LANL2DZ mixed basis set are shown in Fig. 15 and some geometrical parameters of compounds were tabulated in Table 8. In order to check the validity of the applied method, X-ray diffraction data of similar complexes were used to compare the optimized structures of the complexes.8,26 The agreement between the computed structure and X-ray diffraction data was very good. In addition Tables 9 and 10 compares the calculated 1H NMR chemical shifts of Pd(II) complexes with the corresponding experimental data in DMSO-d6 solvent. As observed, there is good agreement between the theoretical and experimental data.
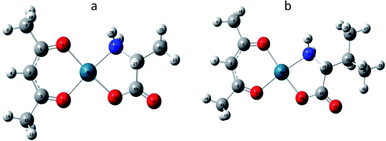 |
| Fig. 15 The optimized structure of [Pd(Ala)(acac)] (a), [Pd(Val)(acac)] (b). Carbon: gray, oxygen: red; nitrogen: blue; palladium: green jade. | |
Table 8 Selected bond lengths (Å) and bond angles (°) for the Pd(II) complexes at B3LYP/6-311G++(d) level
[Pd(acac)(Ala)] |
[Pd(acac)(Val)] |
Bond lengths (Å) |
Pd–O6 |
2.038 |
Pd–O6 |
2.038 |
Pd–O5 |
2.030 |
Pd–O5 |
2.031 |
Pd–N17 |
2.072 |
Pd–N17 |
2.068 |
Pd–O19 |
2.025 |
Pd–O19 |
2.023 |
![[thin space (1/6-em)]](https://www.rsc.org/images/entities/char_2009.gif) |
Bond angles (°) |
O5–Pd–O6 |
92.631 |
O5–Pd–O6 |
92.555 |
O5–Pd–O19 |
92.605 |
O5–Pd–O19 |
93.108 |
O6–Pd–N17 |
93.355 |
O6–Pd–N17 |
93.621 |
O19–Pd–N17 |
81.409 |
O19–Pd–N17 |
80.713 |
Table 9 Experimental and theoretical 1H NMR chemical shifts of [Pd(acac)(Ala)]
Proton number or group |
H(4) |
H(27) |
Methyl group of acac protons |
Methyl group of alanine protons |
Experimental |
5.58 |
3.18 |
2.19 |
1.21 |
Theoretical |
4.80 |
3.15 |
1.63 |
0.86 |
Table 10 Experimental and theoretical 1H NMR chemical shifts of [Pd(acac)(Val)]
Proton number or group |
H(4) |
H(25) |
Methyl group of acac protons |
Methyl group of valine protons |
H(23) |
Experimental |
5.51 |
3.07 |
2.11 |
1.00 |
1.90 |
Theoretical |
4.80 |
2.75 |
1.63 |
0.69 |
1.42 |
The frontier molecular orbitals played an important role in the reactivity of the compounds and in many electric and optic properties. The most important orbitals in a molecule are highest occupied molecular orbital (HOMO) and lowest unoccupied molecular orbital (LUMO). The difference energy between HOMO and LUMO; the band gap; used as a measure of the excitability of the molecule. Therefore small energy gap means low excitation energies for many excited states and known as soft molecule. On the other hand, having a large energy gap means high excitation energies for many excited states and known as hard molecule.
