DOI:
10.1039/C6RA18636J
(Paper)
RSC Adv., 2016,
6, 84505-84511
New post-processing method of preparing nanofibrous SERS substrates with a high density of silver nanoparticles
Received
22nd July 2016
, Accepted 29th August 2016
First published on 30th August 2016
Abstract
SERS enabling substrates with increased sensitivity, their manufacturing reproducibility and enhanced structural stability are highly demanded in SERS applications. Therefore our main aim was to elaborate a simple and inexpensive method of functionalization of electrospun chitosan nanofibers by using silver nanoparticles (AgNP). For that purpose we established a protocol where we were able to control the density of AgNP on the surfaces of nanofibers, and thus electromagnetic hotspots, simply by variation of Tollens' reagent. By webbing of such fibers we were able to prepare films that were enabling SERS either of solutes or macromolecular structures such as bacterial cells. Especially, to detect bacterial cells we established two approaches of immobilisation of cells within the films, which enabled their detection with enhancement of sensitivity by a factor of 105 and an average of 25% spot-to-spot variation.
1 Introduction
Surface-enhanced Raman spectroscopy (SERS) is a powerful analytical method, since it enables several orders of magnitude enhancement of Raman signal. Enhancement of the signal is generated by plasmonic nanoparticles that are in most cases made up of gold or silver. On the surface of such metal moieties within plasmonic nanoparticles the localized surface plasmon resonance of electrons is occurring upon light illumination.1 Theoretically, since the enhancement factor can be as large as 1012, it gives possibilities to detect single molecules.2 Because of these features SERS can be a very appropriate analytical method for use in biomedical applications,3 such as measuring pH either in living cells or in surrounding milieu,4 cancer targeting,5 glucose,5 protein6 as well as nucleic acids.7 In such applications robust and uniform SERS substrates are more convenient than aggregated nanoparticles although currently the latter are more frequently used.8,9 So far, most studies have been focused on the creation of 1D and 2D SERS substrates.10–12 For example, single-walled carbon nanotubes decorated either with silver or gold nanoparticles are an interesting 1D SERS multifunctional nanoprobe for detection and imaging of biological samples.13,14 Single polyaniline@Ag nanofibers, silver nanowires decorated with gold nanoparticles and multilayered rod-like nanocapsules oriented by electric tweezers have been designed for sensitive analyte detection.15–17 Although the efficacy of 1D SERS nanoprobes their practical use is restricted due to limited concentration of hot spots in the irradiated area. In this regard, much attention has been paid to three-dimensional (3D) organization of SERS substrate.18 The large surface area together with porous structure of gold nanosponges, aluminum membrane, nano-dendritic crystal film, paper and cellulose filter, vertically aligned nanotubes have been suggested to concentrate the number of hot spots and sites for adsorption of analyte molecules and therefore improve the sensitivity of SERS substrate.19–22 In this respect, nanofibrous-based SERS substrates obtained by electrospinning method can be easily impregnated with plasmonic nanoparticles providing SERS activity, reveal excellent mechanical strength and flexibility, cost-effective, and can be integrated within microfluidic based systems and ultra-thin layer chromatography to automatize measurements.23–26 These soft films can be also suitable for stand-off measurements, by means to provide hands off SERS based diagnostics.27 Additionally, intrinsic porosity of such material made out of nanofiber mesh can be effectively used for capturing and concentrating of analytes from fluids such as small molecules28 and whole microbial cells.29
Until now, it has been shown that functionalization of electrospun polymeric nanofiber films with plasmonic nanoparticles can be achieved either by impregnation of nanoparticles to as-prepared substrates30–33 or by mixing with a polymer solution before electrospinning.34 However, the simple and fast modification of electrospun nanofibers with plasmonic nanoparticles remains a significant challenge. Therefore, it is essential to establish a new method for the fabrication of SERS based substrates made of electrospun films. In that sense it can be applied an approach that silver nanoparticles (AgNPs), which are good Raman enhancers, can be produced by very simple reduction of Ag ions at room temperature by reactants such as glucose. This approach can be used in situ, directly on already formed substrates, to produce nanoparticles and functionalize nanofibers at the same time. By development of such method, the production of SERS substrates can widen possibilities of their use and can speed-up production process. This can be achieved by the separation of electrospinning process from the process of formation of nanoparticles, and increasing density of nanoparticles within the mesh above the current limitation. The separation of two processes, widen the use of different formulations of solutions of electrospinning polymers, since nanoparticles do not need to be added to the polymer solutions in prior of electrospinning. Moreover, the high density of nanoparticles after electrospinning, needed for SERS, cannot be achieved by conventional methods, since the incorporation of formed nanoparticles within the mesh does not allow increasing of amount of particles above the mesh holding capacity specific for the formed particles. Accordingly, aims of our work were: (i) to develop a method for functionalization of electrospun polymer nanofibers by in situ synthesis of AgNPs and (ii) to characterize dynamic range of use of this substrate by detection either of single type molecules (e.g. Rhodamine B) or large bulk analytes composed of many different types of molecules (e.g. bacterial cells) by using SERS.
