DOI:
10.1039/C6RA17446A
(Paper)
RSC Adv., 2016,
6, 89821-89826
p-Nitrophenol degradation and microbial community structure in a biocathode bioelectrochemical system†
Received
7th July 2016
, Accepted 3rd September 2016
First published on 6th September 2016
Abstract
The biocathode bioelectrochemical system (bioc-BES) was used for p-nitrophenol (PNP) degradation with sodium bicarbonate as the carbon source. The PNP degradation efficiency in bioc-BES with applied voltage of 0.5 V reached 96.1% ± 0.5% within 72 h, which was much higher than that obtained in the biocathode BES without applied voltage (bioc-BES-NAP), open circuit biocathode BES (OC-bioc-BES) or abiotic cathode BES (abioc-BES). With the increase in the PNP concentration from 20 mg L−1 to 60 mg L−1, the PNP concentration of the effluent decreased from 21.66 ± 2.94 mg L−1 to 0.38 ± 0.1 mg L−1 in 48 h. The final reductive product of PNP was p-aminophenol (PAP) in the biocathode chamber. High-throughput 454 pyrosequencing showed that predominant populations in the biocathode biofilm of bioc-BES were affiliated with electrochemically active Delftia and Diaphorobacter, while Aminobacter was the predominant population in the biocathode biofilm of the bioc-BES-NAP and OC-bioc-BES. These results suggested that microbial electrocatalysis and electrochemically active bacteria on the cathodic electrode accelerated PNP degradation in the biocathode BES (bioc-BES). The biocathode BES will be a promising treatment technology for PNP-containing wastewater.
Introduction
p-Nitrophenols (PNP), as common nitroaromatic compounds (NPs), were widely used as synthetic intermediates in dyes, explosives, fungicides, plasticizers and rubber chemicals.1,2 PNP is a priority pollutant in wastewaters for the United States Environmental Protection Agency.3 In recent years, PNP has also been detected in rivers, soils, wastewater and ground water, from the degradation of pesticides used in fields,4 which causes serious environmental problems.5 Nitrophenol oxidization is difficult due to the nitro group with its strong electron affinity.6 The NPs could be transformed to aminophenols with anaerobic reductive conditions. The toxicity of aromatic amines is 500-fold less than that of the corresponding nitroaromatics,7,8 and the aromatic amines are considerably easier to be mineralized.9,10
The bioelectrochemical systems (BESs) use microorganisms to generate different valuable chemicals and energy.11 Recently, microbial electrocatalysis on the cathode of BES was used as a new technology for enhancing various recalcitrant pollutants' treatment. Various pollutants were reduced with biocatalyzed cathodes including azo dyes,12–14 chlorophenols,15–17 chloroethenes,18,19 Cr(VI),20 U(VI),21 perchlorates,22 antibiotics23 in water, and particularly the removal of nitrobenzene8 and p-fluoronitrobenzene.24
BES combined with other conventional processes such as anaerobic sludge blanket reactor, bio-contact and the Fenton system were developed for enhancing the PNP removal.25,26 To date, biocathode BESs have been of special concern as sustainable technology without noble metal catalysts.27 Although nitrobenzene reduction and microbial communities in BES have been reported,8,28 PNP degradation in biocathode BES and the functional populations in microbial communities were not fully investigated. High-throughput sequencing of the taxonomically informative 16S rRNA gene can provide a powerful approach for exploring microbial diversity. In addition, no report has demonstrated the PNP removal using BES with inorganic carbon. In order to assess the contribution of electroactive bacterium accepting electrons from the cathode to reduce organic matter, PNP reduction and functional populations in the two-chamber BES with an inorganic carbon source fed biocathode should be further investigated.
This study investigated for the first time the PNP reduction in microbial electrocatalysis systems using an inorganic carbon source, to evaluate PNP removal efficiency in biocathode BES and conventional anaerobic degradation, and to reveal the microbial community structure of BES reactors using 454-pyrosequencing of 16S rRNA gene.
