DOI:
10.1039/C6RA17934G
(Paper)
RSC Adv., 2016,
6, 87828-87835
Poly(propylene)-grafted thermally reduced graphene oxide and its compatibilization effect on poly(propylene)–graphene nanocomposites†
Received
14th July 2016
, Accepted 9th September 2016
First published on 9th September 2016
Abstract
Graphene is a nanofiller with outstanding mechanical, electrical, and gas barrier properties. However, graphene nanofillers suffer from poor dispersibility in polymer matrices, particularly in non-polar polymers. This paper reports the preparation and characterization of enhanced poly(propylene)/graphene nanoplatelet (PP)/(GNP) nanocomposites, using PP-grafted thermally reduced graphene oxide (TRGO) as a compatibilizer. We optimized the synthesis of the compatibilizers by controlling the temperature of the reduction of graphene oxide. Reduction reactions were carried out at 200 °C (TRGO2), 400 °C (TRGO4) and 600 °C (TRGO6). The crystallinity, crystallization temperature, and onset temperature of PP/GNP nanocomposites were all increased by the presence of the PP-g-TRGO compatibilizers in loadings of 0.1 wt%. The mechanical and gas barrier properties of PP/GNP nanocomposites were enhanced by the presence of only 0.1 wt% PP-g-TRGO compatibilizer. Among the three PP-g-TRGO compatibilizers used to prepare PP/GNP nanocomposites (PP-g-TRGO2, PP-g-TRGO4 and PP-g-TRGO6), those prepared using PP-g-TRGO6 exhibited superior electrical, mechanical and oxygen barrier properties. These results were attributed to high crystallinity, a strong interfacial interaction between GNPs and the compatibilized PP matrix, and the compatibilizer-enhanced dispersibility of the GNPs in the PP matrix. The superior performance of PP-g-TRGO6 in particular is suggested to occur because of the greater availability of strong π–π interactions between GNPs and the increased sp2-hybridised carbon content of TRGO6, relative to the other TRGO systems. The performance-enhancing effect of PP–TRGO6 was evident despite its lower TRGO content, compared to PP-g-TRGO2 and PP-g-TRGO4.
Introduction
Poly(propylene) (PP) is a representative non-polar polymer that is widely used in food packaging, medical parts, household products, toys, and the construction industry. Its widespread use reflects its low price, outstanding processability, and desirable mechanical properties, such as tensile strength, stiffness, and excellent resistance against chemical corrosion.1 However, for applications in advanced sectors such as automotive and electronic parts, PP's physical, chemical, and electrical properties need to be improved by the addition of various fillers. Carbon materials, such as carbon black,2–6 carbon fiber,7–11 carbon nanotubes (CNTs),12–15 graphene and graphene oxide16–34 have been studied as fillers to enhance the performance of PP nanocomposites.
Graphene, which was first obtained by Geim and Novoselov in 2004,35 is considered an excellent material in various applications, because of its outstanding properties and two-dimensional structure. Graphene-based polymer composites have attracted attention among the various applications of graphene because of their extraordinary electrical, mechanical, thermal, and gas barrier properties, which can be achieved using very low graphene loadings. However, the performance of graphene-based polymer composites is limited by the problem of graphene's low dispersibility in polymer matrices, and particularly in non-polar polymers. This low dispersibility occurs, because graphene tends to aggregate due to its strong π–π and van der Waals interactions.36
To overcome this problem, graphene oxide is commonly used as a starting material from which graphene nanosheets are produced. While graphene oxide or graphene nanosheets can be dispersed well in polar polymers such as poly(methyl methacrylate) (PMMA), poly(acrylonitrile) (PAN), poly(acrylic acid) (PAA), poly(ester), epoxy resin, thermoplastic poly(urethane) (TPU), poly(vinyl alcohol) (PVA), poly(aniline), and poly(3-hexylthiophene),37–57 its dispersion in non-polar polymer such as PP and poly(ethylene) (PE) matrices remains challenging. This is because these polymers lack any strong interfacial interactions with graphene. For this reason, much research is now focused on the creation of high-performance graphene dispersions in non-polar polymer matrices.
Some recent studies report the successful preparation of modified graphene dispersions in PP22–34 or PE58–61 matrix. A graphene oxide starting material was used, for its advantageous oxygen-containing functional groups, which aid dispersion. Yun et al.23 prepared alkylated GO by an SN2 reaction between dodecyl iodide and the oxygen-containing groups of GO, followed by solution blending with PP to obtain an alkylated GO/PP nanocomposite. They reported that, by improving the dispersion of GO in the PP matrix, the thermal stability and mechanical properties were improved. Zhang et al.27 prepared PP-grafted GO by reaction between isocyanate-grafted PP and the hydroxyl and carboxylic groups of GO. They reported that the crystallization rate of PP-g-GO was accelerated compared to that for PP/natural graphite composites. Nevertheless, sufficiently enhancing the mechanical, electrical, and gas barrier properties of PP by forming PP/graphene nanocomposites presents an ongoing and challenging problem.