2 Experimental
2.1 Materials
Silver nitrate (AgNO3), D-(+)-glucose (C6H12O6, 180.16 Da, ≥99.5%), NH4OH, poly(ethylene oxide) (PEO, 1000 kDa), Rhodamine B (dye content ∼95%, 479.01 Da) were purchased from Sigma-Aldrich. Chitosan (200 kDa, 80–85% degree of deacetylation) purchased from Joint-Stock Company “Bioprogress” (Russia). In all experiments, Mili Q water with resistivity higher than 18.2 MΩ cm was used.
2.2 Synthesis of electrospun nanofiber films
Chitosan (6% w/v) and PEO (1% w/v) solutions in 70% acetic acid were mixed together under vigorous stirring in a 93
:
3 ratio, respectively. The mixture was further stirred up to 2 h. Nonwoven chitosan nanofiber films were prepared by NanoSpider NS 200 (Elmarco, Czech Republic), a needle-free laboratory scale electrospinning device. Four-wires rotating spinning electrode and the collecting electrode were separated for 150 mm and voltage of 80 kV was applied between spinning and collecting electrodes.
2.3 Preparation of silver chitosan nanofiber films
Prepared nanofibers films (2 cm × 1 cm) by above described electrospinning process were immersed in a mixture of ethanol and Tollens' reagent with ratio 1
:
1 in Petri dish (D × H: 35 mm × 10 mm, volume: 2.4 mL). To prepare Tollens' complex equal volume of two solutions with the same, but variable concentrations (0.1 M, 0.5 M, 1.0 M) in different experiments, of AgNO3 and ammonium hydroxide were mixed together. Then 1 mL D-glucose solution (40% w/v) was added to the reaction volume and immediately the colour of nanofiber films became red stained. After 1 min, films were removed from the reaction volume and were twice washed with ethanol and then with water. At this stage, prepared samples were in a “first reduction” step and the samples hereafter referred as SCNFs-1. The whole protocol was repeated in a “second reduction” step (referred as SCNFs-2).
2.4 Characterization
Scanning electron microscopy (SEM) images were obtained by Philips XL30 electron microscope at an accelerating voltage of 3 kV. To perform Raman measurements we used a confocal Raman microscope (Renishaw inVia, UK) equipped with a diode-pumped 785 nm near IR laser excitation (60 mW) controlled by a neutral optical density filter. The laser beam was focused through a 50× (Leica N PLAN, NA = 0.5) microscope objective. The spectra were acquired with a thermoelectrically cooled CCD detector optimized for near IR (spectral resolution of 1 cm−1). All spectra were collected by using WiRE software V4.1 (Renishaw, UK) and SynchroScan was applied for processing of spectra. The baseline correction tool in the WiRE software V4.1 (Renishaw, U.K.) was used.
Optical properties of SCNFs were measured by using UV-vis spectrometer Synergy H1 Multi-Mode Reader (BioTek Instruments, Inc., USA). We determined material light absorbance properties via spectral scanning in steps of 20 nm from 300 nm up to 800 nm light wavelengths. For each sample, 9 points on the sample were measured. All data were normalized taking minimum value of 0 and divided by the sum.
To perform SERS measurements SCNFs-1 and SCNFs-2 samples were incubated in the water solution of 10−5 M Rhodamine B for 1 hour and then washed in water. All SERS spectra of Rhodamine B were acquired from five randomly selected points on samples at a laser power of 0.06 mW and an integration time of 10 s. The reference spectrum of Raman signal of blank samples, the non-modified chitosan nanofiber films, were obtained after 1 h of their incubation in 10−3 M water solution of Rhodamine B.
To measure SERS signals from immobilized bacterial cells in SCNFs-1 two approaches were used. In the first approach 10 μL of suspension of Escherichia coli bacterial cells in the sterile 0.9% NaCl solution were pipetted on SCNFs-1 films. The SERS spectra were collected from the drop area before the region was dried. In the second approach, SCNFs-1 were immersed in a 1 mL 100 times diluted bacterial suspension and incubated for 20 min. The samples were then twice washed with sterile 0.9% NaCl solution and dried at room temperature in prior of SERS measurements. SERS spectra were acquired from 10 randomly selected points on the sample area at 0.06 mW of laser power and with an integration time of 10 s.
To calculate enhancement factor (EF) for Rhodamine B the following equation was used:
|
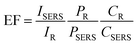 | (1) |
where
I,
P, and
C are intensity, laser power and concentration of an analyte respectively. The indexes correspond to normal Raman and SERS intensities.
