DOI:
10.1039/C6RA17747F
(Paper)
RSC Adv., 2016,
6, 80522-80528
Influence of a non-biodegradable porous structure on bone repair
Received
12th July 2016
, Accepted 15th August 2016
First published on 17th August 2016
Abstract
Pore and interconnection size are two key structural parameters for bone tissue engineering scaffolds. In the present study, four hydroxyapatite (HA) bioceramic scaffolds with similar pore size (500–600 μm) but different interconnection sizes (100, 120, 150 and 200 μm), and three HA scaffolds with similar interconnection size (120 μm) but different pore sizes (400–500, 500–600 and 600–700 μm) were implanted into distal femoral condyle defects for 72 rabbits. The results showed that volume of new bone increased proportionally to the interconnection size. However, significant differences between interconnection size groups were only observed at week 24. Smaller pore size showed significantly more new bone formation at week 4, whereas no significant differences between the three pore size groups were found at weeks 12 and 24. In conclusion, large interconnection size facilitates new bone formation and a minimum size of 120 μm is recommended. Although small pore size improves bone ingrowth at early time points, pore size has limited impact on bone ingrowth later.
1. Introduction
There is an increased demand for bone replacement materials worldwide due to an aging population.1 Annually around 2.2 million bone grafting procedures are carried out worldwide with most of them using autograft tissue.2 Due to the limited availability of autologous bone and donor site morbidity, tissue engineering strategies present a promising alternative for the repair and reconstruction of bone defects.3 Tissue engineered bone requires three main elements, a progenitor or mature cell source, growth factors and a porous scaffold. A porous scaffold is an essential carrier for cells and growth factors, thus permitting bone in-fill in critical-sized defects. Furthermore, it provides temporary mechanical support and osteoconductivity to support new bone regeneration.3,4
Biomaterials for bone tissue engineering applications have been widely studied.5–9 As a commonly used implant material for bone, hydroxyapatite (HA) has attracted much scientific interest because of its similarity in composition to natural bone and excellent biocompatibility. In addition, it can be used as scaffolds for delivery of cells and growth factors, including bone morphogenic protein-2 (BMP-2) and transforming growth factor-beta 2 (TGF-β2) to name a few.10–15
The porous architecture, which facilitates bone ingrowth throughout the three-dimensional (3D) scaffolds, is an essential characteristic to ensure material bioactivity. It is well documented in previous studies that the macropore size of the biomaterial plays a significant role in the dynamics and extent of bone ingrowth inside the material. Many studies have investigated optimum macropore size and the current consensus is that a minimum pore size of 100 μm permits mineralized bone formation through appropriate cell migration and nutrition transport.16,17 Other studies reported that pore size of 100–400 μm support bone ingrowth and mineralization.18,19 However, alternative findings observed bone ingrowth in material with pore sizes inferior to 100 μm.20 It should be noted that many of these studies have assessed fast resorbable bone substitutes, where material degradation and porous structural changes are rapid. Consequently, there is still much to learn on the long-term effect of pore size on bone ingrowth. Besides macropore size, pore interconnection size is also another important factor in bone tissue engineering. It is reported that interconnection size directly influences cell behaviour and blood vessel formation.21 To date, many studies have focused on macropore size, neglecting the important parameters of scaffold interconnectivity.
Hydroxyapatite scaffolds have a slow biodegradation rate, therefore their unaltered macrostructure during the experiment allowed a precise observation of the link between the physical properties of the scaffold and new bone formation. The aim of this study is to evaluate the long-term influence of pore and interconnection size on bone ingrowth in vivo.
2. Experimental
2.1. Materials and methods
Material fabrication and preparation. Cylindrical porous HA bioceramics (diameter = 5 mm, length = 10 mm) were synthesized using a porogen agent following procedure described by Descamps et al.22,23 in which, an organic skeleton was firstly formed by chemically welding polymethylmethacrylate (PMMA) balls with fixed granulometric distribution. Aqueous calcium phosphate slurry was then poured inside voids between polymeric beads, debinded (removal of the organic scaffold) at 400 °C and sintered at 1270 °C. Samples of different pore sizes and interconnection sizes were prepared and divided into two groups. The first group had similar macropore size (500–600 μm) and different interconnection sizes (100 μm, 120 μm, 150 μm and 200 μm, respectively). The second group had similar interconnection size of 120 μm and different macropore sizes (400–500 μm, 500–600 μm and 600–700 μm, respectively). All materials were sterilized in an autoclave for animal surgery.
