DOI:
10.1039/C6RA18504E
(Paper)
RSC Adv., 2016,
6, 90982-90992
Arene ruthenium(II) complexes with chalcone, aminoantipyrine and aminopyrimidine based ligands: synthesis, structure and preliminary evaluation of anti-leukemia activity†
Received
20th July 2016
, Accepted 14th September 2016
First published on 16th September 2016
Abstract
A series of arene ruthenium(II) (Ru) complexes with N-monodentate (AAP) and N,O- and N,N-bidentate chelating ligands (AAPS, ADABS, AAPPA and P2P) have been synthesized and evaluated for preliminary antileukemia activity. Single crystal X-ray studies of AAP-Ru, ADABS-Ru and P2P-Ru indicates that Ru(II) adopted a typical “three-leg piano-stool” geometry with pseudo-tetrahedral (AAP-Ru) and quasi-octahedral arrangements (ADABS-Ru and P2P-Ru). Supramolecular interactions (C–H⋯Cl, N–H⋯Cl and π–π) in the crystal lattice of AAP-Ru and ADABS-Ru resulted in a dimeric structure whereas P2P-Ru showed a supramolecular 1D chain. Importantly, preliminary in vitro anticancer activities for Ru-arene complexes have been evaluated against K562 (human chronic myeloid leukemia cell line) by means of MTT assay that showed strong anti-leukemic activity for AAPS-Ru (IC50 = 12.46 ± 0.47 μM) and P2P-Ru (IC50 = 11.8 ± 0.49 μM). The mechanistic studies indicated reactive oxygen species (ROS) mediated selective leukemic cells death without affecting the normal cells by AAPS-Ru. The initiation of apoptosis by AAPS-Ru treatment, particularly TNF-α induced cell death, was confirmed by pre-treatment with a selective TNF-α inhibitor.
Introduction
In recent years, there has been a growing interest in the development of transition metal based drugs for application of chemotherapeutic agents. In this perspective, platinum-based anti-cancer drugs have primarily been used in the clinic as chemotherapeutic agents.1 However, extensive side effects and cancer cell resistance, renal toxicity and neurotoxicity of platinum based drugs have often restricted their application potential.2 These limitations of cisplatin drugs have led to intensive research on non-platinum based metal complexes for anticancer activity. In particular, organometallic complexes of iron, ruthenium and osmium showed promising activity.3 Of that, arene ruthenium(II) complexes are highly promising materials and considered as potential alternatives to platinum chemotherapeutic agents.4 In particular, ruthenium complexes exhibited admiring properties like acceptable antitumor activity, multiple stable oxidation states under physiological conditions, light-activated reactions with DNA, high delivery to cancer cells, low side effects, high cytotoxicity toward cancer and lower toxicity toward healthy cells.5,2f Thus, different types of ruthenium(II) arene complexes have been investigated as a potential anti-cancer drug candidate.6 Sadler et al. prepared cationic arene ruthenium complex and reported very good anticancer activity both in vitro and in vivo.7
Ru(II)-arene chloroquine showed noticeable anticancer activity against various tumor cell lines.8 Curcumin based arene-Ru(II) complex displayed high in vitro activity against breast MCF7 and ovarian A2780 cell lines.9 The incorporation of carborane and ferrocene with Ru(II) arene complexes significantly increases apoptosis in human lung cancer HCC827 cell line.10 The intensive research effort of ruthenium complexes resulted in developing two strong anticancer active ruthenium(III) compounds, namely KP1019 and NAMI-A. It is noteworthy that, arene-Ru(II) complexes RAPTA-C (developed by Dyson and coworkers) and RM175 (developed by Sadler and coworkers) displayed strong anticancer activity in vivo also (Scheme 1).11–15 Further, the prototype compound RAPTA-C reduces the growth of primary tumors in preclinical models for ovarian and colorectal carcinomas as well as it has shown moderate effects on solid tumour metastases.11 The ligand scaffolds plays important role on the arene ruthenium complexes in controlling the anticancer activities such as improving water solubility and cell permeability. For example, incorporation of bioactive ligand scaffolds, such as flavonoids, isoflavonoids, naphthoquinones, staurosporine or thiosemicarbazone to arene Ru(II) complexes enhanced anticancer activities.16 Pyrimidine analogues have also been evaluated as viable anti-tumour drugs.17 The aminoantipyrine derivatives exhibited wide range of biological activities such as, antimicrobial, fungicidal anti-inflammatory properties.18 In this manuscript, we report the synthesis of Ru-arene complex with antipyrine, chalcone and pyrimidine based mono and bidendate ligands as new chemotherapeutic agents and preliminary screening for anti-cancer activities against leukaemia cell. The structure of Ru-arene complexes (AAP-Ru, AAPPA-Ru, AAPS-Ru, ADABS-Ru, P2P-Ru and BPY-Ru) have been confirmed by spectroscopy (1H NMR, 13C NMR FT-IR and mass spectroscopy) and X-ray diffraction methods. Further anti-cancer activities of Ru-arene complexes have been evaluated against K562 (human chronic myeloid leukaemia cell line) by means of MTT assay in comparison with 5-fluorouracil (5-FU) as a control anticancer drug. Interestingly, AAPS-Ru, antipyrine based N,O-bidendate ligand coordinated complex and pyrimidine based positively charged P2P-Ru complex exhibited strong anticancer activities compared to other complexes. AAPS-Ru anticancer activity has also been tested with human peripheral blood mononuclear cells (PBMC) in vitro to assess its effect on normal healthy cell. The detailed mechanistic studies suggest that AAPS-Ru facilitated ROS mediated leukemic cell death selectively without affecting the normal cells while control drug kills the normal cell also.
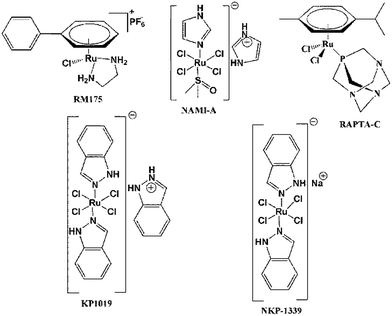 |
| Scheme 1 Structure of ruthenium(II) and ruthenium(III) complexes exhibited strong anticancer activity. | |
Experimental
Materials and methods
Histopaque 1077, acridine orange, ethidium bromide (EtBr), 2,7-dichlorofluorescein diacetate (DCFH2-DA), pentoxifylline (POF), N-acetyl-L-cysteine (NAC), 5-fluorouracil, cell culture grade DMSO were procured from Sigma (St. Louis, MO, USA). Fetal bovine serum (FBS), penicillin, streptomycin, gentamicin were purchased from GIBCO, Invitrogen. RPMI 1640, MTT, was purchased from Himedia, India. TNF-α and IL-10 ELISA sets were purchased from R&D systems. All other chemicals were from Merck-Millipore, SRL and Himedia were of the highest purity grade available. All other reagents and solvents were obtained from commercial suppliers (Aldrich, AlfaAesar, and TCI Chemicals) and used as received; solvents were purified from appropriate drying agents when necessary. All the reactions were carried out under nitrogen atmosphere. The compounds, 4-(2-hydroxybenzylideneamino)-2,3-dimethyl-1-phenyl-1,2-dihydropyrazol-5-one (AAPS),19 1-(4-aminophenyl)-3-(4-(dimethylamino)phenyl)prop-2-en-1-one (ADAB)20 and 4-(pyridine-2-yl)pyrimidin-2-amine (P2P)21 were synthesized as previously described in the literature, are also presented in the ESI.† The complex (η6-p-cymene)(2,2-bipyridine)chloro ruthenium(II) chloride (BPY-Ru) was synthesized based on reported in literature.22 Absorption and fluorescence spectra were recorded using Perking Elmer Lambda 1050 and Jasco fluorescence spectrometer-FP-8200 instruments. FT-IR spectra were measured using a Shimadzu IRAffinity-1S spectrophotometer with KBr pellets.
Synthesis and characterization data
Synthesis of Ru(η6-p-cymene)Cl2(4-amino antipyrine) (AAP-Ru). [(η6-p-Cymene)RuCl2]2 (50 mg, 0.08 mmol) was added to a solution of 4-aminoantipyrine (33 mg, 0.16 mmol) in degassed dichloromethane (5 mL) under N2 atmosphere. The mixture was allowed to stir at room temperature for 3 h. The completion of the reaction was monitored by TLC. After completion of the reaction, the solvent was removed under vacuum and the solid residue was washed with n-hexane/diethyl ether and dried under vacuum that afforded red solid. The reaction mixture was filtered, and ether was allowed to diffuse into the filtrate for a week. Orange crystals of AAP-Ru formed which were filtered off, washed with diethyl ether, and dried in air at room temperature. The crystals were suitable for single crystal X-ray crystallography. Yield: 0.115 g (92%). 1H NMR (400 MHz, CDCl3): δ 9.26 (s, 2H), 7.55–7.42 (m, 2H), 7.41–7.28 (m, 1H), 7.21 (d, J = 7.4 Hz, 1H), 5.93 (t, J = 5.8 Hz, 1H), 5.72 (dd, J = 34.3, 5.4 Hz, 1H), 5.37 (qd, J = 18.8, 13.0 Hz, 3H), 4.56–3.22 (m, 2H), 3.18–2.97 (m, 3H), 2.94–2.67 (m, 1H), 2.43–2.32 (m, 3H), 2.25–2.03 (m, 3H), 1.46–1.05 (m, 6H). 13C NMR (400 MHz, CDCl3): δ 168.13, 161.07, 146.48, 145.33, 134.39, 131.46, 129.96, 129.74, 127.49, 126.25, 124.07, 116.42, 113.44, 103.19, 100.68, 95.81, 82.44, 81.20, 79.57, 36.41, 34.46, 31.01, 30.79, 22.74, 22.77, 21.97, 18.74, 12.20, 11.28. IR (KBr): ν = 3178 (m), 29
549 (m), 1672 (s), 1566 (s), 1494 (s), 1296 (s), 1130 (s), 760 (s), 698 (s), 576 (m), 497 (m).