Relative standard deviation (RSD) was calculated by using following equation:
|
 | (2) |
where SD corresponds to the standard deviation of the SERS intensity and
Im is the mean SERS intensity of a specific peak.
2.5 Image analysis
Analysis of the SEM images was done by using ImageJ software. Selected areas of silver chitosan nanofiber films on SEM images were chosen to count AgNPs. To calculate parameters of AgNPs distribution we were observing areas that are in one plane to ensure that AgNPs are distributed uniformly. The size of the area cross section of films is considered equal to a laser spot size (1.8 μm). For each sample, minimum five images were counted. The significant differences were defined at p < 0.05. To calculate a number of AgNPs a surface filling factor was calculated, which we define as nanoparticle cross section divided by the cross section of nanofiber in the film: |
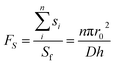 | (3) |
where si is cross section of nanoparticles, Sf corresponds to surface of nanofiber, r0 – is nanoparticle radius, D is diameter of nanofiber and h corresponds to height of nanofiber. The statistics of the surface filling factor was done by one-way ANOVA test. The surface filling factors for all samples were expressed in percentages.
2.6 Bacteria preparation and growth
Bacteria, Escherichia coli strain K-12, were cultivated overnight in liquid GRM medium (15 g and 10 g of pancreatic hydrolysate of fish flour and casein, respectively, 2 g of yeast extract, 3.5 g of NaCl and 1 g glucose in distilled water and adjusted to the final volume of 1 L). The 4 mL of overnight bacterial culture of optical density of about 1.2 measured at 600 nm wavelength (OD600) was centrifuged for 5 min at 5000 g at room temperature. Obtained pellet was three times washed with sterile 0.9% NaCl solution by suspension of pellet and centrifugation at 5000 g for 5 min. The suspension prepared in such a way contained 1.5 × 109 bacterial cells per mL.
Bacterial cells, approximately 108 cells, were stained with Syto14 (5 μM of final concentration in sterile 0.9% NaCl) for 30 min and protected from light. To remove dye after staining, cells were washed three times by centrifugation (see above) and then suspended in 100 μL sterile 0.9% NaCl.
3 Results and discussion
3.1 Preparation of silver chitosan nanofiber films
To cover chitosan nanofiber films with AgNPs we used an approach where these particles grew immediately after addition of both, the Tollens' reagent and a reducing agent, so called the silver mirror reaction (Fig. 1(a)). In our approach, the Ag ions in the Tollens' reagent are electrostatically attracted to hydroxylic and carboxylic groups present on the surface of chitosan fibres. The effective immobilization of negatively charged CTAB gold nanorods (GNRs) by varying CTAB concentration onto hydrophobic poly(L-lactic acid) (PLLA) nanofibrous paper has been shown recently.28 Then hydrophilic SERS active area have been used to confine analyte molecules within it and prevent the random spreading of rhodamine molecules. In our case, chitosan nanofiber films become saturated with Ag ions, which then act as precipitation nuclei, where in place the AgNPs get formed after the addition of glucose, a mild reducing agent. The Ag ions get reduced to the metalic silver due to presence of aldehyde group in glucose and the reduction can be monitored just by a visual inspection since it is formed “silver mirror”, immediately after mixing together the reagents.