Characterization of macrostructure. The structure of the porous HA bioceramics was characterized using field emission scanning electron microscopy (FESEM; JS3-6700F, JEOL, Japan). Two samples from each group were prepared, gold-coated and analysed using FESEM. The macropore size was determined by averaging diameter of 30 different macropores on SEM images, following the method described in ISO 13175-3.24 Likewise, interconnection size was obtained by averaging the diameter of 30 whole interconnections.
Animals. Seventy-two New Zealand white rabbits (three months old) weighing approximately 2.5 kg, were obtained from the Laboratory Animal Center of Shanghai Institute for Biological Sciences (Shanghai, China, Certificate number SCXK 2007-0005). The protocol for use of the rabbits was approved by the Animal Ethics Committee of Shanghai Jiaotong University School of Medicine. The rabbits were randomly divided into six groups of three for each type of bioceramic. Two samples were implanted per rabbit, resulting in six samples of each bioceramic type for each observation time (0, 4, 12 and 24 weeks). For the duration of the experiment, rabbits were housed individually in cages and provided free access to water and fed a commercial pellet diet.
Implantation. After anesthesia with a mixture of ketamine hydrochloride (60 mg kg−1) and xylazine (6 mg kg−1), the bilateral hind limbs were shaved and the skin disinfected with iodine solution. The distal femoral condyle was exposed by a longitudinal lateral incision on the outside of the knee. A cylindrical cavity of 5 mm diameter and 10 mm depth was carefully drilled into the femoral condyle with a manual-driven drill and successive burs of 2.5 mm, 3.5 mm, 4.5 mm and 5 mm in diameter. The defect was irrigated with sterile saline solution to remove bone debris. Porous HA bioceramics were implanted into the cavity by manual press-fit insertion. Finally, the incision was closed in layers. Identical technique was used for the opposing femur at the same surgery time.
Histology. Rabbits were sacrificed at defined intervals and the distal femoral condyles were immediately fixed in 70% ethanol for 10 days, dehydrated in a graded ethanol series and cleared with toluene. Samples were then embedded in polymethylmethacrylate. After hardening, sections of 100 μm thick were cut perpendicular to the implants using a Leica SP 1600 (Leitz, Wetzlar, Germany) under cooling water. The sections were ground to 50 μm thick, polished and stained with Van Gieson's picro-fuchsine staining. Samples were then observed using light microscopy.
Histomorphometry. Pore number, interconnection number and interconnection rate of each bioceramic were measured on the 50 μm thick sections (magnification ×100). In which, the• Pore number represents the average pore number from each field of view.
• Interconnection number represents the average interconnection number from each field of view.
• Interconnection rate was calculated from the interconnection number divided by pore number and represents the average interconnection number in each pore.
An eyepiece micrometer (KPL 16, Carl Zeiss, Germany) and 100-point ocular integrator (KPL 8, Zeiss, Germany) were used to measure the following histomorphometric parameters (magnification ×128). Two sections per sample were observed using light microscopy. In which, the
• Residual material volume (%) represents the percentage of implant occupied by residual material.
• Bone volume (%) represents the percentage of implant occupied by new bone tissue.
• Osteoid volume (%) represents the percentage of implant occupied by osteoid tissue.
Relative osteoid volume was calculated by dividing the osteoid volume by the bone volume and represents the percentage of bone volume occupied by osteoid tissue.
Statistical analysis. Results are expressed as a mean ± standard deviation. Data were analyzed using the analysis of variance (ANOVA) test and p < 0.05 was considered significant. The correlations between bone volume and interconnection size, as well as bone volume and pore size, were studied with the Pearson correlation coefficient (r).