Synthesis of 2,3-dimethyl-1-phenyl-4-(pyridine-2-ylmethyl eneamino)-1,2-dihydropyrazol-5-one (AAPPA). 4-Aminoantipyrine (200 mg, 0.98 mmol) and 2-pyridinecarboxalaldehyde (105 mg, 0.98 mmol) were refluxed in ethanol (15 mL) for 5 h. The solvent was evaporated and dried under vacuum. The yellow solid product was obtained. Yield: 0.256 g (89%). 1H NMR (400 MHz, CDCl3): δ 9.76 (d, J = 21.4 Hz, 1H), 8.69 (d, J = 4.7 Hz, 1H), 7.99 (d, J = 7.9 Hz, 1H), 7.74 (td, J = 7.8, 1.5 Hz, 1H), 7.52–7.45 (m, 2H), 7.40 (d, J = 7.5 Hz, 2H), 7.33 (t, J = 7.3 Hz, 1H), 7.27 (t, J = 3.3 Hz, 1H), 3.19 (s, 3H), 2.52 (s, 3H). 13C NMR (100 MHz, CDCl3): δ 160.37 (s), 156.59 (s), 156.17 (s), 152.46 (s), 149.71 (s), 136.28 (s), 134.65 (s), 129.26 (s), 129.03 (s), 127.15 (s), 124.79 (s), 123.94 (s), 122.84 (s), 121.60 (s), 118.11 (s), 76.80 (s), 35.58 (s), 10.25 (s). IR (KBr): ν = 3041 (m), 1645 (s), 1566 (s), 1457 (m), 1414 (m), 1305 (s), 1134 (m), 764 (s), 567 (m).
Synthesis of (η6-p-cymene)(2,3-dimethyl-1-phenyl-4-(pyridine-2-ylmethyleneamino)-1,2-dihydropyrazol-5-one)chlororuthenium(II) chloride (AAPPA-Ru). Ligand AAP-PA (50 mg, 0.17 mmol) was suspended in dichloromethane (6 mL), followed by [Ru(p-cymene)Cl2]2 (52 mg, 0.08 mmol) was added. The reaction mixture was stirred for 3 h at room temperature. After completion of reaction, the solvent was removed in vacuum under reduced pressure and the solid residue was washed with n-hexane/ether and dried under vacuum, obtained as a red solid. Yield: 0.093 g (95%). 1H NMR (400 MHz, CDCl3): δ 9.73 (d, J = 4.6 Hz, 1H), 8.97 (s, 1H), 8.24 (d, J = 7.6 Hz, 1H), 7.98 (t, J = 7.5 Hz, 1H), 7.84–7.67 (m, 1H), 7.52 (d, J = 7.3 Hz, 2H), 7.48 (d, J = 7.5 Hz, 2H), 7.41 (t, J = 7.2 Hz, 1H), 6.15 (d, J = 5.7 Hz, 1H), 6.06 (dd, J = 8.6, 6.3 Hz, 2H), 5.87 (d, J = 5.6 Hz, 1H), 3.38 (s, 3H), 2.69 (d, J = 9.1 Hz, 3H), 2.57 (s, 1H), 2.18 (s, 3H), 1.02 (dd, J = 13.8, 6.8 Hz, 6H). IR (KBr): ν = 3412 (w), 2964 (m), 1645 (s), 1491 (m), 1309 (m), 1236 (m), 1029 (m), 773 (s), 698 (m), 589 (m).
Synthesis of (η6-p-cymene)(4-(2-hydroxybenzylidene amino)-2,3-dimethyl-1-phenyl-1,2-dihydropyrazol-5-one) chlororuthenium(II) (AAPS-Ru). Ligand AAPS (50 mg, 0.16 mmol) was suspended in dichloromethane (6 mL) and triethyamine (23 mg, 0.16 mmol) and [Ru(p-cymene)Cl2]2 (50 mg, 0.08 mmol) were added. The reaction mixture was stirred for 3 h at room temperature. After completion of reaction, the solvent was removed under vacuum and the solid residue was washed with n-hexane/ether and dried under vacuum, obtained as a red solid. Yield: 0.089 g (93%). 1H NMR (400 MHz, CDCl3): δ 7.64 (s, 1H), 7.50 (t, J = 7.7 Hz, 2H), 7.42 (d, J = 7.8 Hz, 2H), 7.33 (t, J = 7.4 Hz, 1H), 7.16 (t, J = 7.4 Hz, 1H), 7.02–6.78 (m, 2H), 6.36 (t, J = 7.3 Hz, 1H), 5.64 (dd, J = 20.7, 5.9 Hz, 2H), 5.47–5.17 (m, 2H), 4.89 (d, J = 5.8 Hz, 1H), 3.10 (s, 3H), 2.73 (dt, J = 13.8, 6.9 Hz, 1H), 2.50–2.40 (m, 3H), 2.11–1.97 (m, 3H), 1.32 (d, J = 7.3 Hz, 6H). 13C NMR (400 MHz, CDCl3): δ 169.44, 166.00, 161.48, 149.51, 135.78, 134.84, 132.00, 130.44, 129.61, 129.40, 127.40, 124.77, 124.28, 122.95, 118.51, 114.29, 102.28, 95.77, 85.27, 83.27, 83.84, 81.49, 79.81, 45.99, 36.25, 30.57, 22.34, 21.90, 18.29, 11.90, 8.76. IR (KBr): ν = 3437 (w), 2935 (m), 2677 (m), 2494 (m), 1660 (m), 1602 (s), 1525 (s), 1441 (s), 1323 (m), 1286 (m), 1145 (s), 1031 (m), 760 (s).
Synthesis of 1-(4-((Z)-2-hydroxybenzylideneamino)phenyl)-3-(4-(dimethylamino)phenyl)prop-2-en-1-one (ADABS). Compound ADABS was prepared from the reaction of chalcone derivative, 1-(4-((Z)-2-hydroxybenzylideneamino)phenyl)prop-2-en-1-one (200 mg, 0.74 mmol) and salicylaldehyde (90 mg, 0.74 mmol) were refluxed in methanol (25 mL) for 6 h. Then, the reaction mixture was cooled to room temperature; the resulting precipitate was filtered off and dried under vacuum. The product was obtained as a yellow crystalline product. 1H NMR (400 MHz, CDCl3): δ 11.61 (s, 1H), 6.93 (s, 1H), 6.69 (d, J = 8.4 Hz, 2H), 6.64 (s, 2H), 6.39 (s, 2H), 6.37 (s, 2H), 6.15 (s, 2H), 6.01 (s, 2H), 5.85 (s, 2H), 1.66 (s, 6H). 13C NMR (100 MHz, CDCl3): δ 163.89 (s), 161.18 (s), 151.96 (s), 145.98 (s), 145.23 (s), 133.76 (s), 132.68 (s), 130.86 (s), 130.45 (d, J = 15.7 Hz), 129.80 (d, J = 13.6 Hz), 121.30 (s), 121.01 (s), 119.31 (s), 117.39 (s), 116.85 (s), 116.47 (s), 111.85 (s), 111.55 (s), 40.17 (s). IR (KBr): ν = 3420 (w), 2900 (m), 1645 (s), 1558 (s), 1540 (s), 1367 (s), 1224 (m), 1157 (s), 806 (s), 7549 (m), 518 (m). Yield: 0.240 g (87%). ESI-MS (CHCl3) m/z calcd for C24H22N2O2: 370.44 found: 371.17.