The 3D plots of the HOMO and the LUMO of Pd(II) complexes are obtained to get more information about these molecules (see Fig. 16). It is shown that the HOMO and LUMO are localized on almost the acac ligand. Recently, the band gap has been used to prove the bioactivity from intra-molecular charge transfer (ICT) and describes the chemical reactivity, optical polarizability, kinetic stability, and chemical softness–hardness of a molecule.84–86 By using HOMO and LUMO energy values for a molecule, several global molecular descriptors, e.g., electron affinity, ionization potential, electronegativity and chemical softness–hardness can be calculated as follows (Table 11):
χ = (I + A)/2 η = (I − A)/2 S = 1/2η where I = −EHOMO and A = −ELUMO. |
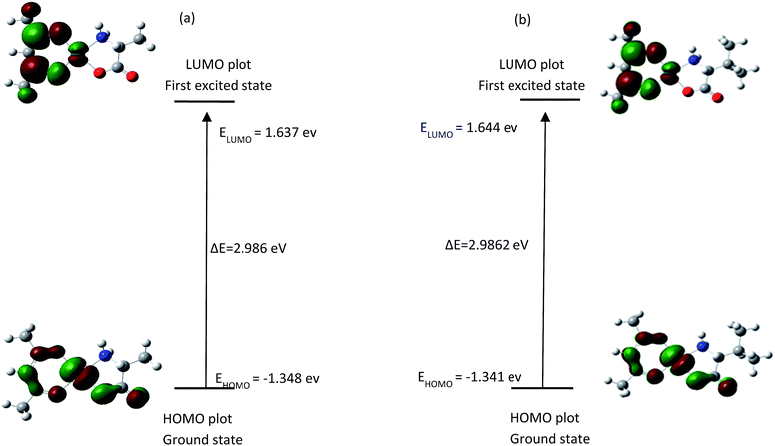 |
| Fig. 16 The frontier molecular orbitals of [Pd(acac)(Ala)] (a), and [Pd(acac)(Val)] (b). | |
Table 11 Electron affinity, ionization potential, electronegativity and chemical softness–hardness
|
[Pd(acac)(Ala)] |
[Pd(acac)(Val)] |
HOMO |
−1.348848 |
−1.341504 |
LUMO |
1.637168 |
1.644784 |
Ionization potential |
1.348848 |
1.341504 |
Electron affinity |
−1.637168 |
−1.644784 |
Electronegativity |
−0.14416 |
−0.15164 |
Hardness |
1.493008 |
1.493144 |
Softness |
0.746504 |
0.746572 |
ΔE |
2.986016 |
2.986288 |
Molecular docking analyses
Molecular docking is a well-established computational technique for prediction of the interactions between the molecules and macromolecules. Also, it can be applied in order to find out the binding character and optimized orientations of the molecule, which results in a new complex with overall minimum energy. The experimental results are complemented with the molecular modeling in which the Pd(II) complex is docked to DNA and BSA in order to characterize the preferred binding sites and modes.41
Besides, docking studies of Pd(II) complexes with DNA duplex of sequence d(CGCGAATTCGCG)2 has been investigated and the results are listed in Table 12 as binding free energies. As shown in Table 12, the binding free energy values are dominated by the vdW_hb_desolv energy are negative, suggesting that the binding events of the two complexes are spontaneous. As well as generally accepted, the lower relative binding free energy results in the more potent of binding affinity of compounds and DNA.87 Since the binding free energy value of [Pd(acac)(Val)] is lower than that of [Pd(acac)(Ala)], it demonstrates that, [Pd(acac)(Val)] displays a higher binding ability as compared to [Pd(acac)(Ala)]. The lowest energy conformations of the docked structures of the present complexes with DNA have been illustrated in Fig. 17. The docking models with DNA shows that, [Pd(acac)(Ala)] and [Pd(acac)(Val)] are located in the DNA major groove in the region of GC–CG and GC–AT residues respectively. These results are very close to DNA-binding affinity obtained from the above experimental observations.
Table 12 Docking results for Pd(II) complexes with DNA at 298 K (unit: kJ mol−1)
Complexes |
Estimated free energy of binding (ΔGbinding)a |
vdW + Hbond + desolv energy |
Electrostac energy |
Final total internal energy |
Torsional free energy |
Unbound system's energy |
ΔGbinding = ΔGvdW+hb+desolv + ΔGelec + ΔGtotal + ΔGtor − ΔGunb. |
[Pd(acac)(Ala)] |
−24.066 |
−14.322 |
−9.744 |
0 |
0 |
0 |
[Pd(acac)(Val)] |
−24.15 |
−18.354 |
−6.972 |
−0.252 |
+1.134 |
−0.252 |
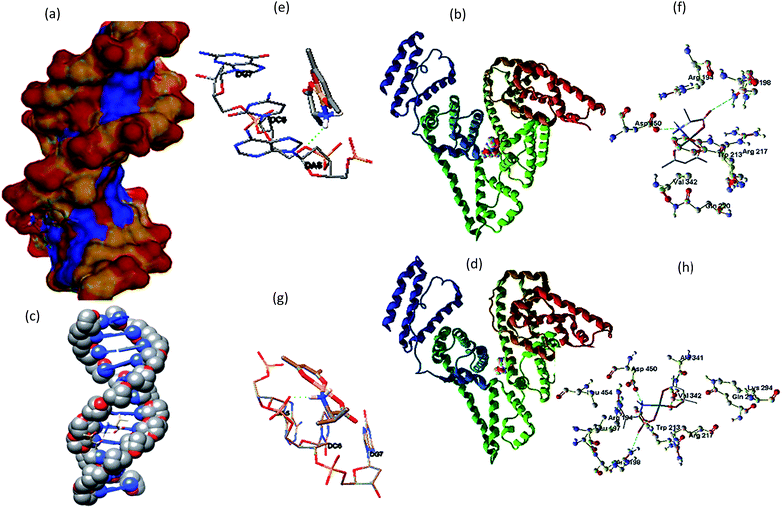 |
| Fig. 17 Molecular docked model of [Pd(acac)(Ala)] (a & b), and [Pd(acac)(Val)] (c & d), complexes with DNA and BSA. Also the interaction mode between [Pd(acac)(Ala)] (e & f), and [Pd(acac)(Val)] (g & h), complexes with DNA and BSA are shown. Dashed line displaying hydrogen bond interaction between them. | |