3. Results
3.1. Porous HA bioceramics characterization
The surface morphology of the porous bioceramics is shown in Fig. 1. The pores are round and distributed uniformly. Each type of porous bioceramic had a well-interconnected structure in absence of blocked pores. The average measured macropore size was close to the lower limit of theoretical pore size range, while the difference between theoretical and actual average interconnection size was less than four micrometers. Differences between actual and theoretical macropore size, interconnection size and ratio in all porous bioceramic types were less than 15%. Our fabrication process allowed total control of the porous architecture, including macropore size and interconnection size, which enabled further study into the biological effect of these parameters.
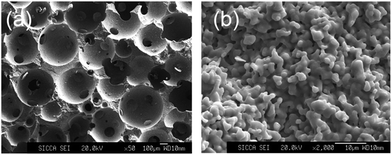 |
| Fig. 1 SEM of bioceramics with 500–600 μm pore size and 120 μm interconnection size. (a) Low magnification at ×50. (b) High magnification at ×2000. | |
Pore number, interconnection number and interconnection rate are shown in Fig. 2. When pore size remained constant, all parameters decreased with decreasing interconnection size. Interconnection number and rate of interconnection in the 200 μm group were around two to three times higher than in the 100 μm group. On the opposite, with constant interconnection size, interconnection rate increased proportionally to the pore size, whereas the latter had an inverse effect on pore and interconnection number. About twice more pores were observed in the 400–500 μm group than the 600–700 μm group.
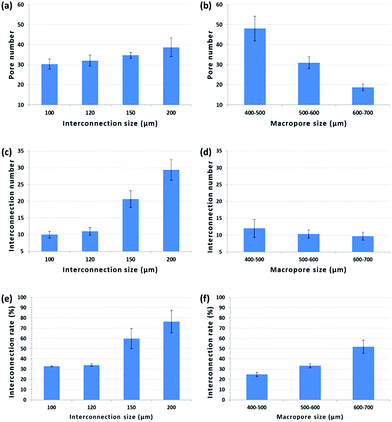 |
| Fig. 2 Pore number (a and b), interconnection number (c and d) and interconnection rate (%) (e and f) for different interconnection size groups (a, c and e) and different pore size groups (b, d and f). | |
3.2. Residual material volume (RMV)
The RMV for the six groups accounted for 40% of the visions immediately following implantation and differences in RMV between groups were not significant (Fig. 3). These data indicate that the porosity of these groups is approximately 60%. Between 4 and 24 weeks following implantation, RMV was stabilized between 30% and 40% at all-time points.
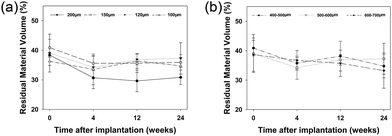 |
| Fig. 3 Residual material volume of different interconnection size groups (a) and different pore size groups (b). | |
3.3. The relationship of interconnection size and bone volume
In the first group with similar macropore size (500–600 μm) and different interconnection sizes (100 μm, 120 μm, 150 μm, 200 μm), bone volume was found to increase with time and new bone formation initiated from the host bone bed, extending from the periphery to the centre (Fig. 4 and 5a). Four weeks after implantation, small patches of new bone (red stain) formed on the majority of macropore walls. Soft tissue was seen throughout the entire bioceramics. At 12 weeks, new bone emerged in all macropores, which began to partially fuse. However, no significant differences were found for bone volume within materials with different interconnection size, both at 4 and 12 weeks after implantation. After 24 weeks, some macropores were completely filled with new bone and decreased bone volume coincided with decreasing interconnection size. The amount of new bone was statistically higher in bioceramics with a 200 μm interconnection size compared to those with 100 μm interconnection size (p < 0.01). However, within the groups with 120 μm, 150 μm and 200 μm interconnection size, no significant differences could be found. Correlation was observed at 24 weeks between interconnection size (100 μm, 120 μm, 150 μm, 200 μm) and bone formation (r = 0.622, p = 0.010).