Synthesis of (η6-p-cymene)(1-(4-((Z)-2-hydroxybenzylidene amino)phenyl)-3-(4-(dimethylamino)phenyl)prop-2-en-1-one)chlororuthenium(II) (ADABS-Ru). Ligand ADABS (59 mg, 0.13 mmol) was suspended in dichloromethane (10 mL) and triethyamine (23 mg, 0.16 mmol) was added. The solution was stirred at room temperature for 15 min and [Ru(p-cymene)Cl2]2 (50 mg, 0.065 mmol) was added The reaction mixture was stirred for 3 h at room temperature. After completion of reaction, the solvent was removed under vacuum and the solid residue was washed with n-hexane/ether and dried under vacuum, obtained as a red solid. Yield: 0.093 g (91%) The reaction mixture was filtered, and ether was allowed to diffuse into the filtrate for a week. Red crystals of compound ADABS-Ru formed which were filtered off, washed with diethyl ether, and dried in air at room temperature. The crystals were suitable for single crystal X-ray crystallography. 1H NMR (400 MHz, CDCl3): δ 8.36 (s, 1H), 8.11 (dd, J = 23.7, 8.5 Hz, 2H), 7.99–7.70 (m, 4H), 7.70–7.52 (m, 2H), 7.41 (dd, J = 15.4, 6.1 Hz, 1H), 7.28 (d, J = 3.1 Hz, 1H), 7.01 (dd, J = 12.7, 8.3 Hz, 1H), 6.84–6.66 (m, 3H), 6.46 (t, J = 6.8 Hz, 1H), 5.66–4.87 (m, 4H), 4.26 (d, J = 5.6 Hz, 1H), 3.11–3.07 (m, 6H), 2.66 (dt, J = 13.8, 7.1 Hz, 1H), 2.19 (dd, J = 13.1, 6.3 Hz, 3H), 1.39 (t, J = 7.3 Hz, 6H). IR (KBr): ν = 3445 (m), 2677 (w), 1589 (s) 1524 (s), 1439 (s), 1332 (s), 1224 (m), 1165 (m), 1031 (m), 812 (w), 661 (w), 416 (w).
Synthesis of (η6-p-cymene)(4-(pyridine-2-yl)pyrimidin-2-amine)chlororuthenium(II) tetrafluoroborate (P2P-Ru). 0.1 g,0.58 mmol of 4-(pyridine-2-yl)pyrimidin-2-amine was suspended in 15 mL methanol and 0.178 g, 0.29 mmol of dichloro(p-cymene)Ru(II)dimer and followed by the addition of 0.032 g, 0.29 mmol of NaBF4 allow to stir this reaction at RT for 3 h. The resulting solution was evaporated in vacuum under reduced pressure. Yield: 0.240 g (96%) the complex was crystallized by slow diffusion of ether into methanol; dark red crystals were formed after 2 days. 1H NMR (300 MHz, D2O): δ 9.23 (d, J = 5.5 Hz, 1H), 8.39 (d, J = 5.0 Hz, 1H), 8.14 (d, J = 8.1 Hz, 1H), 8.01 (t, J = 7.8 Hz, 1H), 7.78–7.54 (m, 1H), 7.39 (d, J = 5.0 Hz, 1H), 6.10 (d, J = 6.1 Hz, 1H), 5.80 (s, 2H), 5.68 (d, J = 6.1 Hz, 1H), 4.70 (s, 2H). 2.10 (d, J = 11.6 Hz, 3H), 0.76 (dd, J = 27.6, 6.9 Hz, 6H). IR (KBr): ν = 3383 (w), 3240 (m), 3136 (m), 1606 (s), 1550 (s), 1481 (s), 1352 (m), 1222 (m), 1120 (m), 1028, 779, 540, 462.
Biological studies
Drug preparation. All the ruthenium complexes, 5-FU, and others inhibitors were dissolved in cell culture grade DMSO to prepare the stock solutions which were freshly diluted in RMPI 1640 to prepare the working concentration. All the experiments were done in triplicate and repeated at least two times.
K562 cell culture and PBMC isolation. K562 cells, obtained from National Centre for Cell Sciences (NCCS), Pune, India, were cultured in RMPI-1640 media supplementated with 10% fetal bovine serum (Gibco) and 1× pen-strep antibiotics in T25 cell culture flask with regular passage as described earlier.23 The confluent culture of K562 cells were used in all experiments. PBMCs were isolated from heparinised blood of healthy donor with prior consent. 1
:
1 diluted blood samples with PBS were layered with Histopaque 1077 (Sigma) followed by centrifuged at 1500 rpm for 30 min. The buffy coat was collected carefully washed three times with PBS and resuspended in complete culture media and used in different experiments.23,24
Cell cytotoxicity. MTT (3-[4,5-dimethylthiazol-2yl]-2,5-diphenyltetrazolium bromide) assays were performed to estimate cell cytotoxicity of K562 cells and PBMCs in the presence or absence of ruthenium complexes (0–100 μM) at different time point (0–72 h) using 2 × 104 cells per well as initial cell number in a 96 well microplate. The absorbance of MTT formazan product was measured using a microplate reader (Biotek, Synergy H1, India) at 570 nm. Cell viability was calculated as a relative percentage to untreated cells.
Inhibitors assay. K562 cells were pre-treated with 60 μM necrostatin-1 (inhibitor of necrosis), 2 mM pentoxifylline (a potent TNF-α inhibitor), 30 μM SP600125 (JNK inhibitor), 10 mM NAC and then treated with AAPS-Ru (12.5 μM) for 24 h. After the treatment schedule, the cells were washed with culture medium and cell viability was determined by MTT assay as described earlier.
Intracellular measurement of reactive oxygen species (ROS). ROS generation was measured using DCFH2-DA as described previously23 using H2O2 (100 mM) as positive control. Fluorescence images were also taken by phase contrast microscope (NIKON, Japan). All the experiments were done in triplicate and repeated at least two times.
Biochemical parameters. Lactic dehydrogenase (LDH) releases assay was done using a spectrophotometric LDH assay kit (Reckon Diagnostics Pvt. Ltd, India) to evaluate cell membrane integrity. Malondialdehyde (MDA), the extent of lipid peroxidation, was estimated as the concentration of thiobarbituric acid reactive product according to the previously describe by Kar Mahapatra et al.25 Reduced glutathione estimation in cell lysate was performed by Ellman's reagent according to the previously described method by Kar Mahapatra et al.25 The oxidized glutathione level was measured after derivatization of GSH with 2-vinyl pyridine followed by using Ellman's reagent according to the previously described method by Kar Mahapatra et al.25 Protein was determined according to Lowry et al., 1951 using bovine serum albumin as standard.26
TNF-α and IL-10 release assay by ELISA. K562 cells and PBMCs were treated with AAPS-Ru (12.5 μM) for 24 h. TNF-α and IL-10 release by K562 cells and PBMC were determined from cell free supernatants of culture media (200 μL) with an enzyme-linked immunosorbent assay (ELISA) (R&D systems) according to the manufacturer's instructions.
Apoptotic morphology by AO/EtBr staining. Apoptotic morphology was detected by K562 cells and PBMC were treatment followed by AO/EtBr staining for 24 h. After the treatment with AAPS-Ru (12.5 μM), K562 cells and PBMCs were collected, washed with cold PBS, and then stained with a mixture of AO (100 μg mL−1) and Et–Br (100 μg mL−1) at room temperature in dark for 5 min. After that cells were washed with PBS, and thin single mono layer films were prepared and observed under fluorescence microscope (Nikon, Japan).
Statistical analysis. Each of the above assays was performed in triplicate repeated at least two times. The data were expressed as mean ± standard deviation. Comparisons between the means of control and treated group were made by two-way ANOVA test (using a statistical package, Origin 6.1, Northampton, MA 01060 USA) with multiple comparison t-tests.
Results and discussion
Synthesis and structural characterization
The arene-ruthenium(II) complexes were synthesized using biologically active N-monodentate and bidentate N,N-, N,O-chelating ligand scaffolds (Scheme 2). Bidendate N,O- and N,N-chelating Schiff base ligands, AAPS and AAPPA, ADABS have been synthesized by reacting 4-aminoantipyrine and amino-chalcone (1-(4-((Z)-2-hydroxybenzylideneamino)phenyl)prop-2-en-1-one with relevant aldehydes (salicylaldehyde and pyridine-2-carbaldehyde) in refluxing alcohol (ethanol/methanol) (Scheme S1, S5 and S6†). The reaction of dimeric ruthenium precursor [Ru(η6-p-cymene)(μ-Cl)Cl]2 with different ligands AAP, AAPPA, AAPS, ADABS and P2P) produced corresponding arene Ru(II) complexes AAP-Ru, AAPPA-Ru, AAPS-Ru, ADABS-Ru and P2P-Ru respectively (Scheme S7–S11†). 1H and 13C NMR spectra of arene Ru(II) complexes recorded in CDCl3 clearly indicate the coordination of ligand with Ru(II) (Fig. S1–S14†). The 1H NMR spectra of arene Ru(II) complexes showed proton signals in the range of δ = 5.37–6.15 ppm due to p-cymene ring protons. The proton chemical shifts in the range between δ = 0.76–1.39 ppm and 2.10–2.32 correspond to isopropyl and methyl protons, respectively. AAP-Ru, AAPPA-Ru, AAPS-Ru, and ADABS-Ru showed proton signals in the range of 6.36–7.52 ppm corresponding to benzene moiety. The ligands AAPS and ADABS showed 1H NMR signals of –OH proton at 9.80 ppm and 11.61 ppm respectively, but the phenolic proton resonance has been absent in their corresponding metal complexes AAPS-Ru and ADABS-Ru which confirmed the coordination of hydroxyl group with ruthenium metal centre. Besides, in complex P2P-Ru, the protons of the pyrimidine and pyridine core shifted to downfield between 7.38–9.24 ppm and NH2 protons shifted up-field to 4.70 ppm compared to free P2P ligand. The 1H NMR spectra for arene Ru(II) complexes showed downfield shift compared to their corresponding free ligands, which confirmed the coordination of AAP, AAPPA, AAPS, ADABS and P2P ligands to Ru(II) metal centres. The 13C NMR spectrum of the arene Ru(II) complexes AAP-Ru, AAPPA-Ru, AAPS-Ru, and ADABS-Ru showed chemical shifts in the range of 160–161 ppm corresponding to –C
O carbon atom. Further, the complexes showed carbon atom signals between 161–169 ppm owing to the presence of –C
N feature. The aromatic carbons are resonated between 108–156 ppm. FT-IR spectra also confirmed the characteristic stretching frequencies and functional groups present in the free ligands as well as complexes (Fig. S15–S23†). For instance, compound AAP-Ru showed ν(NH2) stretching vibration bands at 3178 cm−1 and 2955 cm−1 that are at lower wavenumbers in comparison to the free ligand of AAP bands 3431 cm−1 3325 cm−1, thus authenticates the coordination of AAP with Ru metal centre. Compound AAPPA exhibited ν(C
N) stretching vibration band at 1566 cm−1, upon coordination the ν(C
N) stretching band shifted to lower wave number considerably. In compound AAPS, the ν(C
N) band appears at 1593 cm−1, that is shifted to 1525 cm−1 after coordination with Ru centre. Similarly, the IR spectrum of compound ADABS-Ru showed typical ν(C
N) band at 1523 cm−1, which is considerably at lower wave-number compared to free ADABS (1540 cm−1). Complex, P2P-Ru exhibited typical ν(C
N) bands at 1607 cm−1 and after coordination it is shifted to 1550 cm−1. Further, mass analyses of the complexes have also revealed the presence of molecular species. Mass spectral analysis of AAP-Ru is showed molecular ion peak at 474.08, which was assigned to the m/z of the AAP-Ru molecule with loss of a Cl− anion. The AAPPA-Ru is showed main peak at 563.11, which is based on the m/z of the cationic AAPPA-Ru molecule. In case of AAPS-Ru, the molecular ion peak at m/z 542.31 is due to AAPS-Ru molecule with loss of a Cl− anion. The details are depicted in ESI (Fig. S24–S27†). The mass spectral data's are suggested that, the chloride ion, which is attached to arene Ru(II) complexes is more labile.