In order to understand the effect of a biologically active drug-molecule on function as a therapeutic agent, the understanding of its binding location in the model transport protein environment is very crucial.88
The globular protein BSA has made three homologous domains (I, II, III) which are divided into nine loops (L1–L9) by seventeen disulfide bonds so that each domain is formed of two sub-domains (A and B). The main regions of ligand binding sites in BSA are located in two hydrophobic pockets in sub-domains IIA and IIIA, namely site I and site II (Fig. 18).89 As depicted in Fig. 17, both Pd(II) complexes are situated in the subdomain IIA cavity which indicates that the complex formed through the binding of [Pd(acac)(Ala)] or [Pd(acac)(Val)] to sub-domain IIA which is more stable than sub-domain IIIA in terms of free energy. The amino acid residues involved in complexes–BSA interactions and binding free energy values for the complexes are summarized in Table 13. In this regard, Table 13 shows that, [Pd(acac)(Val)] binds to BSA stronger than [Pd(acac)(Ala)], which is in consistent with the experimental results. Since both complexes have represented the ability of strong binding to DNA and BSA, it is considered worthwhile to study the in vitro anticancer activity of the two complexes in details.
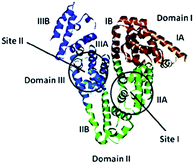 |
| Fig. 18 The structure of BSA. | |
Table 13 Docking results for Pd(II) complexes with BSA at 298 K
Complexes |
Binding energy (kJ mol−1) |
List of amino acid |
[Pd(acac)(Ala)] |
−25.242 |
Ala341, Arg194, Arg198, Arg217, Asp450, Gln220, Leu454, Lys221, Lys294, Trp213, Tyr340, Val342 |
[Pd(acac)(Val)] |
−28.182 |
Ala341, Arg194, Arg198, Arg217, Asp450, Gln220, Leu197, Leu454, Lys221, Lys294, Pro446, Trp213, Tyr340, Val342 |
Conclusion
Two new, water soluble and neutral acetylacetonatopalladium(II) complex of amino acids (alanine and valine) were designed and synthesized. Their structures were characterized on the basis of elemental analysis, conductivity measurement, FT-IR, UV-Vis, 1H-NMR and ESI-MS techniques. The results showed that both acetylacetone and amino acids coordinated as bidentate chelate to the Pd(II) center in a square planar array (Fig. 1). The in vitro cytotoxic activity studies by MTT assay against cancer model cell line K562, indicated that the complexes have good activity potential and possible future improvements. The interactions of these neutral complexes with CT-DNA and BSA were carried out by using absorption spectroscopy, fluorescence titration spectra, ethidium bromide titration and viscosity measurements studies. The results suggest that: (a) both complexes exothermically interact with CT-DNA and BSA at quite low concentrations, (b) interaction affinity of valine complex is more than that of alanine complex, (c) each of the Pd(II) complexes can be stored and moved by the BSA in the body fluid without damaging BSA molecules. This may avoid toxic side effects of complexes in the biological systems, (d) alanine and valine complexes interact with CT-DNA by groove binding and possibly get loaded in main binding site of BSA, (e) probable quenching mechanism of CT-DNA–EB and BSA solutions is a static quenching, (f) number of binding sites for the Pd(II) complexes on CT-DNA and BSA is approximately equal unity, (g) thermodynamic parameters indicated that binding force of both neutral Pd(II) complexes are spontaneous and the complexes were holded in DNA grooves and hydrophobic pocket of BSA via hydrogen and van der Waals binding, (h) the results of molecular docking calculations of [Pd(acac)(Ala)] and [Pd(acac)(Val)] complexes for CT-DNA and BSA are in good accordance with above experimental results. We conclude that these types of complexes may be helpful in the development of drug formulation.