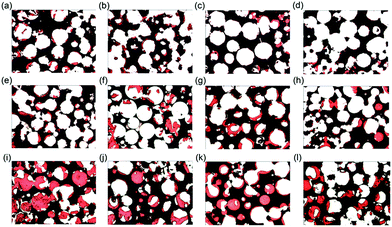 |
| Fig. 4 Hard tissue section of 200 μm (a, e and i), 150 μm (b, f and j), 120 μm (c, g and k) and 100 μm (d, h and l) pore interconnection size at 4 weeks (a–d), 12 weeks (e–h) and 24 weeks (i–l) after implantation (magnification ×100). | |
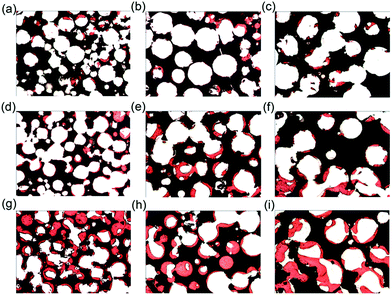 |
| Fig. 5 Hard tissue section of 400–500 μm (a, d and g), 500–600 μm (b, e and h) and 600–700 μm (c, f and i) pore size at 4 weeks (a–c), 12 weeks (d–f) and 24 weeks (g–i) after implantation (magnification ×100). | |
3.4. The relationship between the pore size and new bone volume
In the second group with constant interconnection size (120 μm) but varying pore sizes (400–500 μm, 500–600 μm and 600–700 μm), increasing pore size resulted in the decrease of new bone volume at each time point (Fig. 5b and 6). Four weeks after implantation, both 400–500 μm and 500–600 μm pore size groups had significantly higher bone volume compared to the 600–700 μm pore size group (p < 0.01). Correlation was observed at 4 weeks between pore size (400–500 μm, 500–600 μm, 600–700 μm) and bone formation (r = 0.784, p = 0.002). However, at 12 and 24 weeks, no statistically significant differences for bone volume between groups were detected.
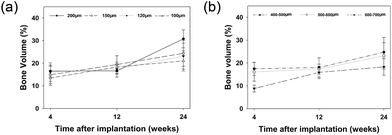 |
| Fig. 6 Bone volume of different interconnection size groups (a) and different pore size groups (b). | |
3.5. The relationship between the interconnection size and osteoid volume
On the surface of newly formed bone, osteoid tissue was observed at every time points with cubical-shaped osteoblasts lining these areas (data not shown). At 4 weeks, no statistical difference in osteoid volume was found between groups (Fig. 7a). At 12 weeks, osteoid volume in the 120 μm, 150 μm and 200 μm interconnection size groups decreased, but an increase was observed within the 100 μm interconnection size group with significantly higher volume compared to the groups with interconnection size of 150 μm (p < 0.01) and 120 μm (p < 0.05). However, at 24 weeks, higher osteoid volume was observed in the 200 μm interconnection size group than in both 150 μm (p < 0.05) and 120 μm (p < 0.05) groups.
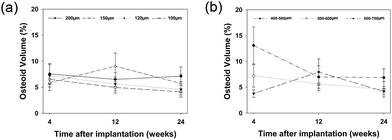 |
| Fig. 7 Osteoid volume of different interconnection size groups (a) and different pore size groups (b). | |
Similar observation applied to osteoid volume/bone volume ratio at 4 and 12 weeks (Fig. 8a). However, 24 weeks after implantation, the 100 μm interconnection size group had the highest ratio due to its low bone volume.
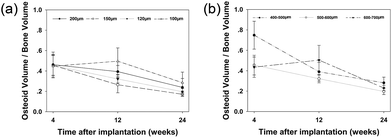 |
| Fig. 8 Osteoid volume/bone volume ratio of different interconnection size groups (a) and different pore size groups (b). | |
3.6. The relationship between the pore size and osteoid volume
At 4 weeks, osteoid volume was inversely proportional to pore size (Fig. 7b). Significantly more osteoid volume was present in the 400–500 μm pore size group compared to the 500–600 μm group (p < 0.01), and in the latter compared to the 600–700 μm group (p < 0.01). At 12 weeks, no statistically significant differences were found among the different pore size groups. However, at 24 weeks, significantly more osteoid volume was observed in the 400–500 μm pore size group compared to the two other groups (p < 0.05).