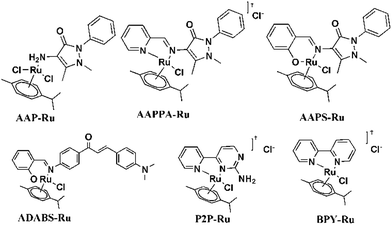 |
| Scheme 2 Structure of the arene ruthenium(II) complexes studied for their anticancer activity. | |
The absorption spectra of ligands, 4-aminoantipyrine (AAP), 2,3-dimethyl-1-phenyl-4-(pyridine-2-ylmethyleneamino)-1,2-dihydropyrazol-5-one(AAPPA), 4-(2-hydroxy benzylidene amino)-2,3-dimethyl-1-phenyl-1,2-dihydropyrazol-5-one (AAPS), and 1-(4-((Z)-2-hydroxybenzylideneamino)phenyl)-3-(4-(dimethylamino)phenyl)prop-2-en-1-one (ADAB-S) and their complexes AAP-Ru, AAPPA-Ru, AAPS-Ru, ADABS-Ru and P2P-Ru have been recorded in CH2Cl2 (Fig. 1). The absorption spectral data are summarized in Table 1. The electronic absorption spectra of free ligand showed peaks in the range between 254–350 nm that can be assigned to intra-ligand n–π* and π–π* transitions.27 The absorption bands in the ranges of 350–400 nm can be assigned to inter-ligand-charge transfer (ILCT) transition. Besides, the absorption spectra of the complexes exhibited low energy bands at 340–425 nm corresponding to the metal to-ligand charge transfer [MLCT = dπ(Ru) → π*(L)]. Further to ascertain the coordination and structure of arene Ru complexes, single crystals were attempted to grow for all compounds. However, only AAP-Ru, ADABS-Ru and P2P-Ru produced quality single crystals by slow diffusion of diethyl ether into CH2Cl2 solution of reaction mixture.
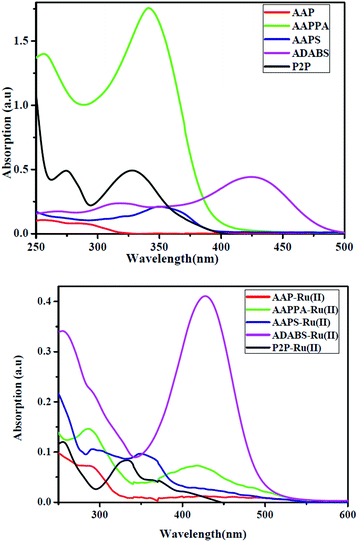 |
| Fig. 1 Absorption spectra of ligands and arene Ru(II) complexes in CH2Cl2 (1 × 10−5 M) at 298 K (a) uncoordinated free ligands (b) arene ruthenium(II) complexes. | |
Table 1 Absorption data for ligands and arene Ru(II) complexes
Free ligands |
λmax (nm) (ε, M−1 cm−1) |
Arene Ru(II) complexes |
λmax (nm) (ε, M−1 cm−1) |
AAP |
255 (8200) |
AAP-Ru |
287 (7500) |
285 (11 000) |
425 (1400) |
AAPPA |
254 (140 000) |
AAPPA-Ru |
286 (14 800) |
341 (170 000) |
418 (7500) |
AAPS |
350 (21 000) |
AAPS-Ru |
290 (10 700) |
348 (9600) |
417 (3000) |
ADABS |
264 (18 500) |
ADABS-Ru |
256 (34 000) |
316 (24 500) |
289 (22 500) |
424 (45 000) |
428 (41 200) |
P2P |
273 (49 700) |
P2P-Ru |
255 (12 200) |
327 (50 000) |
332 (8300) |
367 (4600) |
400 (2100) |
The complex AAP-Ru crystallized in the monoclinic space group C2/c whereas ADABS-Ru and P2P-Ru crystallized in triclinic P
space group (Table 2). A thermal ellipsoidal plot of AAP-Ru, ADABS-Ru and P2P-Ru compounds are shown in Fig. 2. The selected bond lengths and bond angles of compound AAP-Ru are presented in ESI.† The molecular structure of AAP-Ru, ADABS-Ru and P2P-Ru indicates that Ru(II) adopted a typical ‘three leg piano-stool’ conformation (three coordination sites of a quasi-octahedral array and three other donor atoms). In AAP-Ru, two chlorido ligands and NH2 group of 4-aminoantipyrine ligand (N-monodentate with primary terminal amine groups –NH2) act as three legs. In ADABS-Ru, chloride ligand and bidendate hydroxyl oxygen (O1) and imine nitrogen (N1) atoms of chalcone based Schiff-base ligand act as the legs. Whereas in P2P-Ru, chloride ligand and two nitrogens, N1 and N2 from 2-amino-4-(pyridin-2-yl)pyrimidine bidendate, act as the legs. Ru(II) is π-bonded to the p-cymene ring in η6-coordination mode in all three compound. The selected bond lengths and bond angles of AAP-Ru, ADABS-Ru and P2P-Ru are presented in Tables S1–S3.† Ru(II) in AAP-Ru adopted a pseudo-tetrahedral coordination geometry. The bond distance between Ru metal and the centroid of the p-cymene is 1.6554(4) Å. The bond lengths of Ru(1)–Cl(1) and Ru(1)–Cl(2), and Ru(1)–N(1) are found to be 2.4143(7) Å, 2.4047(7) Å and 2.1723(18) Å respectively. The coordination sphere and coordination bond lengths matched with reported arene-Ru(II) complexes.28 The bond angles between Cl(1)–Ru–Cl(2), N(1)–Ru–Cl(1) and N(1)–Ru–Cl(2) are 87.32(1), 82.96(5) and 81.77(5) respectively. The bond parameters around the Ru-center are remarkably similar to RAPTA-C.29 The average Ru–C bond distance is 2.180(2) Å for AAP-Ru. Notably, the two longest Ru–C bonds (Ru(1)–C(13) 2.195(2) and Ru(1)–C(16) 2.199(2); see Table S1†) are trans with respect to Cl atoms as a result of the strong trans influence of these atoms. The monomeric complex AAP-Ru showed N–H⋯Cl hydrogen bonding interactions and produced supramolecular dimeric structure (Fig. 3). The relevant N–H⋯Cl hydrogen bonding distances are 2.622(10) Å [H(1B)⋯Cl(2)] and 2.700(35) Å [H(1A)⋯Cl(1)] and the N–H⋯Cl hydrogen bond angles are 157.13(1) [N(1)–H1B⋯Cl(2)] and 125.01(1) [N(1)–H1A⋯Cl(1)]. The distance between Ru to Ru is 5.940(67) Å, which is similar to the previously studied arene ruthenium(II) complexes.30
Table 2 Crystal data and structure refinement for compounds
Compound |
AAP-Ru |
ADABS-Ru |
P2P-Ru |
Empirical formula |
RuC21H27Cl2N3O |
C34H35ClN2O2Ru |
RuC19H22N4ClBF4 |
Formula weight |
509.42 |
640.16 |
529.73 |
T [K] |
100(2) |
100(2) |
100(2) |
λ [Å] |
0.610 |
0.610 |
0.610 |
Crystal system |
Monoclinic |
Triclinic |
Triclinic |
Space group |
C2/c |
P![[1 with combining macron]](https://www.rsc.org/images/entities/char_0031_0304.gif) |
P![[1 with combining macron]](https://www.rsc.org/images/entities/char_0031_0304.gif) |
a, Å |
12.175(2) |
9.0960(18) |
9.3290(19) |
b, Å |
11.563(2) |
11.993(2) |
10.369(2) |
c, Å |
30.760(6) |
14.283(3) |
11.557(2) |
α, deg |
90 |
74.20(3) |
94.36(3) |
β, deg |
97.18(3) |
75.55(3) |
96.55(3) |
γ, deg |
90 |
89.72(3) |
112.79(3) |
V (Å3) |
4296.4(15) |
1448.5(6) |
1015.0(4) |
Z |
8 |
2 |
2 |
dcalc (mg cm−3) |
1.575 |
1.468 |
1.733 |
μ (mm−1) |
0.657 |
0.444 |
0.632 |
R[I > 2σ(I)] |
R1 = 0.0326 |
R1 = 0.0272 |
R1 = 0.0336 |
wR2 = 0.0859 |
wR2 = 0.0272 |
wR2 = 0.0893 |
R (all data) |
R1 = 0.0378 |
R1 = 0.0314 |
R1 = 0.0398 |
wR2 = 0.0875 |
wR2 = 0.0762 |
wR2 = 0.0916 |
Largest diff. peak/hole [e Å−3] |
1.295/−2.106 |
1.316/−1.427 |
0.646/−2.064 |
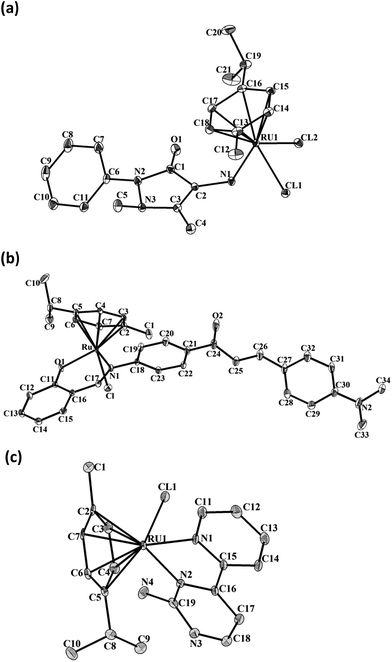 |