Acknowledgements
Funding of our research from the university of Sistan and Baluchestan (USB) is gratefully acknowledged.
References
- B. Rosenberg, L. Van Camp and T. Krigas, Nature, 1965, 205, 698–699 CrossRef CAS PubMed.
- J. Zhang, L. Li, L. Wang, F. Zhang and X. Li, Eur. J. Med. Chem., 2010, 45, 5337–5344 CrossRef CAS PubMed.
- M. Eslami Moghadam, M. Saidifar, A. Divsalar, H. Mansouri-Torshizi, A. A. Saboury, H. Farhangian and M. Ghadamgahi, J. Biomol. Struct. Dyn., 2016, 34, 206–222 CAS.
- M.-C. Zhu, X.-T. Cui, F.-C. Zhao, X.-Y. Ma, Z.-B. Han and E.-J. Gao, RSC Adv., 2015, 5, 47798–47808 RSC.
- M. Frik, J. Jiménez, V. Vasilevski, M. Carreira, A. de Almeida, E. Gascón, F. Benoit, M. Sanaú, A. Casini and M. Contel, Inorg. Chem. Front., 2014, 1, 231–241 RSC.
- V. X. Jin and J. D. Ranford, Inorg. Chim. Acta, 2000, 304, 38–44 CrossRef CAS.
- D. Ajloo, M. E. Moghadam, K. Ghadimi, M. Ghadamgahi, A. A. Saboury, A. Divsalar, M. SheikhMohammadi and K. Yousefi, Inorg. Chim. Acta, 2015, 430, 144–160 CrossRef CAS.
- J. Zhang, L. Ma, H. Lu, Y. Wang, S. Li, S. Wang and G. Zhou, Eur. J. Med. Chem., 2012, 58, 281–286 CrossRef CAS PubMed.
- F. Rocha, C. Barra, A. V. d. G. Netto, A. E. Mauro, I. Carlos, R. C. G. Frem, S. Ananias, M. Quilles, A. Stevanato and M. Da Rocha, Eur. J. Med. Chem., 2010, 45, 1698–1702 CrossRef CAS PubMed.
- E.-J. Gao, M.-C. Zhu, Y. Huang, L. Liu, H.-Y. Liu, F.-C. Liu, S. Ma and C.-Y. Shi, Eur. J. Med. Chem., 2010, 45, 1034–1041 CrossRef CAS PubMed.
- J. Ruiz, J. Lorenzo, C. Vicente, G. López, M. Monge, F. X. Avilés, D. Bautista, V. Moreno and A. Laguna, Inorg. Chem., 2008, 47, 6990–7001 CrossRef CAS PubMed.
- S. Mylonas, A. Valavanidis, V. Voukouvalidis and M. Polyssiou, Chem. Informationsdienst, 1981, 12(33), 2199–2924 Search PubMed.
- A. I. Anzellotti, M. Sabat and N. Farrell, Inorg. Chem., 2006, 45, 1638–1645 CrossRef CAS PubMed.
- H. Mansoori-Torshizi, M. Islami-Moghaddam and A. A. Saboury, Acta Biochim. Biophys. Sin., 2003, 35, 886–890 CAS.
- M. Marques, ISRN Spectrosc., 2013, 2013, 287353 CrossRef.
- V. T. Yilmaz, C. Icsel, F. Suyunova, M. Aygun, N. Aztopal and E. Ulukaya, Dalton Trans., 2016, 45, 10466–10479 RSC.
- K. Karami, Z. Shirani-Sarmazeh, M. Hosseini-Kharat, J. Lipkowski and M. Saeidifar, J. Photochem. Photobiol., B, 2015, 144, 11–19 CrossRef CAS PubMed.
- P. Kalaivani, R. Prabhakaran, M. Kaveri, R. Huang, R. Staples and K. Natarajan, Inorg. Chim. Acta, 2013, 405, 415–426 CrossRef CAS.
- N. Zhou, Y.-Z. Liang and P. Wang, J. Photochem. Photobiol.,
A, 2007, 185, 271–276 CrossRef CAS.