Here, we introduce PP-graft-thermally reduced graphene oxide (TRGO) as a compatibilizer, to obtain high performance PP/graphene nanoplatelets (GNP) nanocomposites, with PP matrices that are free from problematic agglomerations of graphene. We control the degree of reduction of TRGO, using the reduction temperature, to optimize production of an appropriate compatibilizer. The remaining hydroxyl and carboxylic groups of TRGO were functionalized with isocyanate-grafted PP (PP-g-m-TMI). PP-g-m-TMI was synthesized via free-radical grafting of 3-isopropenyl-α,α-dimethylbenzyl isocyanate (m-TMI) onto PP by reactive extrusion.62 PP/GNP nanocomposites with low graphene loading were achieved using this PP-g-TRGO compatibilizer, and showed optimal electrical properties for application in electrostatic discharge (ESD) as well as outstanding mechanical and barrier properties. The crystallization behavior of PP/graphene nanocomposites that were compatibilized with PP-g-TRGO was also studied, to explain the observed improvements in electrical, mechanical, and gas barrier properties.
Experimental
Materials
Poly(propylene) (melt flow index 4.0 g/10 min at 230 °C, 2.16 kg load, ASTM D1238) was provided by LOTTE Chemical Co. Ltd., Korea. 3-Isopropenyl-α,α-dimethylbenzyl isocyanate (m-TMI), molecular weight 201.27 g mol−1, was provided by TCI Co. Ltd., Japan. Dicumyl peroxide (DCP) was used as the free radical initiator for m-TMI grafting of PP. Graphene oxide nanoplatelets (GO; N002-PDE, XY < 7 μm, Z ∼ 3 nm) and graphene nanoplatelets (GNP; N002-PDR, XY < 10 μm, Z ∼ 1 nm) were provided by Angstron Materials.
Preparation of TRGO
The experimental scheme for production of TRGO, PP-g-TRGO, and PP/PP-g-TRGO/GNP composites is presented in Scheme 1. The GO was thermally reduced in a Thermolyne 21100 tube furnace: 50 mg of GO was introduced to the furnace and heated at 5 °C min−1 under an N2 atmosphere to 200 °C, 400 °C, and 600 °C. Each sample was held at its respective temperature for 30 min.
 |
| Scheme 1 Experimental schemes for the preparation of PP/PP-g-TRGO/GNP. | |
Synthesis of PP-g-m-TMI
PP-g-m-TMI was synthesized by a melt radical grafting reaction. m-TMI (4 g) was added to PP (40 g), then dicumyl peroxide (4 g) was added to the mixture. The mixture was reacted in the internal mixer of a torque rheometer (Plasti-corder, Brabender) at 180 °C and 80 rpm for 6 min. The reaction mixture was dissolved in refluxing xylene and hot-filtered, and then the filtered liquor was washed with acetone several times. The precipitated polymer was dried in vacuum oven at 80 °C for 12 h.
Synthesis of PP-g-TRGO2, PP-g-TRGO4, and PP-g-TRGO6
TRGO2 (0.2 g) was suspended in xylene (10 mL) and sonicated for 1 h in an ultrasonic bath (BRANSON 8200). PP-g-m-TMI (1.6 g) was added to a three-necked flask with xylene (50 mL), and the mixture was stirred. The TRGO2 suspension was added to the mixture in the flask, followed by refluxing for 8 h under an N2 atmosphere. The reaction mixture was hot-filtered and the filtered liquor was washed with acetone several times. The precipitated polymer was dried in vacuum oven at 80 °C for 12 h. PP-g-TRGO4 and PP-g-TRGO6 were synthesized using the same method as reported above.
Preparation of PP/PP-g-TRGO2/GNP, PP/PP-g-TRGO4/GNP, and PP/PP-g-TRGO6/GNP nanocomposites
Each PP/PP-g-TRGO/GNP nanocomposite was prepared by melt-mixing (Plasti-corder, Brabender) the initial materials at 180 °C and 80 rpm for 5 min. For each TRGO sample, three PP/PP-g-TRGO/GNP mixing ratios were used: 40/0.04/0.4 g, 40/0.04/0.56 g, and 40/0.04/0.8 g.
Instrumentation
TRGO, PP-g-m-TMI, and PP-g-TRGO were each investigated by Fourier transform infrared spectroscopy, (FT-IR, Thermo Scientific NICOLET iN10MX). The structure of TRGO samples was verified by X-ray photoelectron spectroscopy (XPS), using an AXIS – NOVA (Kratos Inc.) with an Al Kα radiation source at room temperature and maintained under vacuum at 2.0 × 10−9 Torr. 1H NMR was used to characterize PP-g-m-TMI samples, (Agilent DD2 500 MHz) with 1,1,2,2-tetrachloroethane-d2 as solvent. Thermal gravimetric analysis (TGA) was performed on a Q500 (TA instruments) with a heating rate of 10 °C min−1 from 30 °C to 600 °C, under a nitrogen atmosphere. The crystallization behavior of PP-g-TRGO and its nanocomposites was investigated by differential scanning calorimetry (DSC, Q200 TA instruments) under a nitrogen atmosphere. The non-isothermal crystalline melting behavior of the specimens was obtained by heating from 30 °C to 200 °C at a heating rate of 10 °C min−1, followed by cooling to 30 °C at a cooling rate of 10 °C min−1. The electrical properties of PP-g-TRGO and nanocomposites were investigated using a high resistance meter (Advantest R8340 Ultra High Resistance meter, with volume resistivity from 1 × 1010 to 3 × 1016, and a HIRESTA-UP MCP-HT450 from Mitsubishi Chemicals, with volume resistivity from 1 × 104 to 1 × 1013). The dispersion of graphene in the PP matrix was observed by scanning electron microscopy (SEM), using an SU8220 Hitachi scanning electron microscope at 20 kV. The samples were fractured in liquid nitrogen, and then coated with a layer of gold. The tensile properties were tested using an Instron 5566 Ultimate Tensile Testing machine (UTM). Dumb-bell shaped specimens were prepared according to the ASTM D 638 standard. The crosshead speed was 50 mm min−1. The O2 barrier properties of nanocomposites were measured using an OX-TRAN 2/21(MOCON) testing machine at room temperature with 2.4% relative humidity. Sheet specimens were prepared with dimensions of 50 cm2 and thicknesses of 0.95 mm. The conditioning time used was 1 h, and testing time was 22 h.