The osteoid volume/bone volume ratio was significantly higher in the 400–500 μm pore size group than in the other two groups at 4 weeks (p < 0.01) (Fig. 8b). However, at 12 weeks, the 600–700 μm pore size group had the highest osteoid volume/bone volume ratio, whereas at 24 weeks, no statistically significant differences were found between the three groups.
4. Discussion
Hydroxyapatite was chosen as a bone substitute for this study due to its excellent biocompatibility and slow degradation properties. It is reported that after 24 weeks, only 5% of HA implants resorb, while 55% of tricalcium phosphate (TCP) implants resorb in rabbit femoral and tibial condyle defect models.23 Contrary to previous studies, the unchanged macrostructure allows us to analyse precisely the effect of its physical properties on the formation of new bones, which can be extended to its impact in other non-biodegradable materials such as metallic implants. During the 24 weeks observation time of this experiment, the architecture of the HA implants was almost unchanged. However, between 0 and 4 weeks, residual material volume decrease was more important than at the other time points, probably due to the fact that part of the residual material was covered by the newly formed bone on the pore wall.
As reported in previous studies, architectural parameters of porous bone substitutes influence cell migration, vascularization and new bone formation.19,25–29 In addition to pore size, pore interconnection size is an important parameter for new bone formation. Both parameters were therefore taken into consideration and the experiment was divided into two groups: (1) bioceramics with similar pore size and different interconnection sizes; (2) bioceramics with similar interconnection size and different pore sizes. Accurate control of these parameters was possible thanks to the fabrication technique chosen.
The internal physical structure of most porous biomaterials, including interconnection size, carries intrinsic limits for blood vessel supply and subsequent bone ingrowth.30 In vivo studies report that a 10 μm interconnection size only allows cell penetration with fibrous and chondroid tissue formation, whilst new bone ingrowth within the pores requires an interconnection size over 50 μm.21 It has been demonstrated that the minimum channel diameter is approximately 80 μm for cell penetration31 and a diameter larger than 100 μm is recommended for osteoconduction and vascularization.30,32 Thus, the minimum interconnection size fabricated in our study was 100 μm. Differences in bone volume, influenced by interconnection size from 100 μm to 200 μm, were apparent 24 weeks after implantation. At this time point, bone volume increased proportionally to interconnection size, but no significant differences were found between the 120 μm, 150 μm and 200 μm interconnection size groups. This indicates that a larger interconnection size facilitates bone ingrowth, especially 24 weeks after implantation and a minimum size of 120 μm is recommended. Interestingly, the 100 μm interconnection size group showed high osteoid volume and osteoid volume/bone volume ratio after 4 weeks. However, this large amount of osteoid failed to mineralize at later time points: small interconnection size restricts the number and the diameter of blood vessels running through the pores, limiting blood supply.33,34 Scaglione et al. reported that approximately 30% of blood vessels exhibit a mean lumen diameter between 60 and 80 μm and more than 70% of blood vessels display a mean lumen diameter above 60 μm within scaffolds.33 Klenke et al. noticed that significantly more functional capillary is formed when internal size limitation of the macrostructure is superior to 140 μm.35 Furthermore, when interconnection size increased from 100 μm to 200 μm, a proportional correlation was observed on pore number, interconnection number and interconnection rate. It has been hypothesized that interconnection size and density are equally important in non-resorbable materials,21,36 which might explain the influence of interconnection size on bone formation.