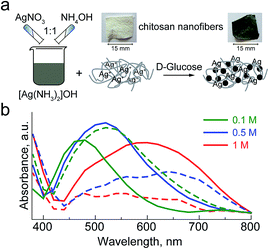 |
| Fig. 1 (a) Fabrication of silver chitosan nanofiber films (SCNFs) by using silver mirror reaction. Optical images correspond to blank chitosan nanofiber films and silver chitosan nanofiber films, respectively. (b) UV-vis adsorption spectra of SCNFs obtained at 0.1 M (green line), 0.5 M (blue line) and 1 M (red line) of Tollens' reagent. The solid and dashed lines correspond to the first and the second reduction reaction, respectively. | |
The SCNFs changed colour from milk white to dark grey after this reduction step (Fig. 1). In our experiments we observed that optical properties of SCNFs are dependent on the concentration of Tollens' reagent as well as on the numbers of applied reduction reactions (Fig. 1(b)). The excitation spectra of SCNFs exhibit clear difference in the plasmonic peak position. Peak shifts and becomes broader when the concentration of Tollens' reagent is increased. Therefore, the plasmon peak occurred at 470 nm when it was used 0.1 M of Tollens' complex and it was shifted to the 520 nm and 600 nm for samples prepared at 0.5 M and 1.0 M of Tollens' reagent, respectively. However, the position of the plasmonic peak and curve shape of SCNFs prepared at lower concentration of Tollens' reagent, either 0.1 M or 0.5 M, after the second reduction appear similar to SCNFs prepared at higher concentrations, 0.5 M or 1.0 M, respectively, after the first reduction. This phenomenon can be explained by the Mie model, since the size of nanoparticles increases and the interparticles distances decrease, either by higher concentration of the reagent or by additional reducing step at lower concentrations of reagents, resulting in the shift of the plasmon peak.35
Concentration of Tollens' reagent also affected the morphology of films (Fig. 2), especially the amount and distribution of AgNPs. We observed high quantity and uniform distribution of AgNPs as well as irregular growth of AgNP in SCNFs when using 0.5 M or 1.0 M versus 0.1 M concentration of Tollens' reagent, respectively. At the lowest concentration of Tollens' reagent (0.1 M) we also observed vast amount of aggregates of AgNPs that appeared mainly within the irregularities of fibre mesh and on the fibre junctions. The high quantity and uniform distribution of AgNP is a very important property of the material since this results in strong plasmon coupling, which exert in high efficiency of production of SERS signals.36
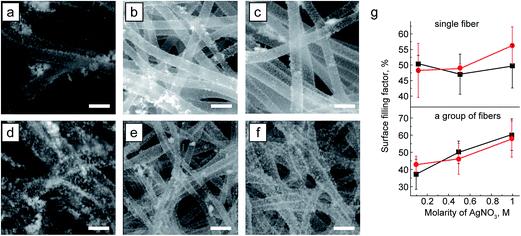 |
| Fig. 2 SEM images of SCNFs-1 and SCNFs-2 prepared at 0.1 M (a, d), 0.5 M (b, e) and 1.0 M (c, f) Tollens' reagent. Figures (a–c) and (d–f) show results of the first and the second reduction process, respectively. SEM images were taken at the back scattered electron mode (BSEM), the scale bar in all images corresponds to 500 nm. (g) Surface filling factor of AgNPs versus molar concentration of Tollens' reagent, estimated for a single nanofiber and a group of nanofibers from SEM images. Red and black curves correspond to the first and to the second reduction. | |
In order to study impact a number of AgNPs on the enhancement factor of SCNFs, all prepared samples were exposed to the second reduction at conditions similar to those applied in the first one. We found that after second reduction the concentration of AgNPs increased in all samples (Fig. 2). This can be attributed to the appearance of AgNPs at the non-occupied hydroxylic and carboxylic groups on the surface of chitosan fibers, which remained non-occupied with AgNP after the first reduction. At the end this resulted in higher densities of AgNPs (see Fig. 2). However, the increase of concentration of the Tollens' did not change dramatically of the surface density of AgNPs on SCNFs-2 (Fig. 2(g)). For example, the surface filling factor was changed just for group of nanofibers and from 40% to 60%, respectively, by the increase of concentration of Tollens' reagent. The low yield of surface density of AgNPs can be attributed to the growth of AgNPs in the solution and not on the surface of fibres either in the first or in the second Ag reduction process. On the other hand, the “vertical” growth of AgNPs can be responsible for the weak increase of the surface density of AgNPs in SCNFs.37 The average size of silver nanoparticles after first reduction was found to be 20 ± 3 nm and which independent on the concentration of Tollens' reagent. At the same time, the average size is increased to 45 ± 10 nm after second reduction and maximum size is about 60 ± 10 nm for 1 M Tollens' reagent.
3.2 SERS capability of SCNFs
Although all SCNFs are showing SERS signals, the SCNF prepared with the 1.0 M Tollens' reagent showed the strongest SERS intensities of the major Rhodamine signatures (Fig. 3(a and b)). The peaks at 737 cm−1 (aromatic ring in-plane bending), 1203 cm−1 (C–C stretching), 1281 cm−1 (C–O–C stretching) and 1507 cm−1 (aromatic stretching of the dye) were clearly observed in SERS spectra. There are peaks between 800 cm−1 and 1150 cm−1 that can be obtained from the chitosan, and not from the Rhodamine, since they appear only in both the unmodified films as well as in SCNFs. These rhodamine signal peaks were not overlapping with those from the bare chitosan nanofiber films, those films without added rhodamine.