Results and discussion
Preparation and characterization of TRGO2, TRGO4, and TRGO6
The structure of GO, TRGO2, TRGO4, and TRGO6 samples was investigated using FT-IR spectroscopy, shown in Fig. 1. The GO samples show bands corresponding to C
O vibrations of carbonyl and carboxylic acid groups (1728 cm−1), C
C vibrations (1626 cm−1), epoxy C–O stretching (1369 cm−1), O–H deformation (1222 cm−1) and alkoxy C–O stretching (1050 cm−1) were detected. TRGO2, TRGO4, and TRGO6 peaks, corresponding to C
O vibrations of carbonyl and carboxylic acid (1734–1736 cm−1), C
C skeleton vibrations (1582 cm−1), epoxy C–O stretching (1356 cm−1), and alkoxy C–O stretching (1242 cm−1) were also present. Reduction of GO in TRGO2, TRGO4, and TRGO6, was indicated by the reduced intensity of the C
O vibrations compared with the C
C peak, and the removal of absorbance bands corresponding to C–O and O–H, which were not detected. TRGO6 was the most reduced sample, indicated by the very low C
O vibration intensity. XPS analysis was used to further confirm the reduction of GO had occurred. XPS spectra of GO, TRGO2, TRGO4, and TRGO6 are shown in Fig. 2, including the survey spectrum (Fig. 2a) and the detailed C 1s spectrum (Fig. 2b). The binding energy of the C 1s peak at 284.8 eV was assigned to the C–C group, which showed much higher intensity for TRGO2, TRGO4, and TRGO6 than for GO. Increasing the temperature at which GO was reduced led to increased peak intensities, with TRGO6 > TRGO4 > TRGO2. The atomic C/O ratios, shown in Fig. 2c, were calculated to be C
:
O = 2.20
:
1, 5.18
:
1, 5.94
:
1 and 9.11
:
1, for GO, TRGO2, TRGO4, and TRGO6, respectively. These data confirm the successful thermal reduction of GO by varying the reduction rate.
 |
| Fig. 1 FT-IR spectra of GO, TRGO2, TRGO4, and TRGO6. | |
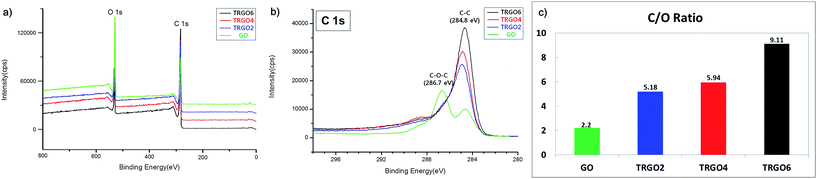 |
| Fig. 2 XPS analysis of GO and TRGO2, TRGO4, and TRGO6. (a) The survey spectra (b) C 1s peaks (c) C/O ratio. | |
Preparation and characterization of PP-g-m-TMI
Fig. 3 shows the FT-IR spectra of PP, m-TMI, and PP-g-m-TMI. PP-g-m-TMI shows a characteristic absorbance at 2260 cm−1, corresponding to the isocyanate group of TMI. The lack of a peak at 1690 cm−1, which corresponds to the vinyl group of m-TMI, further indicates grafting of m-TMI to PP. 1H NMR (Fig. S1†) also confirmed the production of PP-g-m-TMI, as no signal was observed corresponding to the vinyl group of m-TMI, which typically appears at 4.8 ppm.