Biomaterial pore size is important for efficient bone regeneration as they must be large enough to allow cell migration, effective nutrient supply and metabolic waste removal. Nevertheless, pores must remain small enough to provide a sufficient surface area for efficient cell binding and cell–cell interaction.37,38 Previous studies report an absence of new bone formation in HA constructs with 200 μm pore size.39 Scaffolds with 380–405 μm pore size showed enhanced chondrocyte and osteoblast cell growth, whilst scaffolds with a 186–200 μm pore size were better for fibroblast cell growth.38 These findings resulted in selection of 400 μm as the smallest pore size to be studied herein. In our study, new bone volume decreased as pore size increased from 400 μm to 700 μm. However, significant differences were observed only at early time points (4 weeks), likely due to the cells reaching 3D confluence earlier in smaller pores. Earlier differentiation has been shown to occur with larger amounts of bone volume at early time points.40,41 For the 600–700 μm pore size group, osteoid volume increased from 4 to 12 weeks, due to the ability of larger pores to provide a higher surface area per HA volume for cell adherence and a larger internal space for cell proliferation.36 Although bone volume in 600–700 μm pore size group was lower at 4 weeks, it increased faster after 4 weeks. As a result, similar bone volumes were observed in the large pore compared to the small pore groups at 12 and 24 weeks. In addition to pore size, the degree of pore interconnection is also a crucial characteristic to allow cell migration into the scaffold.42,43 With respect to the sample tested, interconnection density was similar within the different pore size groups even if pore density was higher for smaller pores, explaining why no significant differences in bone formation were observed between the different pore size groups. Others similarly reported the limited role on bone formation of pore size, when ranging from 350 μm to 800 μm,44 and failure of pore size from 150 μm to 700 μm to affect osteoblast proliferation and function.45,46 Pore size in this range should be effective but non-detrimental to the bone formation process.47,48
There are some limitations in this investigation. First, in addition to pore and interconnection size, other structural properties have the potential to affect new bone formation, including pore shape, porosity and surface structure to name a few. This research focused solely on pore and interconnection with cycloid-shaped pores, similar porosity and no special surface treatment. Second, the hole-shaped defect in the distal femoral condyle of rabbits is a non-weight-bearing bone defect model. Therefore, the impact of pore and interconnection size on the mechanical properties were not studied. As mechanical strength and interconnection size are inversely proportional, the behaviour of the material when used in weight-bearing area should be taken into consideration. Nevertheless, most of the porous bioceramics on the market are indicated for non-weight-bearing.49,50
5. Conclusions
It was shown in the present study that for a given pore size range (400–700 μm), interconnection size is the key regulator of new bone formation in non-degradable hydroxyapatite scaffolds. During long-term observation (24 weeks) of HA material in vivo, new bone formation increased proportionally with pore interconnection size from 100 μm to 200 μm, the latter appearing to mainly influence late bone formation. A minimal interconnection size of 120 μm is recommended as osteoid tissue is poorly mineralized in interconnection smaller than this critical size. Even if smaller pore size in the range of 400–700 μm induces larger amounts of bone formation, significant increases are only found at early time points (4 weeks), limiting its effect on new bone formation. This observation could be extended to other non-degradable materials such as metals.
Acknowledgements
This work was financially supported by the National Basic Research Program of China (Grant No. 2012CB619100), the National Natural Science Foundation of China (Grant No. 51232002).
Notes and references
- K. A. Hing, Philos. Trans. R. Soc., A, 2004, 362, 2821–2850 CrossRef CAS PubMed.
- S. F. F. Malak and I. A. Anderson, Med. Eng. Phys, 2008, 30, 717–724 CrossRef PubMed.
- A. S. Mistry and A. G. Mikos, Adv. Biochem. Eng./Biotechnol., 2005, 94, 1–22 CrossRef PubMed.
- S. Bose, M. Roy and A. Bandyopadhyay, Trends Biotechnol., 2012, 30, 546–554 CrossRef CAS PubMed.
- J. Guan, J. Yang, J. Dai, Y. Qin, Y. Wang, Y. Guo, Q. Ke and C. Zhang, RSC Adv., 2015, 5, 36175–36184 RSC.
- S. C. Cox, J. A. Thornby, G. J. Gibbons, M. A. Williams and K. K. Mallick, Mater Sci Eng C, 2015, 47, 237–247 CrossRef CAS PubMed.
- Y. Xie, D. Chopin, C. Morin, P. Hardouin, Z. Zhu, J. Tang and J. Lu, Biomaterials, 2006, 27, 2761–2767 CrossRef CAS PubMed.
- Y. Gan, K. Dai, P. Zhang, T. Tang, Z. Zhu and J. Lu, Biomaterials, 2008, 29, 3973–3982 CrossRef CAS PubMed.