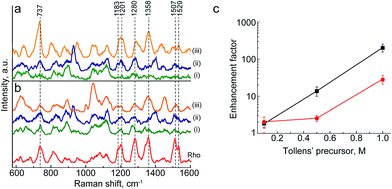 |
| Fig. 3 SERS spectra of Rho B acquired from silver functionalized chitosan substrates prepared after first (a) and second (b) reductions, respectively. Numbers in each curve correspond to different concentration of Tollens' reagent (i) −0.1 M; (ii) −0.5 M, (iii) −1 M. Raman spectrum of Rho B with concentration 10–3 M acquired from the unmodified chitosan substrate is shown in (b). All spectra were acquired through a 50× microscope objective using 785 nm wavelength. (c) An enhancement factor estimated by eqn (1) for three concentrations of Tollens' complex used for films functionalization. Here red and black curves correspond to the first and the second reduction process, respectively. The error bars show standard deviation. | |
To determine differences in SERS properties of SCNFs-1 and SCNFs-2, the analytical enhancement factor (AEF) was calculated according to eqn (1). The enhancement factor of SCNFs-1 and SCNFs-2 is increased as the concentration of Tollens' complex rises (Fig. 3(c)) with the maximum enhancement at about 174 ± 40 for SCNFs-1 obtained at the 1.0 M concentration of the Tollens' reagent. The enhancement factor did not change both for SCNFs-1 and SCNFs-2 fabricated at 0.1 M of Tollens' reagent. Although, the surface density of AgNPs was increased in SCNFs-2, the SERS performance of SCNFs-2 was lower than the SCNFs-1 (Fig. 3(c)). The optimal surface density distribution of AgNPs and thus electromagnetic hotspots in SCNFs-1 can be responsible for its better SERS performance.38 The additional reason for larger SERS efficiency of SCNFs-1 can be attributed as well as to the position of the plasmonic peak that was closer to the excitation wavelength at the 785 nm in the Raman measurements.
3.3 SERS detection of bacteria using SCNFs
We used model organism the E. coli K-12 strain to determine the potential of SCNFs to detect microorganisms by SERS. To immobilize bacterial cells in SCNFs-1 two approaches were used. In the first approach, the suspension of bacterial cells were pipetted on SCNFs-1 and then immediately the SERS spectra were measured. In the second approach, the SCNFs-1 were incubated in the suspension of bacteria for 20 min and then SERS measurements were made on dried samples. Since generally we did not apply techniques of bacterial cell lysis the Raman bands in obtained spectra can be assigned to biomolecules located in the bacterial cell wall (Table 1). The peak at 517 cm−1 can be attributed to the stretching of S–S bond.39,40 Two peaks at 573 and 578 cm−1 are usually characteristic of tryptophan and phosphatidylinositol,40 while 655 and 659 cm−1 bands assigned to (COO−) deformation.41,42 The strong peak at 738 cm−1 originated from adenine in the lipid part, while bands at 755 and 818 cm−1 are due to symmetric breathing of tryptophan and C–C stretching in spectrum of bacteria, which were pipetted on SCNFs-1. While bands at 517, 573, 655 and 738 cm−1 are measured only in SCNFs-1 incubated in bacteria suspension. These variations in bands position can be related to different interaction between biomolecules in bacteria wall and AgNPs in SCNFs-1. For example, pipetted bacteria bound poorly with SCNFs-1 and thus cell wall lipids are not in normal physiological conformation, which then appear in SERS spectrum as a band at 1463 cm−1 position.45 Additionally, the motion of bacterial cells during Raman measurements can reduce interaction between biomolecules in cell wall and AgNPs in SCNFs-1, which then change SERS signatures in the spectrum. In addition, the shift of Raman bands can be assigned to release of some of internal components out from bacterial cells due to the drying of cells and consequently the disintegration of the cell wall and membrane.
Table 1 Assignments of SERS signatures of Escherichia coli acquired from SCNFs and Raman bands of Escherichia coli taken from bacteria suspension in 0.9% NaCl
Raman peak position (cm−1) |
SERS peak position (cm−1) |
Assignments |
Pipetting |
Incubation |
517 |
|
517 |
(S–S) stretching |
573 |
578 |
573 |
Tryptophan, phosphatidylinositol |
655 |
659 |
655 |
(COO–) deformation |
725 |
755 |
738 |
Flavin, adenine, tryptophan |
1102 |
818 |
|
(C–C) stretching in protein |
1107 |
1102 |
1107 |
Phenylalanine |
1130 |
1130 |
|
CH2 deformations |
1204 |
1204 |
|
CH2 deformations |
|
1239 |
1271 |
Amide III |
1331 |
1323 |
1331 |
Adenine |
1445 |
1362 |
|
CH2 deformations |
1463 |
1463 |
1445 |
CH2 deformations |
On the other hand, incubation leaded to strong interaction of membrane biomolecules with SCNFs-1 and ultimately with AgNPs. The strong coupling may change structural properties of biomolecules inside or on the cell wall, which can be different from those prepared by pipetting bacteria on SCNFs-1.46 Strong coupling of bacteria with SCNFS-1, as observed proteins.43 Two peaks centred at 1102 and 1107 cm−1 are normally the contribution of phenylalanine amino acid within proteins.41 The bands at 1239 and 1271 cm−1 can be originated from amide III,41,42,44 while at 1323 and 1331 cm−1 can be assigned to adenine containing molecules.40 Contributions from the changes in lipids structure (CH2 deformations) appear at 1130, 1204, 1362 1450 and 1463 cm−1.40,41,44
The vibrational bands observed in SERS spectra of E. coli were different, which depended on the preparation of samples, either by incubation or by pipetting of bacterial cells. The presence of bands mainly differ at 500–850 cm−1 and 1000–1500 cm−1 regions of the SERS spectra. For example peaks at 578, 659, 677, 755, 818 cm−1 appears only in the SERS with confocal microscope (Fig. 4(c)), can resulted in the high SERS signals. Soaking bacteria into the intrinsically porous porosity chitosan nanofiber mesh resulted in very efficient binding within the matrix.29 After the immobilisation bacteria became restricted to move and this increased signal to noise ratio during the process of obtaining the SERS spectra. It can be also expected that the cell wall composition of E. coli cells between incubated or directly measured can be also responsible for the changes in the position of vibrational bands in the spectrum.