| Fig. 2 Thermal ellipsoid plot at the 50% probability level of the arene ruthenium(II) complexes, AAP-Ru (a); ADABS-Ru(b); and P2P-Ru (c). Hydrogen atoms have been omitted for clarity. | |
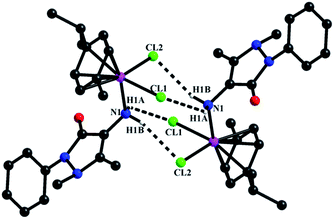 |
| Fig. 3 Supramolecular interactions in the crystal structure of AAP-Ru. Dimers of the 4-aminoantipyrine-Ru(II) complex (AAP-Ru) linking through N–H⋯Cl hydrogen bonds. Hydrogen atoms that are not involved in H-bonding are omitted for clarity. Selected hydrogen bond distance: Cl1–H14 = 2.875 Å. | |
In ADABS-Ru and P2P-Ru, Ru(II) adopted a quasi-octahedral arrangement. Further structural analysis of ADABS-Ru reveals that the Ru(1)–Cl(1) distance is 2.4409(6) Å is slightly longer than reported in literature,31 this might be due to the C–H⋯Cl intermolecular hydrogen bonding interaction. However, Ru(1)–O(1) and Ru(1)–N(1) bond lengths are 2.0668(12) Å and 2.0801(14) Å are comparable to those of reported ruthenium(II)-arene complexes. The bond distance observed between the Ru metal and centroid of the p-cymene is 1.6603(5) Å.27 Interestingly, ADABS-Ru molecule interact with each other through C–H⋯Cl (Cl(1)⋯H(14), 2.875(26) Å) hydrogen-bonding and π–π stacking interaction (3.659(74) Å) (Fig. 4). The combination of both intermolecular hydrogen bonding and π–π interactions leads to dimeric structure of complex. Ru(1)–Cl(1) distance is 2.4040(8) Å and the average Ru–N distances (Ru(1)–N(1), 2.082(2) Å; Ru(1)–N(2), 2.112(2) Å) are comparable to those of ruthenium(II)-arene complexes reported in literature.30 The bond angles for N(1)–Ru(1)–Cl(1) and N(2)–Ru(1)–Cl(1) are 87.60(6)°, and 87.18(6)° respectively. Further hydrogen bonding (both C–H⋯Cl and N–H⋯Cl hydrogen bonding) and π–π stacking interactions in the crystal lattice of P2P-Ru resulted in the formation of infinite 1D chain (Fig. 5).
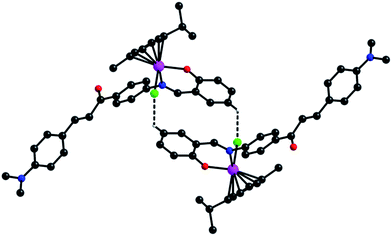 |
| Fig. 4 Supramolecular dimer of ADABS-Ru. The ruthenium complex (ADABS-Ru) dimer linking through CCym–H⋯Cl hydrogen bond. Hydrogen atoms that are not involved in H-bonding are omitted for clarity. Selected hydrogen bond distance: Cl1–H14 = 2.875 Å. Color code: N, blue; O, red; C, gray; Cl, green; Ru, purple. | |
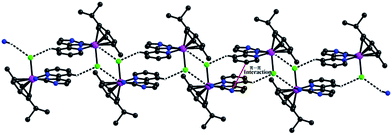 |
| Fig. 5 Supramolecular 1D chain formed by the combination C–H⋯Cl and N–H⋯Cl hydrogen bonding and π–π stacking interaction in the crystal structure of [(η6-p-cymene)-RuCl(P2P)]BF4 (P2P-Ru). The intermolecular hydrogen bonds are shown as dashed lines. π–π interactions: aromatic interatomic distances, C(15)–C(15) = 3.431(42) Å; C(14)–C(16) = 3.774(42) Å; C(15)–C(16) = 3.319(35) Å. | |
In vitro anti-leukaemia activities
The in vitro anticancer activities of Ru-arene complexes were screened against K562, a chronic myeloid leukemia cell line, to screen the best complex for further studies (Table 3). Interestingly, Ru-arene complex based N,O-bidendate Schiff base derived from AAP (AAPS-Ru) and a positively charged bipyridine complex (P2P-Ru) exhibited strong inhibition of cells growth. The IC50 values of anti-leukemic activity for AAPS-Ru and P2P-Ru were found to be 12.46 ± 0.47 μM and 11.8 ± 0.49 μM, respectively (Table 3). It is noted that Ru-arene complex with simple AAP (AAP-Ru) and bipyridine (BPY-Ru) did not show any inhibition of leukemia cells growth even though the later complex is positively charged. In contrast to Ru-arene complex with AAPS ligand, complex prepared from N,O-bidendate Schiff base ligand of chalcone derivative (ADABS) showed only weak activity against leukemia cells. Similarly, Ru-arene complexes of N,N-bidendate Schiff base ligand (AAPPA) also exhibited weak activity. These structure-activity comparison indicate that N,O-bidendate ligand with hydrophilic functionality and N,N′-bidendate ligand with hydrophilic functionality and ionic nature such as positive charge could be suitable for antileukemic activities. Although ADABS-Ru complex has N,O-bidendate chelating unit, the increased conjugation via phenyl groups are expected to increase the hydrophobic character. The positively charged Ru complexes have already shown to exhibit good anticancer activities since the positive charge facilitate strong interaction with negatively charged membrane that helps in the infiltration in both cancer and normal healthy cells to kill them.7,9 It is noted that positively charged P2P-Ru showed strong inhibition of both cancer as well as healthy cells, while, neutral AAPS-Rs exhibited inhibition of cancer cell growth alone (Table 3).
Table 3 IC50 value (μM) of ruthenium complexes against K562 and human PBMC. Values not sharing a common letter (a–f) are significantly different (p < 0.05)
Compound |
IC50 against K562 (μM) (mean ± SEM) |
IC50 against human PBMC (μM) (mean ± SEM) |
BPY-Ru |
65.83 ± 2.76a |
>100 |
P2P-Ru |
11.8 ± 0.49b |
28.73 ± 1.49 |
AAP-Ru |
87.93 ± 4.22c |
92.54 ± 4.72 |
AAPPA-Ru |
59.81 ± 2.53a,d |
84.68 ± 3.43 |
AAPS-Ru |
12.46 ± 0.47b,e |
>100 |
ADABS-Ru |
53.25 ± 2.59d,f |
71.95 ± 3.02 |
Hence, we have chosen the neutral AAPS-Ru arene complex for further detailed anti-leukemia activity studies in comparison with 5-FU as a control drug to elucidate the possible mechanism of action. Cell viability study on K562 and human PBMC cells were performed after AAPS-Ru treatment to evaluate the cell killing ability of the Ru-arene complex drug by exposing different concentration (0.78 μM to 100 μM) after 24 h of time period (Fig. 6a). It was observed that AAPS-Ru exhibited cytotoxicity on K562 cells from 0.78 μM onwards and 100 μM concentrations decreased the cell viability by 84.5%. Interestingly, cell viability of normal cells (PBMC) was decreased by 38% only when exposing 100 μM of AAPS-Ru. The time dependent studies of cell viability with AAPS-Ru drug suggest that exposing 12.5 μM can reduce the cell viability by 65% after 72 h (Fig. 6b). So, further studies were performed using this concentration with 24 h incubation time.