Materials and methods
BESs configuration
A two-chamber BES reactor was designed using two 300 mL glass bottles (Fig. 1). The two chambers of the BESs were separated by a cation exchange membrane (7 cm2) CMI-7000 (Membrane International Inc., USA). The graphite brush (5 cm diameter × 10 cm length; fiber type: T700-12K, Toray Industries Co., Ltd.) was used as the anode and cathode after pretreatment by 1 M NaOH and 1 M HCI for 12 h, respectively, then washed to neutral by deionized water. The batch tests were run over an external resistor of 10 Ω connected to a data acquisition system (Keithley 2700, Keithley Co., Ltd., USA) and the results were recorded every 30 min. An inserted Ag/AgCl reference electrode (+0.197 V vs. SHE) was closed to the cathode for potential measurement (model-217, Shanghai Precise. Sci. Instru. Co., Ltd., China).
 |
| Fig. 1 Schematic of the two-chamber biocathode biological electrochemical system: (1) graphite brush anode, (2) graphite brush biocathode, (3) cation exchange membrane, (4) clamp, (5) reference electrode, (6) rubber stopper. | |
Inoculation and operation of BESs
Wastewater as the inoculum was collected from primary clarifier of the Harbin Wenchang Wastewater Treatment Plant. The BES reactors with abiotic cathode were autoclaved (121 °C, 15 min) before inoculation. A medium including 50 mM phosphate buffer solution (PBS), trace minerals, vitamins and 1.67 g L−1 sodium acetate was used for enriching exoelectrogenic bacteria in the anode chamber.29,30 The same medium minus sodium acetate, which was replaced with NaHCO3 (1 g L−1) and PNP (20 mg L−1), was used to culture the biocathode biofilm. The reactors were operated in a temperature-controlled room at 25 °C ± 2 °C. All the tests were operated in fed-batch with a batch cycle, defined as the period during which the solution is refilled at regular intervals of 3–5 days to reduce the reaction time. All the experiments were conducted over at least three cycles. An external voltage of 0.5 V was applied on biocathode BES (bioc-BES) using a DC power supply except if otherwise mentioned. In order to evaluate the role of biocathode BES, the control tests including biocathode BES without applied voltage (bioc-BES-NAP), open circuit biocathode BES (OC-bioc-BES), abiotic cathode BES (abioc-BES) and open circuit abiotic cathode BES (OC-abioc-BES) were performed.
Chemical analysis
PNP (>98% purity, Aladdin Inc., Shanghai, China) and p-aminophenol (PAP) (98% purity, Aladdin Inc., Shanghai, China) were purchased and HPLC grade methanol was purchased from Sigma-Aldrich. A set of PNP (or PAP) standard solutions with concentrations between 0 and 100 mg L−1 were used to make a calibration curve. The quantification of the PNP or PAP was determined based on the calibration curve.
The samples were taken from the cathode chamber and filtered through the 0.45 μm pore filter membrane immediately. The concentration of PNP was measured by high performance liquid chromatography (HPLC, model-e2695, Waters Co., USA) at 30 °C and a UV-visible detector (model-2489 Waters, USA) was used for measurement at 317 nm. The methanol/water (pH = 3.0) (40
:
60 v/v) was used as the mobile phase with an RP18 column (5 μm, 3.9 × 150 mm) and pumped at a flow rate of 1.00 mL min−1.
The metabolites of PNP were measured by UV-vis spectrophotometry (TU-1810) and HPLC-MS (high performance liquid chromatography-mass spectrometry, Thermo Finnigan LCQ DECA XP MAX). The effluent was taken at 72 h for UV-vis spectrophotometry (pH = 9). The effluent from biocathode chamber was taken at 4, 8, 12, 24, 36, 48, 60 and 72 h of an operational cycle and then filtered through a 0.2 μm pore diameter filter for analysis by HPLC-MS. The detector was equipped with an electrospray ionization source and was operated in the positive/negative polarity mode. The intermediates were scanned in the normal mass range from 50 m/z (mass to charge ratio) to 450 m/z.23 The concentration of PAP was analysed by UV-visible spectrophotometry at 630 nm. The kinetics of PNP degradation were modeled using a first-order kinetic model C = C0e−kt, where C is the PNP concentration (mg L−1) at time t (h), and C0 is the initial PNP concentration (52.1 mg L−1). The rate constant k (1/h) was calculated by Origin 2016 software analysis.