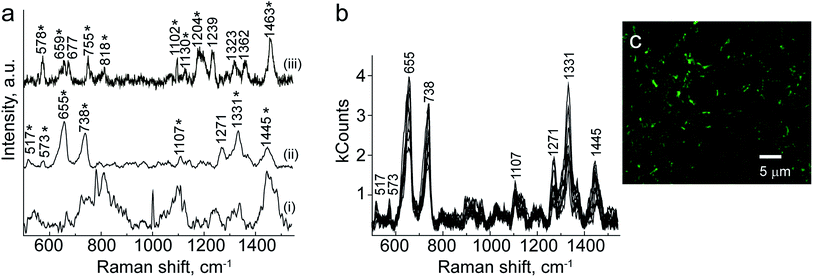 |
| Fig. 4 (a) SERS spectra of E. coli strain K-12 obtained from SCNFs-1 prepared at 0.1 M Tollens' reagent. (i) Normal Raman spectrum of E. coli; (ii) and (iii) are SERS spectra of E. coli immobilized on SCNFs-1 by incubation in microorganisms suspension and pipetting on SCNFs-1, respectively. (b) The SERS spectra of bacterial cells incubated with SCNFs-1, which acquired from 10 randomly selected points on a sample. The SERS signatures close to E. coli bands in normal Raman spectrum are marked with asterisks. (c) Fluorescent confocal image of dye stained bacterial cells adsorbed on SCNFs. | |
To use such platform to detect bacterial cells it is essential to estimate the enhancement factor for SERS signals. In this case we used most intensive SERS peak present at 1445 cm−1. Assuming that the average concentration of bacteria for Raman and SERS was around 1.5 × 109, the enhancement factor of E. coli can be 1.7 × 105. However, when taken into account a real number of bacterial cells within the laser spot, it can be calculated even larger enhancement. According to the size of the laser spot, which is about 2 μm for objective with 50× (NA = 0.5) magnification, and size of E. coli cells (0.4–0.8 × 1–3 μm), the maximum numbers of cells within the laser spot can be between 2 to 5. Based on this prediction the enhancement factor of E. coli in SERS measurements can be as much as 1010.
The reproducibility of the signal is a very important property of the SERS substrates when using in applications. To verify the spot-to-spot variation in the SERS spectra Raman measurements were collected from various areas in samples, after these samples were incubated with bacteria (Fig. 4(b)). To calculate relative standard deviation (RSD) the absolute intensity of the peak height in the position at 738 cm−1 was used. The RSD of 25% of this peak intensity collected from 10 points on SCNFs was determined. The obtained RSD is larger than that which has been achieved for detection of bacteria with convective assembly method,43 but it can be comparable with RSD shown for electrospun nanofibers modified with gold layers, reported just recently.29
4 Conclusions
We introduced method of fabrication SERS substrates by applying silver mirror reaction on electrospun chitosan nanofibers. This method allowed to simplify fabrication of electrospun nanofibers used as a SERS substrates. Based on one-way ANOVA test, we found that concentration of Tollens' reagent mainly influences on number of AgNPs in a group of nanofibers rather than in a single nanofiber. The enhancement factor of Rhodamine B is dependent on density of AgNPs and it was found to be uniform by measuring in several randomly selected positions on the SERS substrates. The immobilization of bacteria on SERS substrates is achieved by both pipetting bacteria suspension on nanofiber films and incubating SERS substrates in the suspension of the bacterial cells. Although two approaches allowed us enhancing bacteria signatures, incubation promote more effective enhancement reaching up to 105 and at the same time with very low spot-to-spot variations in SERS spectra. We envisioned that introduced methods would be feasible for the rapid diagnostics and discrimination of pathogens particularly bacterial cells, which remains one of the most challenged task in health care, clinical environments, food and water supplies.