- K. Karami, M. Hosseini-Kharat, H. Sadeghi-Aliabadi, J. Lipkowski and M. Mirian, Eur. J. Med. Chem., 2014, 73, 8–17 CrossRef CAS PubMed.
- L. Zhu, K. Zheng, Y.-T. Li, Z.-Y. Wu and C.-W. Yan, J. Photochem. Photobiol., B, 2016, 155, 86–97 CrossRef CAS PubMed.
- S. Jovanovic, K. Obrencevic, Z. D. Bugarcic, I. Popović and B. Petrovic, Dalton Trans., 2016, 45, 12444–12457 RSC.
- A. Divsalar, I. Zhila, A. A. Saboury, M. Nabiuni, M. Razmi and H. Mansuri-Torshizi, J. Iran. Chem. Soc., 2013, 10, 951–959 CrossRef CAS.
- A. Divsalar, A. Saboury, R. Yousefi, A. Moosavi-Movahedi and H. Mansoori-Torshizi, Int. J. Biol. Macromol., 2007, 40, 381–386 CrossRef CAS PubMed.
- K. Nakamoto, Infrared and Raman Spectra of Inorganic and Coordination Compounds, Wiley Online Library, 1986 Search PubMed.
- S. P. Khranenko, E. A. Bykova, S. A. Gromilov, M. R. Gallyamov, S. G. Kozlova, N. K. Moroz and S. V. Korenev, Polyhedron, 2012, 31, 272–277 CrossRef CAS.
- R. J. Angelici, Synthesis and technique in inorganic chemistry, 1969 Search PubMed.
- G.-J. Chen, X. Qiao, P.-Q. Qiao, G.-J. Xu, J.-Y. Xu, J.-L. Tian, W. Gu, X. Liu and S.-P. Yan, J. Inorg. Biochem., 2011, 105, 119–126 CrossRef CAS PubMed.
- Z. Fu, X. Chen and F. Jiao, Lat. Am. Appl. Res., 2012, 42, 211–216 Search PubMed.
- M. Chikira, J. Inorg. Biochem., 2008, 102, 1016–1024 CrossRef CAS PubMed.
- M. A. Teir, J. Ghithan, S. Darwish and M. M. Abu-Hadid, J. Appl. Biol. Sci., 2012, 6, 45–55 Search PubMed.
- S. Shahraki, H. Mansouri-Torshizi, A. Heydari, A. Ghahghaei, A. Divsalar, A. Saboury, H. Ghaemi, M. Doostkami and S. Zareian, Iran. J. Sci. Technol., 2015, 39, 187 Search PubMed.
- N. Shahabadi and M. Maghsudi, J. Mol. Struct., 2009, 929, 193–199 CrossRef CAS.
- S. Shahraki, H. Mansouri Torshizi, Z. Sori Nezami, A. Ghahghaei, F. Yaghoubi, A. Divsalar, A. A. Saboury and F. H. Shirazi, Iran. J. Pharm. Res., 2014, 13, 1279–1294 CAS.
- M. Islami-Moghaddam, H. Mansouri-Torshizi, A. Divsalar and A. Saboury, J. Iran. Chem. Soc., 2009, 6, 552–569 CrossRef CAS.
- M. Saeidifar, H. Mansouri-Torshizi, Y. Palizdar, M. Eslami-Moghaddam, A. Divsalar and A. A. Saboury, Acta Chim. Slov., 2014, 61, 126–136 CAS.
- R. Yousefi, R. Mohammadi, A. Taheri-Kafrani, M. Bagher Shahsavani, M. Dadkhah Aseman, S. Masoud Nabavizadeh, M. Rashidi, N. Poursasan and A.-A. Moosavi-Movahedi, J. Lumin., 2015, 159, 139–146 CrossRef CAS.
- E. M. Mrkalić, R. M. Jelić, O. R. Klisurić and Z. D. Matović, Dalton Trans., 2014, 43, 15126–15137 RSC.
- S. I. Pillai, K. Vijayaraghavan and S. Subramanian, Der Pharma Chemica, 2014, 6(1), 379–389 CAS.
- S. Shahraki, F. Shiri, H. Mansouri-Torshizi and J. Shahraki, J. Iran. Chem. Soc., 2015, 1–9 Search PubMed.
- H. Li, J. Chem., 2013, 2013, 494706 Search PubMed.
- M. Arivazhagan and S. Jeyavijayan, Spectrochim. Acta, Part A, 2011, 79, 376–383 CrossRef CAS PubMed.