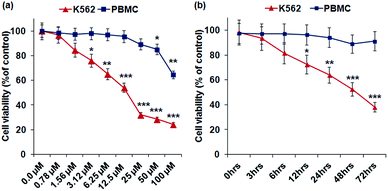 |
| Fig. 6 (a) Concentrations and (b) time dependent K562 cell viability decreased due to AAPS-Ru exposure. Data represented here as mean ± SEM from all the experimental values. *p < 0.05, **p < 0.01, and ***p < 0.001 compared with respective untreated control cells. | |
The decreased cell viability of AAPS-Ru was correlated with induction of apoptosis by studying morphology changes of K562 cells using fluorescence microscopy. The untreated and AAPS-Ru treated K562 cells and PBMCs were stained with acridine orange/ethidium bromide (AO/EB) staining. Viable cells will uptake only AO and emit green fluorescence where apoptotic cell will uptake AO/EtBr both and emit green with red/orange fluorescence. In this study, the presences of apoptosis associated nuclear morphological changes were inferred as follows: orange-red color significantly increased in AAPS-Ru treated K562 cells but not in PBMC, indicating the early and late apoptotic K562 cells. The absence of apoptotic cells in PBMC were confirmed by only green fluorescence since it uptakes only AO (Fig. 7). It is noted that 5-FU control drug treated K562 and human PBMC cells clearly show orange-red color and suggest apoptosis in both infected as well as normal cells. To study the mechanism of action, we have performed AAPS-Ru induced ROS generation experiments since ROS generation is the early events in drug-induced killing of K562 cells.32 AAPS-Ru (12.5 μM) induced ROS generation in K562 cells were measured after 60 min exposure. The results strongly indicate that AAPS-Ru selectively and significantly (p < 0.01) provoked ROS generation in K562 cells (Fig. 8a). Moreover, AAPS-Ru was more efficient than control 5-FU in ROS generation in K562 cells. Fluorescence microscopy images further supported ROS generation in K562 cells and PBMC (Fig. 8b). To confirm the critical role of AAPS-Ru-induced ROS generation in K562 cells killing, we have also performed experiments with pre-treatment of N-acetyl-L-cysteine (NAC), a selective ROS inhibitor in wide range of cells.33 It clearly indicates that, inhibition of ROS generation by NAC interfered with AAPS-Ru promoted decreased cell viability in K562 cells significantly (p < 0.05). Hence, it could be assumed that, AAPS-Ru-induced ROS generations were the critical events in K562 cells death. Most of the standard antileukemic drugs induce apoptosis passing through the ROS generation inside the leukemic cells that lead to oxidative damages of the lipid, protein and DNA molecule.32 Hence, AAPS-Ru induces ROS generation inside the K562 cells might damage the cellular building blocks and finally destroy the cells via apoptosis process.
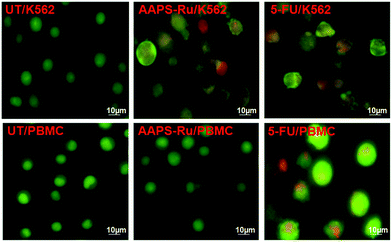 |
| Fig. 7 AAPS-Ru induced alteration in nuclear morphology of K562 cells and PBMCs by acridine orange/ethidium bromide (AO/EB) staining. Data represented hare as best photographs obtained from all experimental sets. | |
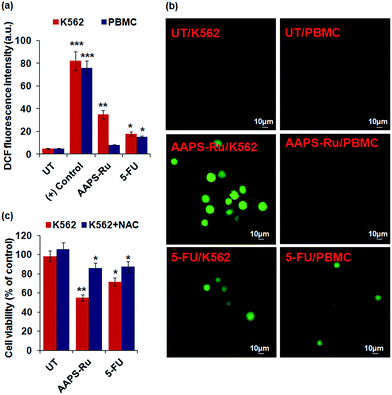 |
| Fig. 8 Induction of ROS generation is critical for the AAPS-Ru induced K562 cells killing. (a) ROS generations by K562 and PBMC were measured by spectrofluorometric method using DCFH2DA probe and bar represented here as arbitrary unit (a.u.) of fluorescence intensity of DCF. (b) Photographs of ROS generation in K562 and PBMC were taken using fluorescence microscope (Nikon) and represented hare as best photographs obtained from duplicate experimental sets obtaining similar kind of results. (c) Cell viability determination in presence of 10 mM NAC (potent ROS inhibitor). Data represented hare as mean ± SEM from all the experimental values. *p < 0.05, **p < 0.01, and ***p < 0.001 compared with respective untreated control cells. | |
LDH release, loss of cellular membrane integrity, lipid peroxidations are the major events in ROS-induced cells death.34 The LDH release and lipid peroxidation has also been evaluated in AAPS-Ru treated K562 cells and PBMC. The results showed that LDH release and MDA levels (indicator of lipid peroxidation) were significantly (p < 0.01) increased in AAPS-Ru treated K562 cells (Fig. 9a and b). This increased LDH leakages in cell culture medium of AAPS-Ru treated K562 cells were due to swift cell-membrane damage that directed to cell death and provided evidences that the membrane leakages were occurred as a consequence of the apoptosis.35 It is well known that, ROS generation induced cellular damage as well as LDH release either through apoptosis or necrosis process. Necrostatin-1 (selective inhibitor of necrosis) pre-treatment could not affect the decreased cell viability in AAPS-Ru treated K562 cells (Fig. 9c). Hence, it may be assumed that AAPS-Ru induced ROS generation activated the programmed cell death events (Apoptosis) in K562 cells. ROS mediated apoptosis is solely depended upon the cellular antioxidant and pro-oxidant status. Reduced glutathione (GSH) is a major oxidant and oxidized glutathione (GSSG) is a major pro-oxidant in cellular level, and their ratio determines the cellular damage intensity.23,25 Hence, we have measured their levels and their ratio in AAPS-Ru treated K562 cells. The results suggest that, GSH levels were significantly (p < 0.01) decreased and GSSG levels were significantly (p < 0.01) decreased in K562 cells when treated with AAPS-Ru (Fig. 9d and e). Moreover, the GSH/GSSG ratios were drastically decreased in K562 cells (Fig. 9f), indicating the cellular damage in K562 cells and corroborating with ROS generations, LDH releases and MDA levels in AAPS-Ru treated K562 cells. Tumor necrosis factor α (TNF-α) is well known to induce apoptosis/cell death in K562 cells.36 The TNF-α release in cell free supernatant from AAPS-Ru treated K562 cells and PBMC was measured using ELISA method. The results indicate strong TNF-α release associated with AAPS-Ru treatment that might induce the K562 cell killing but not in PBMC (Fig. 9a).
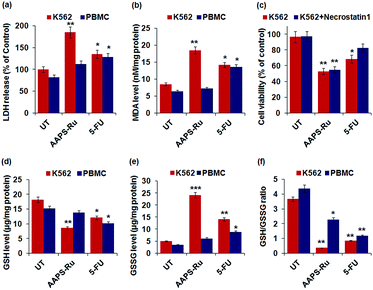 |
| Fig. 9 AAPS-Ru induced changes in (a) LDH release, (b) lipid peroxidation, (d) GSH level, (e) GSSG level, (f) GSH/GSSG ratio in K562 cells. (c) Effect of necrostatin-1 pretreatment in cell viability of AAPS-Ru treated K562 cells. All the experiments were done in duplicate and repeated at least three times. Data represented hare as mean ± SEM from all the experimental values. *p < 0.05, **p < 0.01, and ***p < 0.001 compared with respective untreated control cells. | |
To provide evidence of critical role of TNF-α, we have used pentoxifylline (POF; irreversible inhibitor of TNF-α). Pre-treatment with POF almost totally reversed back the affected cell viability during AAPS-Ru treatment (Fig. 10a). These result strongly evidenced that, AAPS-Ru selectively induced TNF-α potentiated for the cancer cell death without much affecting healthy cells, PBMC. It is well established that cancer cells secret more interleukin 10 (IL-10) for their survival through opposing the role of TNF-α and inducing immune suppression. Therefore, we have also studied the level of IL-10 in cell culture supernatant of K562 cells with or without treatment of AAPS-Ru (Fig. 10b). AAPS-Ru exposure to K562 cells decreased the IL-10 production significantly (p < 0.01) that might be responsible for inducing TNF-α mediated cell death. c-Jun-N-terminal kinase (JNK) is a member of the mitogen-activated protein (MAP) kinase family. Several reports indicated the vital role of JNK in induction of apoptosis through critical regulation of TNF-α and IL-10.28,33 AAPS-Ru-treated K562 cells death that was pre-treated with JNK inhibitor (SP600125) has also provided evidence of involving JNK signalling molecule in the apoptosis (Fig. 10c).