 |
| Fig. 3 FT-IR spectra of PP (red), m-TMI (purple), and PP-g-m-TMI (blue). | |
Preparation and characterization of PP-g-TRGO2, PP-g-TRGO4, and PP-g-TRGO6
The FT-IR spectra of GO, PP-g-m-TMI, PP-g-TRGO2, PP-g-TRGO4, and PP-g-TRGO6 are shown in Fig. 4. PP-g-TRGO2, PP-g-TRGO4 and PP-g-TRGO6 show peaks corresponding to C
O vibrations of carbonyl and carboxylic acid groups (1726 cm−1), and C
C framework vibrations of GO (1582 cm−1). The intensity of the isocyanate (CNO) absorbance of PP-g-TRGO2, PP-g-TRGO4, and PP-g-TRGO6 (2255 cm−1) appears to be decreased with respect to the isocyanate absorbance of PP-g-m-TMI (2251 cm−1), suggesting that the isocyanate of PP-g-m-TMI has formed bonds to the –OH and epoxide groups of TRGO. These results confirm covalent bonding between the PP polymer chains of PP-g-m-TMI and the surface of TRGO, although amide linkages were not detected because of the overlap with the hydroxyl and vinyl groups of TRGO. TGA was used to ascertain the TRGO content of PP-g-TRGO, indirectly. The TGA analysis of PP-g-m-TMI, PP-g-TRGO2, PP-g-TRGO4, and PP-g-TRGO6 are shown in Fig. 5. The amount of residue remaining after thermal degradation at 600 °C for PP-g-m-TMI, PP-g-TRGO2, PP-g-TRGO4, and PP-g-TRGO6, was 0.1, 2.3, 1.5, and 1.2 wt%, respectively. The TGA results show that TRGO2 was incorporated into the PP-g-TRGO materials in the largest amount, and TRGO6 was least incorporated. This is congruent with TRGO2 possessing the most oxygen functionality, while few oxygen-containing groups remained in the more highly reduced TRGO6. Thus, TRGO2 was able to react to a greater extent with the isocyanate groups of PP-g-m-TMI, and the overall TRGO loading could therefore be controlled by varying the thermal reduction temperature of GO. The non-isothermal crystallinity of PP-g-m-TMI, PP-g-TRGO2, PP-g-TRGO4, and PP-g-TRGO6, obtained by DSC, are shown in Table 1. The crystallization temperature (Tc) and onset temperature (Ton) of PP-g-TRGO were slightly increased with respect to PP-g-m-TMI, but the differences between these species were minor. Fig. 6 shows the electrical properties of PP-g-m-TMI, PP-g-TRGO2, PP-g-TRGO4, and PP-g-TRGO6. The lowest value of volume resistivity among all the samples was measured for PP-g-TRGO6, with 5.0 × 107 Ω cm. This suggests that PP-g-TRGO6 has the best electrical properties, afforded by the high proportion of sp2-hybridized carbon atoms resulting from the high degree of reduction of TRGO in this sample. This superior performance occurs despite a lower TRGO content for PP-g-TRGO6 than for PP-g-TRGO2 and PP-g-TRGO4.
 |
| Fig. 4 FT-IR spectra of GO, PP-g-m-TMI, PP-g-TRGO2, PP-g-TRGO4, and PP-g-TRGO6. | |
 |
| Fig. 5 TGA analysis of PP-g-m-TMI, PP-g-TRGO2, PP-g-TRGO4, and PP-g-TRGO6. | |
Table 1 Crystallization behavior of PP-g-m-TMI, PP-g-TRGO2, PP-g-TRGO4, and PP-g-TRGO6
Sample |
Tc (°C) |
ΔHc (J g−1) |
Ton (°C) |
PP-g-m-TMI |
107.9 |
77.3 |
110.7 |
PP-g-TRGO2 |
105.6 |
71.3 |
110.8 |
PP-g-TRGO4 |
106.6 |
73.6 |
110.9 |
PP-g-TRGO6 |
106.6 |
76.8 |
110.9 |
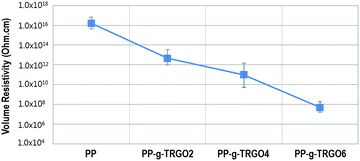 |
| Fig. 6 Volume resistivity of PP, PP-g-TRGO2, PP-g-TRGO4, and PP-g-TRGO6 nanocomposites. | |
Characterization of PP/PP-g-TRGO2/GNP, PP/PP-g-TRGO4/GNP, and PP/PP-g-TRGO6/GNP nanocomposites
In order to demonstrate the compatibilization effect of PP-g-TRGO, nanocomposites of PP/GNPs were made using PP-g-TRGO to aid the dispersion of GNPs in the PP matrix. Nanocomposites were made with GNP loadings of 1.0 wt% and PP-g-TRGO2, PP-g-TRGO4 and PP-g-TRGO6 loadings of 0.1 wt% each. Fig. S2† shows the XRD pattern of PP, PP/GNP, PP/PP-g-TRGO2/GNP, PP/PP-g-TRGO4/GNP, and PP/PP-g-TRGO6/GNP nanocomposites. No change can be found around 2θ = 26.5° (representing the graphitic structure) in the diffraction profiles of nanocomposites. PP/GNP nanocomposites with low loading of GNP cannot be found the restacking of GNP in the matrix by XRD easily. SEM was used to examine the morphology of the GNPs dispersed within the PP matrix. Cryogenically fractured surfaces of the PP/PP-g-TRGO/GNP nanocomposites were imaged, as shown in Fig. 7. When no PP-g-TRGO was present, GNPs were strongly aggregated in the PP matrix, and large agglomerations (red circle, Fig. 7a) could be seen. However, when PP-g-TRGO was present (Fig. 7b–d), the agglomerate sizes were dramatically reduced. This improvement in the morphology of the nanocomposites is attributed to the improved dispersion of GNPs in the PP matrix, afforded by the presence of the PP-g-TRGO2, PP-g-TRGO4, and PP-g-TRGO6 compatibilizers.