DNA extraction
The graphite fibers were cut from the biocathode of the BES reactors using sterile scissors and sampled from the top, middle and bottom sections of the brush cathodes. Three parts were combined after rinsing with deionized water to remove the cathode solution.31 DNA was extracted using a PowerSoil DNA Isolation Kit (Mobio Laboratories, Inc., Carlsbad, CA) according to manufacturer's instructions. The quantity and quality of the extracted DNA were checked by measuring its absorbance at 260 nm and 280 nm using a Nanodrop N8000. The PCR amplification was performed as previously described.32
454 pyrosequencing of 16S rRNA gene and data analysis
Amplicon libraries were constructed for 454 pyrosequencing using bacterial primers 8F (5′-AGAGTTTGATCCTGGCTCAG-3′) and 533R (5′-TTACCGCGGCTGCTGGCAC-3′) for the V1–V3 region of the 16S rRNA gene.31 Amplicon of 16S rRNA gene was pooled in equimolar concentrations to obtain similar numbers, which were sequenced using 454 GS-FLX Pyrosequencing System (Roche Diagnostics Corporation). The abundance of a given phylogenetic group was defined by the proportion of the number of sequences affiliated to that group of the total number of obtained sequences. Operational taxonomic units (OTUs) were clustered with 97% similarity by the 454 pyrosequencing data, and microbial diversity and species richness were determined based on a threshold of 97% using the MOTHUR program (http://www.mothur.org/wiki/Main_Page).
Results
Biocathode BES accelerate PNP degradation
Biocathode BES (bioc-BES) showed the highest PNP degradation efficiency of 74% ± 5.7% within 48 h, while the open-circuit biocathode BES (OC-bioc-BES), biocathode BES without applied voltage (bioc-BES-NAP) and abiotic cathode BES (abioc-BES) showed lower PNP degradation efficiency of 31.7% ± 3.2–38.7% ± 7.1%. In the OC-abioc-BES, the PNP concentration was declined slightly (2.9% ± 1.1%) without detectable products. PNP concentration levelled off in the bioc-BES and was less than the environmental safety limit of 10 mg L−1 after 96 h (Fig. 2).3
 |
| Fig. 2 Degradation of PNP under different operating conditions in fed batch BESs. Error bars represent standard deviation (SD) based on three tests. The PNP removal rates (k) are: bioc-BES, R2 = 0.95288, k = 0.02978 ± 0.00339; bioc-BES-NAP, R2 = 0.96264, k = 0.00999 ± 7.44931 × 10−4; OC-bioc-BES: R2 = 0.99323, k = 0.00762 ± 2.29271 × 10−4; abioc-BES, R2 = 0.9374, k = 0.01455 ± 0.00154; the initial PNP concentration was 52.1 mg L−1. | |
UV-vis spectrum analyses showed that a peak at 400 nm of the influent shifted to two characteristic peaks at 231 and 297 nm of the effluent (Fig. 3a), suggesting that PNP degradation was present in the effluent. HPLC-MS analysis further demonstrated that p-aminophenol (PAP) was the dominant product of PNP in the bioc-BES. The m/z of degraded products matched the standard of PNP and PAP (molecular ion at m/z of 138.19 and 108.09, respectively) (Fig. 3b).
 |
| Fig. 3 (a) UV-vis spectra of the effluent and influent of bioc-BES and (b) HPLC-MS analysis of the metabolites of PNP. | |
Effect of initial concentrations on PNP degradation in BES
When the PNP concentration of the influent was under 30 mg L−1, the PNP concentration of the effluent was under 0.82 ± 0.4 mg L−1 in 48 h, suggesting that the bioc-BES system could promote PNP degradation and the final reductive product PAP formation (Fig. 4). With the increase of PNP concentration from 20 mg L−1 to 60 mg L−1, the PNP removal efficiency decreased from 98.2% ± 0.5%–63.9% ± 4.8% within 48 h, while the PAP formation in the effluent was from 14.14 ± 0.1 mg L−1 to 28.6 ± 2.9 mg L−1 (Fig. 4).