- G. Petersson and M. A. Al-Laham, J. Chem. Phys., 1991, 94, 6081–6090 CrossRef CAS.
- U. Gowthaman, M. Jayakanthan and D. Sundar, BMC Bioinf., 2008, 9, 1 CrossRef PubMed.
- T. Khamkar, M. Abhyankar, G. Tendulkar, V. G. Khanna and K. Kannabiran, Adv. Chem. Eng. Sci., 2013, 2, 24–29 Search PubMed.
- Z. Asadi, M. Asadi and M. R. Shorkaei, J. Iran. Chem. Soc., 2016, 13, 429–442 CrossRef CAS.
- K. Misra, G. K. Ghosh, I. Mitra, S. Mukherjee, W. Linert, B. Misini, S. Mukhopadhyay and S. C. Moi, RSC Adv., 2015, 5, 12454–12462 RSC.
- Y. W. Yi and I. Bae, DNA Repair, 2011, 10, 1084 CAS.
- Q. Wang, W. Li, F. Gao, S. Li, J. Ni and Z. Zheng, Polyhedron, 2010, 29, 539–543 CrossRef CAS.
- K. Karami, Z. M. Lighvan, S. A. Barzani, A. Y. Faal, M. Poshteh-Shirani, T. Khayamian, V. Eigner and M. Dušek, New J. Chem., 2015, 39, 8708–8719 RSC.
- H.-y. Liu, Z.-h. Xu, X.-h. Liu, P.-x. Xi and Z.-z. Zeng, Chem. Pharm. Bull., 2009, 57, 1237–1242 CrossRef CAS PubMed.
- J. E. Huheey, E. A. Keiter and R. L. Keiter, Inorganic chemistry: principles of structure and reactivity, Harper and Row, 1983, pp. 160–162 Search PubMed.
- H. Mansuri-Torshizi, T. Srivastava, H. Parekh and M. Chitnis, J. Inorg. Biochem., 1992, 45, 135–148 CrossRef CAS PubMed.
- U. Chaveerach, A. Meenongwa, Y. Trongpanich, C. Soikum and P. Chaveerach, Polyhedron, 2010, 29, 731–738 CrossRef CAS.
- I. R. Edwards and J. K. Aronson, Lancet, 2000, 356, 1255–1259 CrossRef CAS.
- Q.-L. Zhang, J.-G. Liu, H. Chao, G.-Q. Xue and L.-N. Ji, J. Inorg. Biochem., 2001, 83, 49–55 CrossRef CAS PubMed.
- A. Shanmugapriya, G. Kalaiarasi, P. Kalaivani, F. Dallemer and R. Prabhakaran, Inorg. Chim. Acta, 2016, 449, 107–118 CrossRef CAS.
- L. Tabrizi, H. Chiniforoshan and H. Tavakol, Spectrochim. Acta, Part A, 2015, 141, 16–26 CrossRef CAS PubMed.
- S. Jahani, M. Khorasani-Motlagh and M. Noroozifar, J. Biomol. Struct. Dyn., 2016, 34, 612–624 CAS.
- Z. Aramesh-Boroujeni, M. Khorasani-Motlagh and M. Noroozifar, J. Biomol. Struct. Dyn., 2016, 34, 414–426 CAS.
- L. K. Fraiji, D. M. Hayes and T. Werner, J. Chem. Educ., 1992, 69, 424 CrossRef CAS.
- M. Hof, R. Hutterer, V. Fidler and O. Wolfbeis, Proteins, DNA, and Cells, 2005 Search PubMed.
- H. Derakhshankhah, A. A. Saboury, R. Bazl, H. A. Tajmir-Riahi, M. Falahati, D. Ajloo, H. Mansoori-Torshizi, A. Divsalar, A. Hekmat and A. A. Moosavi-Movahedi, J. Iran. Chem. Soc., 2012, 9, 737–746 CrossRef CAS.
- S. Shaikh, J. Seetharamappa, P. Kandagal and D. Manjunatha, Int. J. Biol. Macromol., 2007, 41, 81–86 CrossRef CAS PubMed.
- B. H. Hussein, J. Lumin., 2011, 131, 900–908 CrossRef CAS.
- A. Belatik, S. Hotchandani, J. Bariyanga and H. Tajmir-Riahi, Eur. J. Med. Chem., 2012, 48, 114–123 CrossRef CAS PubMed.