- J. Lu, A. Gallur, B. Flautre, K. Anselme, M. Descamps, B. Thierry and P. Hardouin, J. Biomed. Mater. Res., 1998, 42, 357–367 CrossRef CAS PubMed.
- X. Liu, K. Zhao, T. Gong, J. Song, C. Bao, E. Luo, J. Weng and S. Zhou, Biomacromolecules, 2014, 15, 1019–1030 CrossRef CAS PubMed.
- S. Kim, S. S. Kim, S. H. Lee, S. Eun Ahn, S. J. Gwak, J. H. Song, B. S. Kim and H. M. Chung, Biomaterials, 2008, 29, 1043–1053 CrossRef CAS PubMed.
- K. Na, Biomaterials, 2007, 28, 2631–2637 CrossRef CAS PubMed.
- Y. Sogo, A. Ito, T. Matsuno, A. Oyane, G. Tamazawa, T. Satoh, A. Yamazaki, E. Uchimura and T. Ohno, Biomed. Mater., 2007, 2, 116–123 CrossRef CAS PubMed.
- K. Sena, D. R. Sumner and A. S. Virdi, J. Biomed. Mater. Res., Part A, 2010, 92, 1210–1217 Search PubMed.
- X. Cao and D. Chen, Gene, 2005, 357, 1–8 CrossRef CAS PubMed.
- J. Klawitter and S. Hulbert, J. Biomed. Mater. Res., 1971, 5, 161–229 CrossRef.
- J. Rouwkema, N. C. Rivron and C. A. van Blitterswijk, Trends Biotechnol., 2008, 26, 434–441 CrossRef CAS PubMed.
- M. Bohner and F. Baumgart, Biomaterials, 2004, 25, 3569–3582 CrossRef CAS PubMed.
- C. M. Murphy, M. G. Haugh and F. J. O'Brien, Biomaterials, 2010, 31, 461–466 CrossRef CAS PubMed.
- A. I. Itala, H. O. Ylanen, C. Ekholm, K. H. Karlsson and H. T. Aro, J. Biomed. Mater. Res., 2001, 58, 679–683 CrossRef CAS PubMed.
- J. Lu, B. Flautre, K. Anselme, P. Hardouin, A. Gallur, M. Descamps and B. Thierry, J. Mater. Sci.: Mater. Med., 1999, 10, 111–120 CrossRef CAS PubMed.
- M. Descamps, T. Duhoo, F. Monchau, J. Lu, P. Hardouin, J. Hornez and A. Leriche, J. Eur. Ceram. Soc., 2008, 28, 149–157 CrossRef CAS.
- J. Lu, M. Descamps, J. Dejou, G. Koubi, P. Hardouin, J. Lemaitre and J.-P. Proust, J. Biomed. Mater. Res., 2002, 63, 408–412 CrossRef CAS PubMed.
- ISO 13175-3:2012, Implants for surgery — Calciumphosphates — Part 3: Hydroxyapatite and beta-tricalciumphosphate bone substitutes.
- K. Kim, D. Dean, J. Wallace, R. Breithaupt, A. G. Mikos and J. P. Fisher, Biomaterials, 2011, 32, 3750–3763 CrossRef CAS PubMed.
- B. W. Thimm, S. Wust, S. Hofmann, H. Hagenmuller and R. Muller, Acta Biomater., 2011, 7, 2218–2228 CrossRef CAS PubMed.
- Q. Fu, M. N. Rahaman, B. S. Bal, K. Kuroki and R. F. Brown, J. Biomed. Mater. Res., Part A, 2010, 95, 235–244 CrossRef PubMed.
- K. Kim, A. Yeatts, D. Dean and J. P. Fisher, Tissue Eng., Part B, 2010, 16, 523–539 CrossRef CAS PubMed.
- M. Bohner, Y. Loosli, G. Baroud and D. Lacroix, Acta Biomater., 2011, 7, 478–484 CrossRef CAS PubMed.
- M. Mastrogiacomo, S. Scaglione, R. Martinetti, L. Dolcini, F. Beltrame, R. Cancedda and R. Quarto, Biomaterials, 2006, 27, 3230–3237 CrossRef CAS PubMed.