 |
| Fig. 7 SEM image of (a) PP/GNP, (b) PP/PP-g-TRGO2/GNP, (c) PP/PP-g-TRGO4/GNP, and (d) PP/PP-g-TRGO6 nanocomposites with GNP (1.0 wt%) and PP-g-TRGO2/4/6 (0.1 wt%). | |
Crystallization behavior of PP/PP-g-TRGO2/GNP, PP/PP-g-TRGO4/GNP, and PP/PP-g-TRGO6/GNP nanocomposites
DSC was used to investigate the non-isothermal crystallization behavior of PP, PP/GNP, PP/PP-g-TRGO2/GNP, PP/PP-g-TRGO4/GNP, and PP/PP-g-TRGO6/GNP nanocomposites, prepared with GNP loadings of 1.0 wt% and PP-g-TRGO2/4/6 content of 0.1 wt% each. Exothermal heat flow curves, measured during the non-isothermal crystallization, are shown in Fig. 8. A cooling rate of 10 °C min−1 was used The observed crystallization temperature (Tc), onset temperature (Ton), crystallization enthalpy (ΔHc) and degree of crystallinity (Xc) are summarized in Table 2. The crystallinity of nanocomposites was determined using eqn (1). |
 | (1) |
where ΔHm is melting enthalpy, fPP is weight fraction of PP and ΔH0 is the heat of fusion of 100% crystalline PP, which is reported to be 209 J g−1.63 An increase of 11 °C was observed in the Tc of the PP/GNP nanocomposite, as compared with the PP homopolymer. This is suggested to be because of the nucleation effect of graphene in the PP matrix. Ton and Tc of PP/PP-g-TRGO/GNP nanocomposites were slightly increased compared to the PP/GNP nanocomposite that lacked any compatibilizing agent. Furthermore, the crystallinity of PP/PP-g-TRGO/GNP nanocomposites was 1.6%, 1.7% and 2.5% higher for PP-g-TRGO2, PP-g-TRGO4 and PP-g-TRGO6, respectively, than for PP/GNP alone. The increases in Ton and Xc of PP-g-TRGO-compatibilized nanocomposites is attributed to improved dispersion of graphene in the PP matrix, leading to an amplification of the nucleating effect caused by π–π interactions between graphene and TRGO. PP/PP-g-TRGO6/GNP showed the highest crystallinity and Ton, due to its increased proportion of sp2-hybridised carbon from its greater reduction degree. This effect occurs even though the graphene content of TRGO6 is lower than in the other TRGO-containing nanocomposites.
 |
| Fig. 8 Crystallization behavior of PP, PP/GNP, PP/PP-g-TRGO2/GNP, PP/PP-g-TRGO4/GNP and PP/PP-g-TRGO6 nanocomposites with GNP (1.0 wt%) and PP-g-TRGO2/4/6 (0.1 wt%). | |
Table 2 Crystallization behavior of PP, PP/GNP, PP/PP-g-TRGO2/GNP, PP/PP-g-TRGO4/GNP and PP/PP-g-TRGO6 nanocomposites with GNP (1.0 wt%) and PP-g-TRGO2/4/6 (0.1 wt%)
Sample |
Tc (°C) |
ΔHc (J g−1) |
Ton (°C) |
Xc (%) |
PP |
113.2 |
101.5 |
119.8 |
49.1 |
PP/GNP (1%) |
124.2 |
103.8 |
127.7 |
50.9 |
PP/PP-g-TRGO2 (0.1%)/GNP (1.0%) |
124.7 |
105.7 |
128.5 |
51.7 |
PP/PP-g-TRGO4 (0.1%)/GNP (1.0%) |
124.7 |
105.8 |
128.5 |
51.8 |
PP/PP-g-TRGO6 (0.1%)/GNP (1.0%) |
124.7 |
107.4 |
128.8 |
52.2 |
Mechanical properties of PP/PP-g-TRGO2/GNP, PP/PP-g-TRGO4/GNP, and PP/PP-g-TRGO6/GNP nanocomposites
The mechanical properties of PP/GNP nanocomposites with various GNP loadings were investigated. Nanocomposites were made either with PP-g-TRGO loadings of 0.1 wt%, or without any PP-g-TRGO compatibilization, and the results of tensile strength and Young's modulus investigations of these materials are shown in Fig. 9a and b, respectively. The tensile strength and Young's modulus of PP/GNP nanocomposites with a GNP loading of 1.0 wt% were enhanced, compared to PP alone. However, further increasing the GNP loading of the PP/GNP nanocomposites, to 1.4 and 2.0 wt% GNP, caused a reduction in the tensile strength and Young's modulus. Song et al.22 attributed this behavior to the agglomeration of graphene at higher graphene loading. However, all compatibilized PP/PP-g-TRGO/GNP nanocomposites showed greater tensile strength and Young's modulus than PP/GNP nanocomposites that lacked PP-g-TRGO compatibilization. With GNP loading of 1 wt%, the tensile strength of the PP/PP-g-TRGO2/GNP, PP/PP-g-TRGO4/GNP and PP/PP-g-TRGO6/GNP nanocomposites was 2%, 4% and 11% greater, respectively, than PP/GNP nanocomposites. The Young's modulus of PP/PP-g-TRGO2/GNP, PP/PP-g-TRGO4/GNP, and PP/PP-g-TRGO6/GNP nanocomposites was 7%, 21%, and 25% higher than PP/GNP nanocomposites, respectively. These enhancements in tensile strength and Young's modulus are not only dependent on graphene being well dispersed in the PP matrix and high crystallinity of the nanocomposites, but also on strong interfacial interactions between PP and the graphene filler.13,18,32 Among the three TRGO compatibilizers, PP/PP-g-TRGO6/GNP nanocomposites exhibit particularly significant enhancements. The high degree of graphene reduction in this sample leads to strong interfacial interactions, through increased π–π interactions, between PP–TRGO and the GNPs. We therefore attribute the observed enhancements in performance to these strong interactions, combined with the high crystallinity and highly dispersed graphene of the PP/PP-g-TRGO6/GNP nanocomposites.