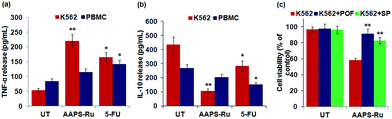 |
| Fig. 10 (a and b) AAPS-Ru increased TNF-α and decreased IL-10 to kill the K562 cells. (c) Pentoxifylline (POF) and JNK inhibitor (SP600125) increased the cell viability of AAPS-Ru treated K562 cells. Data represented hare as mean ± SEM from all the experimental values. *p < 0.05, and **p < 0.01 compared with respective untreated control cells. | |
Conclusions
In conclusion, we have synthesized and characterized new arene-Ru complexes with N-donor monodentate (AAP) and N,O- and N,N-bidentate chelating ligands (AAPS, ADABS, AAPPA and P2P) and screened for antileukemic activities. Ru(II) adopts typical “three-leg piano-stool” geometry with pseudo-tetrahedral (AAP-Ru) and quasi-octahedral arrangement (ADABS-Ru and P2P-Ru) in the coordination complex. Further, supramolecular interactions (C–H⋯Cl, N–H⋯Cl and π–π) produced dimeric structure in AAP-Ru and ADABS-Ru crystal lattice and 1D chain in P2P-Ru. The arene Ru-complexes were evaluated for in vitro anticancer activities against K562 cells, human chronic myeloid leukemia cell line, by using MTT assay. The results indicate strong anti-leukemic activity for neutral AAPS-Ru (IC50 = 12.46 ± 0.47 μM) and cationic P2P-Ru (IC50 = 11.8 ± 0.49 μM). Although cationic P2P-Ru exhibited strong anti-leukemic activities, it also kills normal healthy PBMC cells. Importantly, the neutral AAPS-Ru kills selectively chronic leukemic cells without killing healthy PBMC cells. Thus neutral complex arene-Ru complex would be more suitable for chemotherapeutic agents. The detailed mechanistic studies indicated selective ROS generation in K562 by AAPS-Ru that mediated cells death via apoptosis without affecting the normal cells. Further, the initiation of apoptosis by AAPS-Ru treatment, particularly TNF-α induced cell death, confirmed by pre-treatment with selective TNF-α inhibitor. The detailed interaction studies of Ru complexes with DNA and protein are under progress by using UV-Vis and fluorescence techniques.
Ethics statement
Human blood sample from healthy volunteers, collected by medical personal, was used to isolate the PBMC. Prior written consent was taken from the volunteers. All the experiments with PBMC was carried out in strict guideline of Institutional Biosafety Committee (IBSC) with prior approval (number: IBSC/21/2014, dated: 08.09.2014).
Acknowledgements
We are thankful to SERB, DST, Government of India (DST Fast Track scheme no. SB/FT/CS-182/2011) is acknowledged with gratitude. VM is also grateful to UGC, Government of India for UGC research award-2014-16 (No. 30-11/2015 (SA-II)). SKM is acknowledging DST-FIST programme, Govt. of India (SR/FST/ETI-331/2013) and SERB, Govt. of India (SB/YS/LS-285/2013) for instrumental and financial support.
References
-
(a) E. Alessio, Bioinorganic Medicinal Chemistry, Wiley-VCH, Weinheim, Germany, 2011 Search PubMed;
(b) A. L. Noffke, A. Habtemarian, A. M. Pizarro and P. J. Sadler, Chem. Commun., 2012, 48, 5219–5246 RSC;
(c) S. Komeda and A. Casini, Curr. Top. Med. Chem., 2012, 12, 219–223 CrossRef CAS PubMed;
(d) A. Bergamo, C. Gaiddon, J. H. M. Schellens, J. H. Beijnen and G. J. Sava, Inorg. Biochem., 2012, 106, 90–99 CrossRef CAS PubMed;
(e) A. Bergamo and G. Sava, Dalton Trans., 2011, 40, 7817–7823 RSC;
(f) M. A. Fuertes, C. Alonso and J. M. Perez, Chem. Rev., 2003, 103, 645 CrossRef CAS PubMed;
(g) D. Wang and S. J. Lippard, Nat. Rev. Drug Discovery, 2005, 4, 307 CrossRef CAS PubMed.
-
(a) K. D. Mjos and C. Orvig, Chem. Rev., 2014, 114, 4540 CrossRef CAS PubMed;
(b) N. Muhammad and Z. Guo, Curr. Opin. Chem. Biol., 2014, 19, 144 CrossRef CAS PubMed;
(c) A. A. Bekhit, O. A. El-Sayed, T. A. Al-Allaf, H. Y. Aboul-Enein, M. Kunhi, S. M. Pulicat, K. Al-Hussain, F. Al-Khodairy and J. Arif, Eur. J. Med. Chem., 2004, 39, 499 CrossRef CAS PubMed;
(d) W. M. Motswainyana, M. O. Onani and A. M. Madiehe, Polyhedron, 2012, 41, 44 CrossRef CAS;
(e) A. R. Kapdi and I. J. Fairlamb, Chem. Soc. Rev., 2014, 43, 4751 RSC;
(f) A. K. Singh, D. S. Pandey, Q. Xu and P. Braunstein, Coord. Chem. Rev., 2014, 31, 270 Search PubMed.
-
(a) C. G. Hartinger and P. J. Dyson, Chem. Soc. Rev., 2009, 38, 391 RSC;
(b) G. Gasser, I. Ott and N. J. Metzler-Nolte, Med. Chem., 2011, 54, 3 CrossRef CAS PubMed;
(c) Y. Fu, A. Habtemariam, A. M. Basri, G. J. Clarkson and P. J. Sadler, Dalton Trans., 2011, 40, 10553 RSC;
(d) A. F. A. Peacock, A. Habtemariam, R. Fernández, V. Wall, F. P. A. Fabbiani, S. Parsons, R. E. Aird, D. I. Jodrell and P. J. Sadler, J. Am. Chem. Soc., 2006, 128, 1739 CrossRef CAS PubMed;
(e) R. Schuecker, R. O. John, M. A. Jakupec, V. B. Arion and B. K. Keppler, Organometallics, 2008, 27, 6587 CrossRef CAS;
(f) I. Romero-Canelón and P. J. Sadler, Inorg. Chem., 2013, 52, 12276 CrossRef PubMed.
-
(a) A. Casini, C. G. Hartinger, A. A. Nazarov and P. J. Dyson, Top. Organomet. Chem., 2010, 32, 57 CrossRef CAS;
(b) P. J. Dyson and G. Sava, Dalton Trans., 2006, 1929 RSC;
(c) A. Peacock and P. J. Sadler, Chem.–Asian J., 2008, 3, 1890 CrossRef CAS PubMed;
(d) L. Ronconi and P. J. Sadler, Coord. Chem. Rev., 2007, 251, 1633 CrossRef CAS;
(e) C. G. Hartinger, A. D. Phillips and A. Nazarov, Curr. Top. Med. Chem., 2011, 11, 2688 CrossRef CAS PubMed;
(f) C. Mu, S. W. Chang, K. E. Prosser, A. W. Y. Leung, S. Santacruz, T. Jang, J. R. Thompson, D. T. T. Yapp, J. J. Warren, M. B. Bally, T. V. Beischlag and C. J. Walsby, Inorg. Chem., 2016, 55, 177 CrossRef CAS PubMed;
(g) G. S. Smith and B. Therrien, Dalton Trans., 2011, 40, 10793 RSC.
-
(a) G. Suss-Fink, Dalton Trans., 2010, 39, 1673 RSC;
(b) J. Reedijk, Platinum Met. Rev., 2008, 52, 2 CrossRef CAS;
(c) C. S. Allardyce and P. J. Dyson, Platinum Met. Rev., 2001, 45, 62 CAS;
(d) M. J. Clarke, Coord. Chem. Rev., 2003, 236, 209 CrossRef CAS;
(e) B. T. Loughrey, P. C. Healy, P. G. Parsons and M. L. Williams, Inorg. Chem., 2008, 47, 8589–8591 CrossRef CAS PubMed.
- H. K. Liu and P. J. Sadler, Acc. Chem. Res., 2011, 44, 349 CrossRef CAS PubMed.
-
(a) R. E. Morris, R. E. Aird, P. D. S. Murdoch, H. Chen, J. Cummings, N. D. Hughes, S. Parsons, A. Parkin, G. Boyd, D. L. Jodrell and P. J. Sadler, J. Med. Chem., 2001, 44, 3616 CrossRef CAS PubMed;
(b) O. Novakova, H. Chen, O. Vrana, A. Rodger, P. J. Sadler and V. Brabec, Biochemistry, 2003, 42, 11544 CrossRef CAS PubMed.
-
(a) A. Martínez, C. S. K. Rajapakse, R. A. Sánchez-Delgado, A. Varela-Ramirez, C. Lema and R. J. Aguilera, Inorg. Biochem., 2010, 104, 967 CrossRef PubMed;
(b) C. S. K. Rajapakse, A. Martínez, B. Naoulou, A. A. Jarzecki, L. Suárez, C. Deregnaucourt, V. Sinou, J. Schrével, E. Musi, G. Ambrosini, G. K. Schwartz and R. A. Sánchez-Delgado, Inorg. Chem., 2009, 48, 1122 CrossRef CAS PubMed.