 |
| Fig. 4 Effect of initial PNP concentration on the performance of bioc-BES and error bars represent standard deviation (SD) based on three tests. | |
Microbial community structure in biocathode biofilms of BES
High throughput sequencing showed that 467 (bioc-BES), 596 (bioc-BES-NAP) and 562 (OC-bioc-BES) operational taxonomic units (OTUs) were obtained at the threshold of 97% from total reads of 32
296 (Table 1). Three communities of BESs had shared 67 OTUs (Fig. 5a), and bioc-BES possessed more unique OTUs of 42 (Fig. 5b). The results indicated that the microbial community of bioc-BES had slightly higher diversity (Shannon = 4.07) compared to communities in other BESs. The principal component analysis (PCA) and the heat map of OTUs showed a clear distinction between communities of different BES despite the fact that they shared the same inoculums (Fig. S1 and S2 in the ESI†).
Table 1 Operational taxonomic units (OTUs) and community diversity indices of different biocathode biofilms based on 454 pyrosequencing of 16S rRNA gene amplicons
Sample |
Chao |
Shannon |
Coverage |
Ace |
OTUs |
Reads |
Simpson |
Bioc-BES |
788 |
4.07 |
0.977196 |
893 |
467 |
8551 |
0.0636 |
Bioc-BES-NAP |
1042 |
3.73 |
0.976768 |
1354 |
596 |
11 880 |
0.0889 |
OC-bioc-BES |
1088 |
3.37 |
0.977244 |
1303 |
562 |
11 865 |
0.1188 |
 |
| Fig. 5 (a) The Venn diagram of genus and the overlap of the three bacterial communities from bioc-BES, bioc-BES-NAP and OC-bioc-BES and (b) the taxonomic identities of the shared OTUs at genus level. The “others” was the genera less than 1% of the total. | |
The majority of predominant phyla of the cathode biofilm in bioc-BES belonged to Proteobacteria (84%), while Proteobacteria (49.5%) and Bacteroidetes (36.02%) were the predominant phyla in bioc-BES-NAP. In contrast, the predominant phyla in OC-bioc-BES were Proteobacteria (40.13%), Acidobacteria (31.54%) and Bacteroidetes (16.17%) (Fig. 6). The microbial communities of cathode biofilms in BESs were different at class or subclass level. The communities were dominated by Betaproteobacteria (44.60%) and Alphaproteo-bacteria (25.26%) in bioc-BES, with Alphaproteobacteria (43.24%) and Sphingobacteria (23.21%) in bioc-BES-NAP, and Alphaproteobacteria (36.44%) and Acidobacteria (31.54%) in OC-bioc-BES.
 |
| Fig. 6 Relative abundance of predominant phyla and classes in the cathode biofilms of BESs. The “others” represents the phyla and class less than 1% of the total. | |
The predominant populations in biocathode biofilm of bioc-BES were affiliated with Delftia (28.86%), Diaphorobacter (9.4%), Aquamicrobium (7.1%), Raoultella (5.78%) and Shinella (5.55%), while the predominant populations in bioc-BES-NAP were Aminobacter (26.18%) and Chryseobacterim (11.94%), and Aminobacter (17.24%), Afipia (6.64%) and Sphingomonas (6.2%) in OC-bioc-BES (Fig. 7).