- F. R. Rose, L. A. Cyster, D. M. Grant, C. A. Scotchford, S. M. Howdle and K. M. Shakesheff, Biomaterials, 2004, 25, 5507–5514 CrossRef CAS PubMed.
- M. W. Betz, A. B. Yeatts, W. J. Richbourg, J. F. Caccamese, D. P. Coletti, E. E. Falco and J. P. Fisher, Biomacromolecules, 2010, 11, 1160–1168 CrossRef CAS PubMed.
- S. Scaglione, C. Ilengo, M. Fato and R. Quarto, Tissue Eng., Part A, 2009, 15, 155–163 CrossRef CAS PubMed.
- F. Bai, Z. Wang, J. Lu, J. Liu, G. Chen, R. Lv, J. Wang, K. Lin, J. Zhang and X. Huang, Tissue Eng., Part A, 2010, 16, 3791–3803 CrossRef CAS PubMed.
- F. M. Klenke, Y. Liu, H. Yuan, E. B. Hunziker, K. A. Siebenrock and W. Hofstetter, J. Biomed. Mater. Res., Part A, 2008, 85, 777–786 CrossRef PubMed.
- P. Kasten, I. Beyen, P. Niemeyer, R. Luginbuhl, M. Bohner and W. Richter, Acta Biomater., 2008, 4, 1904–1915 CrossRef CAS PubMed.
- J. A. Sanz-Herrera, J. M. Garcia-Aznar and M. Doblare, Acta Biomater., 2009, 5, 219–229 CrossRef CAS PubMed.
- S. H. Oh, I. K. Park, J. M. Kim and J. H. Lee, Biomaterials, 2007, 28, 1664–1671 CrossRef CAS PubMed.
- J. H. Kuhne, R. Bartl, B. Frisch, C. Hammer, V. Jansson and M. Zimmer, Acta Orthop. Scand., 1994, 65, 246–252 CrossRef CAS PubMed.
- T. Mygind, M. Stiehler, A. Baatrup, H. Li, X. Zou, A. Flyvbjerg, M. Kassem and C. Bunger, Biomaterials, 2007, 28, 1036–1047 CrossRef CAS PubMed.
- C. Gorriz, F. Ribeiro, J. M. Guedes and P. R. Fernandes, Procedia Eng., 2015, 110, 82–89 CrossRef CAS.
- C. Vitale-Brovarone, M. Miola, C. Balagna and E. Verné, Chem. Eng. J., 2008, 137, 129–136 CrossRef CAS.
- L. Galois and D. Mainard, Acta Orthop. Belg., 2004, 70, 598–603 Search PubMed.
- S. M. Roosa, J. M. Kemppainen, E. N. Moffitt, P. H. Krebsbach and S. J. Hollister, J. Biomed. Mater. Res., Part A, 2010, 92, 359–368 CrossRef PubMed.
- S. L. Ishaug, G. M. Crane, M. J. Miller, A. W. Yasko, M. J. Yaszemski and A. G. Mikos, J. Biomed. Mater. Res., Part A, 1997, 36, 17–28 CrossRef CAS.
- Y. C. Wu, S. Y. Shaw, H. R. Lin, T. M. Lee and C. Y. Yang, Biomaterials, 2006, 27, 896–904 CrossRef CAS PubMed.
- H. W. Kim, S. Y. Shin, H. E. Kim, Y. M. Lee, C. P. Chung, H. H. Lee and I. C. Rhyu, J. Biomater. Appl., 2008, 22, 485–504 CrossRef CAS PubMed.
- M. C. von Doernberg, B. von Rechenberg, M. Bohner, S. Grunenfelder, G. H. van Lenthe, R. Muller, B. Gasser, R. Mathys, G. Baroud and J. Auer, Biomaterials, 2006, 27, 5186–5198 CrossRef CAS PubMed.
- W. Suchanek and M. Yoshimura, J. Mater. Res., 1998, 13, 94–117 CrossRef CAS.
- A. J. Salinas and M. Vallet-Regí, Z. Anorg. Allg. Chem., 2007, 633, 1762–1773 CrossRef CAS.
|
This journal is © The Royal Society of Chemistry 2016 |