Acknowledgements
The authors acknowledge Government of the Russian Federation (grant no. 14.Z50.31.0004 to support scientific research projects implemented under the supervision of leading scientists at Russian institutions and Russian institutions of higher education), RFBR research project no. 15-29-01172 ofi_m and the Marie-Curie IRSES project “DINaMIT” (PIRSES-GA-2013-612673). B. V. Parakhonskiy is grateful for support by the FWO.
Notes and references
- A. Campion and P. Kambhampati, Chem. Soc. Rev., 1998, 27, 241 RSC.
- P. G. Etchegoin and E. C. Le Ru, Phys. Chem. Chem. Phys., 2008, 10, 6079–6089 RSC.
- J. J. Giner-Casares, M. Henriksen-Lacey, M. Coronado-Puchau and L. M. Liz-Marzán, Mater. Today, 2015, 1–10 Search PubMed.
- K. V. Kong, U. S. Dinish, W. K. O. Lau and M. Olivo, Biosens. Bioelectron., 2014, 54, 135–140 CrossRef CAS PubMed.
- D. Sun, G. Qi, S. Xu and W. Xu, RSC Adv., 2016, 6, 53800–53803 RSC.
- X. Wu, P. Fu, W. Ma, L. Xu, H. Kuang and C. Xu, RSC Adv., 2015, 5, 73395–73398 RSC.
- Y. Zhao, L. Liu, H. Kuang, L. Wang and C. Xu, RSC Adv., 2014, 4, 56052–56056 RSC.
- M. Liu, Z. Wang, L. Pan, Y. Cui and Y. Liu, Biosens. Bioelectron., 2015, 69, 142–147 CrossRef CAS PubMed.
- M. Delcea, N. Sternberg, A. M. Yashchenok, R. Georgieva, H. Bäumler, H. Möhwald and A. G. Skirtach, ACS Nano, 2012, 6, 4169–4180 CrossRef CAS PubMed.
- P. Taladriz-Blanco, L. Rodríguez-Lorenzo, M. Sanles-Sobrido, P. Hervés, M. A. Correa-Duarte, R. A. Álvarez-Puebla and L. M. Liz-Marzán, ACS Appl. Mater. Interfaces, 2009, 1, 56–59 CAS.
- Y. Sun, K. Liu, J. Miao, Z. Wang, B. Tian, L. Zhang, Q. Li, S. Fan and K. Jiang, Nano Lett., 2010, 10, 1747–1753 CrossRef CAS PubMed.
- J. Perumal, K. V. Kong, U. S. Dinish, R. M. Bakker and M. Olivo, RSC Adv., 2014, 4, 12995 RSC.
- X. Wang, C. Wang, L. Cheng, S.-T. Lee and Z. Liu, J. Am. Chem. Soc., 2012, 134, 7414–7422 CrossRef CAS PubMed.
- R. Singhal, Z. Orynbayeva, R. V. Kalyana Sundaram, J. J. Niu, S. Bhattacharyya, E. A. Vitol, M. G. Schrlau, E. S. Papazoglou, G. Friedman and Y. Gogotsi, Nat. Nanotechnol., 2011, 6, 57–64 CrossRef CAS PubMed.
- S. Mondal, U. Rana and S. Malik, ACS Appl. Mater. Interfaces, 2015, 7, 10457–10465 CAS.
- R. Gunawidjaja, S. Peleshanko, H. Ko and V. V. Tsukruk, Adv. Mater., 2008, 20, 1544–1549 CrossRef CAS.
- X. Xu, K. Kim, H. Li and D. L. Fan, Adv. Mater., 2012, 24, 5457–5463 CrossRef CAS PubMed.
- R. A. Alvarez-Puebla, A. Agarwal, P. Manna, B. P. Khanal, P. Aldeanueva-Potel, E. Carbó-Argibay, N. Pazos-Pérez, L. Vigderman, E. R. Zubarev, N. A. Kotov and L. M. Liz-Marzán, Proc. Natl. Acad. Sci. U. S. A., 2011, 108, 8157–8161 CrossRef CAS PubMed.
- G. Q. Wallace, M. S. Zuin, M. Tabatabaei, P. Gobbo, F. Lagugné-Labarthet and M. S. Workentin, Analyst, 2015, 140, 7278–7282 RSC.
- P. Dawson, J. A. Duenas, M. G. Boyle, M. D. Doherty, S. E. J. Bell, A. M. Kern, O. J. F. Martin, A. S. Teh, K. B. K. Teo and W. I. Milne, Nano Lett., 2011, 11, 365–371 CrossRef CAS PubMed.
- C. Feng, Y. Zhao and Y. Jiang, RSC Adv., 2015, 5, 4578–4585 RSC.