 |
| Fig. 9 Tensile strength and Young's modulus of PP, PP/GNP, PP/PP-g-TRGO2/GNP, PP/PP-g-TRGO4/GNP, and PP/PP-g-TRGO6/GNP nanocomposites with GNP (1.0 wt%, 1.4 wt%, 2.0 wt%) and PP-g-TRGO2, 4, 6 (0.1 wt%). | |
Electrical properties of PP/PP-g-TRGO2/GNP, PP/PP-g-TRGO4/GNP, and PP/PP-g-TRGO6/GNP nanocomposites
The volume resistivity of the PP/GNP nanocomposites, shown in Fig. 10, was measured to determine their electrical properties. Nanocomposites of PP/GNP with a GNP loading of 1.4 wt% and a PP–TRGO(2, 4, or 6) compatibilizer content of either 0.1 wt% or 0 wt%, were investigated. The volume resistivity of PP alone was 1.6 × 1016 Ω cm, consistent with an insulator. The electrical threshold value of PP/GNP nanocomposites was 6.3 × 109 Ω cm for a 1.4 wt% GNP loading, introduced by a melt mixing method (Fig. S3†). The volume resistivity of PP/GNP nanocomposites increased successively for PP-g-TRGO2, PP-g-TRGO4, and PP-g-TRGO6 despite only being present in amounts of 0.1 wt%. The PP/PP-g-TRGO6/GNP nanocomposites exhibited the lowest volume resistivity of the compatibilizer-containing samples, with a value of 2.5 × 108 Ω cm. This resistivity enhancement was attributed to improved dispersion of graphene in the PP matrix by PP-g-TRGO6, as well as the higher conductivity of PP-g-TRGO6 compared with PP-g-TRGO2 and PP-g-TRGO4, because of its high sp2 hybridized carbon content.
 |
| Fig. 10 Volume resistivity of PP/GNP, PP/PP-g-TRGO2/GNP, PP/PP-g-TRGO4/GNP, and PP/PP-g-TRGO6/GNP nanocomposites with GNP (1.4 wt%) and PP-g-TRGO2, 4, 6 (0.1 wt%). | |
Barrier properties of PP/PP-g-TRGO2/GNP, PP/PP-g-TRGO4/GNP, and PP/PP-g-TRGO6/GNP nanocomposites
The O2 barrier properties of PP/GNP nanocomposites, with a GNP loading of 1.4 wt%, and a PP-g-TRGO(2, 4, or 6) compatibilizer content of either 0.1 wt% or 0 wt%, were investigated. The O2 barrier properties of PP/PP-g-TRGO2/GNP, PP/PP-g-TRGO4/GNP, and PP/PP-g-TRGO6/GNP nanocomposites show, respectively, 3%, 13%, and 14% increases compared with PP/GNP nanocomposites. The O2 barrier property of PP/PP-g-TRGO6/GNP was most improved, compared with the PP/GNP nanocomposites. This enhancement was attributed firstly to the well-dispersed graphene content in the PP matrix owing to the strong interfacial interactions between TRGO6 and graphene, and to the high crystallinity of PP/PP-g-TRGO6/GNP, similarly to the mechanical properties already discussed. These features of the PP/PP-g-TRGO6/GNP nanocomposites suggest that pathways for gas diffusion in the PP matrix are increased by both the two-dimensional structure of graphene and spherulitic structure of the PP crystals (Fig. 11).
 |
| Fig. 11 O2 permeability of PP/GNP, PP/PP-g-TRGO2/GNP, PP/PP-g-TRGO4/GNP, and PP/PP-g-TRGO6 nanocomposites with GNP (1.4 wt%) and PP-g-TRGO2/4/6 (0.1 wt%). | |
Conclusions
In summary, we have synthesized three types of TRGO-based compatibilizers, with varied degrees of graphene reduction, by controlling the temperature at which GO was reduced. These compatibilizers improve the interfacial interactions between graphene and PP, leading to improved dispersion of graphene in a PP matrix. FT-IR, XPS, NMR, and TGA results confirmed the successful fabrication of PP-g-TRGO2, PP-g-TRGO4, and PP-g-TRGO6 compatibilizers. The mechanical, electrical and gas barrier properties of PP/PP-g-TRGO2/GNP, PP/PP-g-TRGO4/GNP, and PP/PP-g-TRGO6/GNP nanocomposites were improved over those of PP/GNP nanocomposites that lacked a compatibilizer. The crystallinity of the nanocomposites also increased, because of the increased nucleating effect of graphene facilitated by the compatibilizers. The improved properties achieved with the use of TRGO-based compatibilizers are attributed to the improved dispersion of graphene in the PP matrix, strong interfacial interactions between graphene and PP, and high crystallinity of the nanocomposites. Among the three compatibilizers, PP-g-TRGO6 showed the most improved mechanical, electrical and barrier properties, even at very low loadings of 0.1 wt%. These superior results were attributed to the increased sp2-hybridised content of the more highly reduced TRGO6, which facilitates greater dispersion of graphene in the PP matrix by increased availability of strong π–π interactions between graphene and TRGO6. This effect was evident even though the TRGO content of PP-g-TRGO6 was lower than in either PP-g-TRGO2 or PP-g-TRGO4.