- T. Mukherjee, B. Sen, E. Zangrando, G. Hundal, B. Chattopadhyay and P. Chattopadhyay, Inorg. Chim. Acta, 2013, 406, 176–183 CrossRef CAS.
- A. Divsalar, A. A. Saboury, L. Ahadi, E. Zemanatiyar and H. Mansouri-Torshizi, BMB Rep., 2010, 43, 766–771 CrossRef CAS PubMed.
- R. C. Gupta and G. Spencer-Beach, Regul. Toxicol. Pharmacol., 1996, 23, 14–21 CrossRef CAS PubMed.
- S.-S. Wu, W.-B. Yuan, H.-Y. Wang, Q. Zhang, M. Liu and K.-B. Yu, J. Inorg. Biochem., 2008, 102, 2026–2034 CrossRef CAS PubMed.
- L. Yang, D. Huo, C. Hou, M. Yang, H. Fa and X. Luo, Spectrochim. Acta, Part A, 2011, 78, 1349–1355 CrossRef PubMed.
- J. M. Kelly, A. B. Tossi, D. J. McConnell and C. OhUigin, Nucleic Acids Res., 1985, 13, 6017–6034 CrossRef CAS PubMed.
- D. Suh and J. B. Chaires, Bioorg. Med. Chem., 1995, 3, 723–728 CrossRef CAS PubMed.
- Z.-H. Xu, F.-J. Chen, P.-X. Xi, X.-H. Liu and Z.-Z. Zeng, J. Photochem. Photobiol., A, 2008, 196, 77–83 CrossRef CAS.
- A. Sułkowska, J. Mol. Struct., 2002, 614, 227–232 CrossRef.
- G. Kaur, S. Kumar, N. Dilbaghi, B. Kaur, R. Kant, S. K. Guru, S. Bhushan and S. Jaglan, Dalton Trans., 2016, 45, 6582–6591 RSC.
- Y.-Q. Wang, H.-M. Zhang, G.-C. Zhang, W.-H. Tao and S.-H. Tang, J. Lumin., 2007, 126, 211–218 CrossRef CAS.
- J. R. Lakowicz and G. Weber, Biochemistry, 1973, 12, 4161–4170 CrossRef CAS PubMed.
- V. Anbazhagan and R. Renganathan, J. Lumin., 2008, 128, 1454–1458 CrossRef CAS.
- P. Banerjee, S. Ghosh, A. Sarkar and S. C. Bhattacharya, J. Lumin., 2011, 131, 316–321 CrossRef CAS.
- X.-D. Jin, Y. Yang, Y. Jiang, X. Han, X. Wan, D. Liu, X.-M. Song and X. Chang, J. Organomet. Chem., 2015, 791, 24–33 CrossRef CAS.
- Y. Yang, Q. Hu, Y. Fan and H. Shen, Spectrochim. Acta, Part A, 2008, 69, 432–436 CrossRef PubMed.
- S. Bi, D. Song, Y. Tian, X. Zhou, Z. Liu and H. Zhang, Spectrochim. Acta, Part A, 2005, 61, 629–636 CrossRef PubMed.
- H. Moghanian, A. Mobinikhaledi and R. Monjezi, J. Mol. Struct., 2013, 1052, 135–145 CrossRef CAS.
- S. Mukherjee, I. Mitra, R. Saha, S. R. Dodda, W. Linert and S. C. Moi, RSC Adv., 2015, 5, 76987–76999 RSC.
- D. Farmanzadeh and S. Ghazanfary, Struct. Chem., 2014, 25, 293–300 CrossRef CAS.
- K. Zheng, L. Jiang, Y.-T. Li, Z.-Y. Wu and C.-W. Yan, RSC Adv., 2015, 5, 51730–51744 RSC.
- R. Mi, Y.-J. Hu, X.-Y. Fan, Y. Ouyang and A.-M. Bai, Spectrochim. Acta, Part A, 2014, 117, 163–169 CrossRef CAS PubMed.
- J. Ao, L. Gao, T. Yuan and G. Jiang, Chemosphere, 2015, 119, 590–600 CrossRef CAS PubMed.
Footnote |
† Electronic supplementary information (ESI) available. See DOI: 10.1039/c6ra18803f |
|
This journal is © The Royal Society of Chemistry 2016 |
Click here to see how this site uses Cookies. View our privacy policy here.