- H. Ko, S. Chang and V. V. Tsukruk, ACS Nano, 2009, 3, 181–188 CrossRef CAS PubMed.
- Y. Xie, S. Yang, Z. Mao, P. Li, C. Zhao, Z. Cohick, P. H. Huang and T. J. Huang, ACS Nano, 2014, 8, 12175–12184 CrossRef CAS PubMed.
- J. Chen, J. Abell, Y. Huang and Y. Zhao, Lab Chip, 2012, 12, 3096–3102 RSC.
- L.-L. Qu, D.-W. Li, J.-Q. Xue, W.-L. Zhai, J. S. Fossey and Y.-T. Long, Lab Chip, 2012, 12, 876–881 RSC.
- Y. Qian, G. Meng, Q. Huang, C. Zhu, Z. Huang, K. Sun and B. Chen, Nanoscale, 2014, 6, 4781 RSC.
- J. A. Webb, J. Aufrecht, C. Hungerford and R. Bardhan, J. Mater. Chem. C, 2014, 2, 10446–10454 RSC.
- J. Shao, L. Tong, S. Tang, Z. Guo, H. Zhang, P. Li, H. Wang, C. Du and X. Yu, ACS Appl. Mater. Interfaces, 2015, 7(9), 5391–5399 CAS.
- T. Szymborski, E. Witkowska, W. Adamkiewicz, J. Waluk and A. Kamińska, Analyst, 2014, 139, 5061–5064 RSC.
- C.-L. Zhang, K.-P. Lv, H.-P. Cong and S.-H. Yu, Small, 2012, 8, 648–653 CrossRef CAS PubMed.
- W. Tang, D. B. Chase and J. F. Rabolt, Anal. Chem., 2013, 85, 10702–10709 CrossRef CAS PubMed.
- W. Gao, G. Chen, W. Xu, C. Yang and S. Xu, RSC Adv., 2014, 4, 23838–23845 RSC.
- A. N. Severyukhina, B. V. Parakhonskiy, E. S. Prikhozhdenko, D. A. Gorin, G. B. Sukhorukov, H. Möhwald and A. M. Yashchenok, ACS Appl. Mater. Interfaces, 2015, 7, 15466–15473 CAS.
- T. Yang, H. Yang, S. J. Zhen and C. Z. Huang, ACS Appl. Mater. Interfaces, 2015, 7, 1586–1594 CAS.
- B. Khlebtsov, V. Zharov, A. Melnikov, V. Tuchin and N. G. Khlebtsov, Nanotechnology, 2006, 17, 5167–5179 CrossRef CAS.
- A. Schweikart, N. Pazos-Pérez, R. A. Alvarez-Puebla and A. Fery, Soft Matter, 2011, 7, 4093 RSC.
- B. N. Khlebtsov, V. A. Khanadeev, E. V. Panfilova, D. N. Bratashov and N. G. Khlebtsov, ACS Appl. Mater. Interfaces, 2015, 7, 6518–6529 CAS.
- B. N. Khlebtsov, V. A. Khanadeev, E. V. Panfilova, D. N. Bratashov and N. G. Khlebtsov, ACS Appl. Mater. Interfaces, 2015, 7, 6518–6529 CAS.
- M. Kahraman, M. M. Yazıcı, F. Şahin and M. Çulha, Langmuir, 2008, 24, 894–901 CrossRef CAS PubMed.
- Z. Movasaghi, S. Rehman and I. U. Rehman, Appl. Spectrosc. Rev., 2007, 42, 493–541 CrossRef CAS.
- K. C. Schuster, I. Reese, E. Urlaub, J. R. Gapes and B. Lendl, Anal. Chem., 2000, 72, 5529–5534 CrossRef CAS PubMed.
- Y. Liu, Y.-R. Chen, X. Nou and K. Chao, Appl. Spectrosc., 2007, 61, 824–831 CrossRef CAS PubMed.
- M. Çulha, M. M. Yazıcı, M. Kahraman, F. Şahin and S. Kocagöz, J. Nanotechnol., 2012, 2012, 1–7 Search PubMed.
- A. Walter, A. März, W. Schumacher, P. Rösch and J. Popp, Lab Chip, 2011, 11, 1013 RSC.
- H. Zhou, D. Yang, N. P. Ivleva, N. E. Mircescu, R. Niessner and C. Haisch, Anal. Chem., 2014, 86, 1525–1533 CrossRef CAS PubMed.
- T.-Y. Liu, K.-T. Tsai, H.-H. Wang, Y. Chen, Y.-H. Chen, Y.-C. Chao, H.-H. Chang, C.-H. Lin, J.-K. Wang and Y.-L. Wang, Nat. Commun., 2011, 2, 538 CrossRef PubMed.
|
This journal is © The Royal Society of Chemistry 2016 |