Acknowledgements
This research was supported by the KUSTAR-KAIST Institute, KAIST, Korea.
References
- M. C. Celina, Polym. Degrad. Stab., 2013, 98(12), 2419 CrossRef CAS.
- M. A. Chinelatto and J. A. M. Agnelli, Polym. Degrad. Stab., 1995, 50(1), 13 CrossRef CAS.
- M. Mucha, J. Marszalek and A. Fidrych, Polymer, 2000, 41(11), 4137 CrossRef CAS.
- S. Xu, M. Wen, J. Li, S. Guo, M. Wang, Q. Du, J. Shen, Y. Zhang and S. Jiang, Polymer, 2008, 49(22), 4861 CrossRef CAS.
- W. Gao, Y. Zheng, J. Shen and S. Guo, ACS Appl. Mater. Interfaces, 2015, 7(3), 1541 CAS.
- M. Drubetski, A. Siegmann and M. Narkis, J. Mater. Sci., 2007, 42(1), 1 CrossRef CAS.
- R. J. Foster, P. J. Hine and I. M. Ward, Polymer, 2009, 50(16), 4018 CrossRef CAS.
- R. M. Novais, J. A. Covas and M. C. Paiva, Composites, Part A, 2012, 43(6), 833 CrossRef CAS.
- A. J. Paleo, J. Silva, F. W. J. van Hattum, S. Lanceros-Mendez and A. I. Ares, J. Polym. Sci., Part B: Polym. Phys., 2013, 51(3), 207 CrossRef CAS.
- A. Ameli, P. U. Jung and C. B. Park, Carbon, 2013, 60, 379 CrossRef CAS.
- S. H. Han, H. J. Oh and S. S. Kim, Compos. Sci. Technol., 2014, 91, 55 CrossRef CAS.
- T. Kashiwagi, E. Grulke, J. Hilding, K. Groth, R. Harris, K. Butler, J. Shields, S. Kharchenko and J. Douglas, Polymer, 2004, 45(12), 4227 CrossRef CAS.
- B. X. Yang, K. P. Pramoda, G. Q. Xu and S. H. Goh, Adv. Funct. Mater., 2007, 17, 2062 CrossRef CAS.
- A. A. Koval’chuk, A. N. Shchegolikhin, V. G. Shevchenko, P. M. Nedorezova, A. N. Klyamkina and A. M. Aladyshev, Macromolecules, 2008, 41, 3149 CrossRef.
- J. I. Lee, S. B. Yang and H. T. Jung, Macromolecules, 2009, 42, 8328 CrossRef CAS.
- J. Xu, C. Chen, Y. Wang, H. Tang, Z. Li and B. S. Hsiao, Macromolecules, 2011, 44, 2808 CrossRef CAS.
- J. Yang, Y. Huang, Y. Lv, P. Zhao, Q. Yang and G. Li, J. Mater. Chem. A, 2013, 1, 11184 CAS.
- A. S. Robert and T. C. Frances, Composites, Part A, 2012, 43, 1092 CrossRef.
- F. d. C. Fim, J. M. Guterres, N. R. Basso and G. B. Galland, J. Polym. Sci., Part A: Polym. Chem., 2010, 48, 692 CrossRef CAS.
- Y. J. Huang, Y. W. Qin, Y. Zhou, H. Niu, Z. Z. Yu and J. Y. Dong, Chem. Mater., 2010, 22, 4096 CrossRef CAS.
- V. G. Shevchenko, S. V. Polschikov, P. M. Nedorezova, A. N. Klyamkina, A. N. Shchegolikhin and A. M. Aladyshev, Polymer, 2012, 53, 5330 CrossRef CAS.
- P. Song, Z. Cao, Y. Cai, L. Zhao, Z. Fang and S. Fu, Polymer, 2011, 52, 4001 CrossRef CAS.
- Y. S. Yun, Y. H. Bae, D. H. Kim, J. Y. Lee, I. Chin and H. Jin, Carbon, 2011, 49, 3553 CrossRef CAS.
- Y. Cao, J. Feng and P. Wu, J. Mater. Chem., 2012, 22, 14997 RSC.
- N. Song, J. Yang, P. Ding, S. Tang, Y. Liu and L. Shi, Ind. Eng. Chem. Res., 2014, 53, 19951 CrossRef CAS.
- F. Qiu, Y. Hao, X. Li, B. Wang and M. Wang, Composites, Part B, 2015, 71, 175 CrossRef CAS.
- C. Zhang, T. Wang, X. Gu and L. Feng, RSC Adv., 2015, 5, 65058 RSC.
- F. You, D. Wang, X. Li, M. Liu, G. H. Hu and Z. M. Dang, RSC Adv., 2014, 4, 8799 RSC.
- J. Z. Xu, Y. Y. Liang, G. J. Zhong, H. L. Li, C. Chen, L. B. Li and Z. M. Li, J. Phys. Chem. Lett., 2012, 3, 530 CrossRef CAS PubMed.
- J. S. Fan, R. J. Huang, S. B. Ye, T. J. Li and J. C. Feng, Polymer, 2014, 55, 4341 CrossRef CAS.
- S. H. Ryu and A. M. Shanmugharaj, Chem. Eng. J., 2014, 244, 552 CrossRef CAS.