-
(a) F. Caruso, M. Rossi, A. Benson, C. Opazo, D. Freedman, E. Monti, M. B. Gariboldi, J. Shaulky, F. Marchetti, R. Pettinari and C. J. Pettinari, Med. Chem., 2012, 55, 1072 CrossRef CAS PubMed;
(b) R. Pettinari, F. Marchetti, F. Condello, C. Pettinari, G. Lupidi, R. Scopelliti, S. Mukhopadhyay, T. Riedel and P. J. Dyson, Organometallics, 2014, 33, 3709 CrossRef CAS;
(c) S. Banerjee and A. R. Chakravarty, Acc. Chem. Res., 2015, 48, 2075 CrossRef CAS PubMed.
- C. H. Wu, D. H. Wu, X. Liu, G. Guoyiqibayi, D. D. Guo, G. Lv, X. M. Wang, H. Yan, H. Jiang and Z. H. Lu, Inorg. Chem., 2009, 48, 2352 CrossRef CAS PubMed.
-
(a) C. Scolaro, A. Bergamo, L. Brescacin, R. Delfino, M. Cocchietto, G. Laurenczy, T. J. Geldbach, G. Sava and P. J. Dyson, J. Med. Chem., 2005, 48, 4161 CrossRef CAS PubMed;
(b) A. Weiss, R. H. Berndsen, M. Dubois, C. Mueller, R. Schibli, A. W. Griffioen, P. J. Dyson and P. Nowak-Sliwinska, Chem. Sci., 2014, 5, 4742 RSC.
- A. Bergamo, A. Masi, A. F. A. Peacock, A. Habtemariam, P. J. Sadler and G. J. Sava, Inorg. Biochem., 2010, 104, 79–86 CrossRef CAS PubMed.
-
(a) A. Bergamo, C. Gaiddon, J. H. M. Schellens, J. H. Beijnen and G. Sava, J. Inorg. Biochem., 2012, 106, 90–99 CrossRef CAS PubMed;
(b) G. Sava, E. Alessio, A. Bergamo and G. Mestroni, Topics in Biological Inorganic Chemistry, ed. M. J. Clarke and P. J. Sadler, Springer-Verlag, Berlin, 1999, vol. 1, p. 143 Search PubMed;
(c) J. M. Rademaker-Lakhai, D. Van Den Bongard, D. Pluim, J. H. Beijnen and J. Schellens, Clin. Cancer Res., 2004, 10, 3717–3727 CrossRef CAS PubMed;
(d) E. Alessio, G. Mestroni, A. Bergamo and G. Sava, Curr. Top. Med. Chem., 2004, 4, 1525–1535 CrossRef CAS PubMed.
- C. G. Hartinger, S. Zorbas-Seifried, M. A. Jakupec, B. Kynast, H. Zorbas and B. K. Keppler, J. Inorg. Biochem., 2006, 100, 891–904 CrossRef CAS PubMed.
-
(a) R. Trondl, P. Heffeter, C. R. Kowol, M. A. Jakupec, W. Berger and B. K. Keppler, Chem. Sci., 2014, 5, 2925–2932 RSC;
(b) P. Heffeter, B. Atil, K. Kryeziu, D. Groza, G. Koellensperger, W. Koerner, U. Jungwirth, T. Mohr, B. K. Keppler and W. Berger, Eur. J. Cancer, 2013, 49, 3366–3375 CrossRef CAS PubMed.
- E. A. Hillard, A. Vesseires and G. Jaouen, Top. Organomet. Chem., 2010, 32, 81–117 CrossRef CAS.
- W. E. Hunt, C. H. Schwalbe, K. Bird and P. D. Mallinson, Biochemical, 1980, 187, 533 CrossRef CAS.
-
(a) G. Turan-Zitonni, M. Sivaci, F. S. Kilic and K. Erol, Eur. J. Med. Chem., 2007, 36, 685 CrossRef;
(b) A. Pastrakuljic, R. N. Patel, S. Kumar and K. B. Pandeya, J. Inorg. Biochem., 2002, 89, 61 CrossRef.
- V. Jaiswal, R. B. Rastogi and R. J. Kumar, J. Mater. Chem. A, 2014, 2, 10424–10434 CAS.
- C.-G. Niu, A.-L. Guan, G.-M. Zeng, Y.-G. Liu and Z.-W. Li, Anal. Chim. Acta, 2006, 577, 264–270 CrossRef CAS PubMed.
- Y. F. Liu, C.-L. Wang, W. J. Bai, N. Han, J.-P. Jiao and X. L. Qi, Org. Process Res. Dev., 2008, 12, 490–495 CrossRef CAS.
- K. Gupta, D. Tyagi, A. D. Dwivedi, S. M. Mobin and S. K. Singhm, Green Chem., 2015, 17, 4618–4627 RSC.
- S. K. Dash, S. S. Dash, S. Chattopadhyay, T. Ghosh, S. Tripathy, S. Kar Mahapatra, B. G. Bag, D. Das and S. Roy, RSC Adv., 2015, 5, 24144–24157 RSC.
- L. Hudson and F. C. Hay, in Practical Immunology, Blackwell Publishing, Oxford, UK, 3rd edn, 1989 Search PubMed.
- S. Kar Mahapatra, S. P. Chakraborty, S. Majumdar, B. G. Bag and S. Roy, Eur. J. Pharmacol., 2009, 623, 132–140 CrossRef CAS PubMed.
- O. H. Lowry, N. J. Rosebrough, A. L. Farr and R. J. Randall, J. Biol. Chem., 1951, 193, 265–275 CAS.
- R. Gaur, R. A. Khan, S. Tabassum, P. Shah, M. L. Siddiqi and L. J. Mishra, J. Photochem. Photobiol., A, 2011, 220, 145–152 CrossRef CAS.
- C. M. Clavel, E. Paunescu, P. Nowak-Sliwinska and P. J. Dyson, Chem. Sci., 2014, 5, 1097–1101 RSC.
-
(a) C. M. Clavel, E. Păunescu, P. Nowak-Sliwinska, A. W. Griffioen, R. Scopelliti and P. J. Dyson, J. Med. Chem., 2014, 57, 3546–3558 CrossRef CAS PubMed;
(b) K. J. Kilpin and P. J. Dyson, Chem. Sci., 2013, 4, 1410–1419 RSC.
- I. N. Stepanenko, M. S. Novak, G. Muhlgassner, A. Roller, M. Hejl, V. B. Arion, M. A. Jakupec and B. K. Keppler, Inorg. Chem., 2011, 50, 11715–11728 CrossRef CAS PubMed.
-
(a) L. Glans, A. Ehnbom, C. de Kock, A. Martínez, J. strada, P. J. Smith, M. Haukka, R. A. Sánchez-Delgado and E. Nordlander, Dalton Trans., 2012, 41, 2764–2773 RSC;
(b) E. Ekengard, L. Glans, I. Cassells, T. Fogeron, P. Govender, T. Stringer, P. Chellan, G. C. Lisensky, W. H. Hersh, I. Doverbratt, S. Lidin, C. de Kock, P. J. Smith, G. S. Smith and E. Nordlander, Dalton Trans., 2015, 44, 19314–19329 RSC.
- A. Ganguly, B. Das, A. Roy, N. Sen, S. B. Dasgupta, S. Mukhopadhayay and H. K. Majumder, Cancer Res., 2007, 67, 11848–11858 CrossRef CAS PubMed.
-
(a) K. B. A. Ahmed, S. Kar Mahapatra, M. R. Charan Raja, S. Subramaniam, M. Sengan, N. Rajendran, S. K. Das, K. Haldar, S. Roy, S. Sivasubramanian and V. Anbazhagan, RSC Adv., 2016, 6, 18980–18989 RSC;
(b) S. Kar Mahapatra, S. P. Chakraborty, S. Majumdar, B. G. Bag and S. Roy, Eur. J. Pharmacol., 2009, 623, 132–140 CrossRef CAS PubMed.
- M. J. Chow, C. Licona, G. Pastorin, G. Mellitzer, W. H. Ang and C. Gaiddon, Chem. Sci., 2016, 7, 4117–4124 RSC.
- S. Gurunathan, J. Raman, S. N. A. Malek, P. A. John and S. Vikineswary, Int. J. Nanomed., 2013, 8, 4399–4413 Search PubMed.
-
(a) L. Wang, F. Du and X. Wang, Cell, 2008, 133, 693 CrossRef CAS PubMed;
(b) S. Chattopadhyay, S. K. Das, S. Kar Mahapatra, S. Tripathy, T. Ghosh, B. Das, D. Das, P. Pramanik and S. Roy, J. Biol. Inorg. Chem., 2014, 19, 399–414 CrossRef CAS PubMed.
Footnote |
† Electronic supplementary information (ESI) available: Synthesis, 1H and 13C NMR spectra, IR spectra, mass spectra and tables of complete bond lengths and angles for compounds AAP-Ru, ADABS-Ru and P2P-Ru. CCDC 1476964–1476966. For ESI and crystallographic data in CIF or other electronic format see DOI: 10.1039/c6ra18504e |
|
This journal is © The Royal Society of Chemistry 2016 |