- B. Yuan, C. Bao, L. Song, N. Hong, K. M. Liew and Y. Hu, Chem. Eng. J., 2014, 237, 411 CrossRef CAS.
- Y. Cao, J. Feng and P. Wu, Carbon, 2010, 48, 1683 CrossRef CAS.
- M. El Achaby, F.-E. Arrakhiz, S. Vaudreuil, K. Qaiss el, M. Bousmina and O. Fassi-Fehri, Polym. Compos., 2012, 33, 733 CrossRef CAS.
- K. S. Novoselov, A. K. Geim, S. V. Morozov, D. Jiang, Y. Zhang, S. V. Dubonos, I. V. Grigorieva and A. A. Firsov, Science, 2004, 306, 666 CrossRef CAS PubMed.
- D. Li, M. B. Muller, S. Gilje, R. B. Kaner and G. G. Wallace, Nat. Nanotechnol., 2008, 3, 101 CrossRef CAS PubMed.
- H. Kim, A. A. Abdala and C. W. Macosko, Macromolecules, 2010, 43, 6515 CrossRef CAS.
- T. Ramanathan, A. A. Abdala, S. Stankovich, D. A. Dikin, M. Herrera-Alonso and R. D. Piner, Nat. Nanotechnol., 2008, 3, 327 CrossRef CAS PubMed.
- B. Debelak and K. Lafdi, Carbon, 2007, 45, 1727 CrossRef CAS.
- H. Kim and C. W. Macosko, Macromolecules, 2008, 41, 3317 CrossRef CAS.
- M. A. Rafiee, J. Rafiee, Z. Wang, H. Song, Z. Z. Yu and N. Koratkar, ACS Nano, 2009, 3, 3884 CrossRef CAS PubMed.
- Y. R. Lee, A. V. Raghu, H. M. Jeong and B. K. Kim, Macromol. Chem. Phys., 2009, 210, 1247 CrossRef CAS.
- J. Liang, Y. Huang, L. Zhang, Y. Wang, Y. Ma and T. Guo, Adv. Funct. Mater., 2009, 19, 2297 CrossRef CAS.
- X. Zhao, Q. Zhang, D. Chen and P. Lu, Macromolecules, 2010, 43, 2357 CrossRef CAS.
- Y. Xu, W. Hong, H. Bai, C. Li and G. Shi, Carbon, 2009, 47, 3538 CrossRef CAS.
- A. V. Raghu, Y. R. Lee, H. M. Jeong and C. M. Shin, Macromol. Chem. Phys., 2008, 209, 2487 CrossRef CAS.
- D. W. Wang, F. Li, J. P. Zhao, W. C. Ren, Z. G. Chen and J. Tan, ACS Nano, 2009, 3, 1745 CrossRef CAS PubMed.
- M. M. Stylianakis, E. Stratakis, E. Koudoumas, E. Kymakis and S. H. Anastasiadis, ACS Appl. Mater. Interfaces, 2012, 4, 4864 CAS.
- J. Zhu, H. Gu, Z. Luo, N. Haldolaarachige, D. P. Young, S. Wei and Z. Guo, Langmuir, 2012, 28, 10246 CrossRef CAS PubMed.
- Y. F. Huang and C. W. Lin, Polymer, 2012, 53, 2574 CrossRef CAS.
- L. M. Veca, M. J. Meziani, W. Wang, X. Wang, F. Lu and P. Zhang, Adv. Mater., 2009, 21, 2088 CrossRef CAS.
- M. A. Rafiee, J. Rafiee, I. Srivastava, Z. Wang, H. Song and Z. Z. Yu, Small, 2010, 6, 179 CrossRef CAS PubMed.
- H. Kim, Y. Miura and C. W. Macosko, Chem. Mater., 2010, 22, 3441 CrossRef CAS.
- S. Morimune, T. Nishino and T. Goto, ACS Appl. Mater. Interfaces, 2012, 4, 3596 CAS.
- Y. Q. Li, T. Y. Yang, T. Yu, L. X. Zheng and K. Liao, J. Mater. Chem., 2011, 21, 10844 RSC.
- S. Morimune, T. Nishino and T. Goto, Polym. J., 2012, 44, 1056 CrossRef CAS.
- H. J. Salavagione, M. A. Gómez and G. Martínez, Macromolecules, 2009, 42, 6331 CrossRef CAS.
- Y. Lin, J. Jin and M. Song, J. Mater. Chem., 2011, 21, 3455 RSC.
- H. Kim, S. Kobayashi, M. A. AbdurRahim, M. J. Zhang, A. Khusainova and M. A. Hillmyer, Polymer, 2011, 52, 1837 CrossRef CAS.
- T. Kuila, S. Bose, C. E. Hong, M. E. Uddin, P. Khanra, N. H. Kim and J. H. Lee, Carbon, 2011, 49, 1033 CrossRef CAS.
- N. H. Kim, T. Kuila and J. H. Lee, J. Mater. Chem. A, 2014, 2, 2681 CAS.
- P. Aggarwal, N. Raghu, A. Karmarkar and S. Chuahan, Mater. Des., 2013, 43, 112 CrossRef CAS.
- Q. Yuan and R. D. K. Misra, Polymer, 2006, 47, 4421 CrossRef CAS.
Footnote |
† Electronic supplementary information (ESI) available. See DOI: 10.1039/c6ra17934g |
|
This journal is © The Royal Society of Chemistry 2016 |