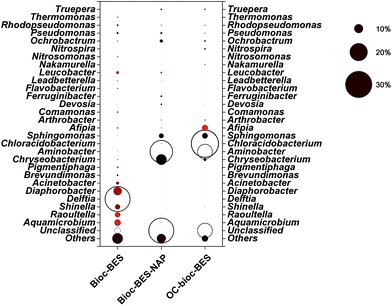 |
| Fig. 7 Relative abundance of predominant populations in the cathode biofilms of BESs. The “others” was the genera less than 1% of the total. | |
Discussion
Most previous investigations reported PNP removal in BES supplemented with the organic carbon source. Previous studies showed that the organic carbon source highly enhanced PNP reduction because it could function as the electron donor for reduction and the organic matter also made great biomass. In this study, sodium bicarbonate was used as the carbon source; the results demonstrated that the sole electron for PNP reduction was transferred from the cathodic electrode by electroactive bacterium. The PNP degradation efficiency (over 96%) in the two-chamber BESs fed with inorganic carbon source is similar to that obtained in the single- or two-chamber BESs fed with sodium acetate.33,34 Compared to an abiotic cathode BES (abioc-BES) and OC-bioc-BES, bioc-BES showed a higher PNP reduction efficiency, implying that microbial electrocatalysis on the cathode plays an important role in PNP degradation. It was found that a much higher cathodic current was generated in the biocathode than in the abiotic cathode,23 which indicated that cathodic biofilm catalyzed the bioelectrochemical PNP reduction.
High-throughput sequencing analysis showed that the microbial communities of biocathode biofilms differentiated between the bioc-BES, bioc-BES-NAP and OC-bioc-BES. Delftia and Diaphorobacter were abundant in the bioc-BES, but in low abundance (<0.1%) in the bioc-BES-NAP and the OC-bico-BES. Compared to a previous report, the predominant populations were affiliated with Myroides and Longilinea in the cathode biofilm of the single-chamber BESs fed with PNP.28 Recent investigations proved that Delftia had the capability of soluble gold reduction and extracellular electron transfer.35 Diaphorobacter was the second most abundant genus in the biocathode biofilm of bioc-BES but in low abundance in bioc-BES-NAP and OC-bioc-BES, which dominated in the early stages of biofilm formation,36 and possibly transferred an electron in the denitrification reaction.37 Raoultella was created in 2001 from the genus Klebsiella,38 isolated from the anodic biofilm of MFC using glucose as substrate,39 which can be an electron shuttle. These three genera may be affiliated with putative electroactive bacteria that reduce PNP degradation using electrons from the cathode.
In the bioc-BES-NAP and OC-bioc-BES the communities had more than 10% PNP-degrading bacteria (Pseudomonas, Ochrobactrum, Arthrobacter, Flavobacterium and Sphingomonas), suggesting that the main factor of PNP removal was the PNP-degrading bacteria. The key factor of the highest PNP degradation in bioc-BES than in the others performed is the interactive effect of the applied voltage and the bacteria. As is shown in Fig. 6, there are different community compositions on the cathode surfaces of the three BESs. The PNP degraders were heavily enriched in the bioc-BES-NAP and the OC-bioc-BES, but the difference in community composition was so significant that cannot be distinguished in the PNP degradation efficiency.
454 Pyrosequencing analyses of 16S rRNA gene amplicons indicated that applied voltage affected bacterial community's composition on the cathode. These results showed that the bacteria selectively enriched on the BES biocathode and the different reactors had a prominent effect on the community structures. Our results revealed that the applied voltage can enhance the enrichment of both the electrochemically active bacteria and the anaerobic bacteria, and can also establish the electron flows through the direct and indirect stimulation of the microbial metabolism.40 The applied voltage supported the bioc-BES microbial growth and activity. Our results suggest that the cooperation of electrocatalysis and electrochemically active bacteria on the cathodic electrode accelerates PNP degradation in the biocathode BES.
Conclusions
This study indicated that p-nitrophenol (PNP) degradation was implied using a biocathode BES with sodium bicarbonate. Results demonstrated PNP degradation and PAP formation; within 48 h, 74% ± 5.7% of PNP was removed. The biocathode microbial communities analysed by 454 pyrosequencing showed that Proteobacteria was the dominant phylum in bioc-the BES, bioc-BES-NAP and OC-bioc-BES, whereas Acidobacteria was abundant only in the OC-bioc-BES. The dominant populations in bioc-BES were Delftia and Diaphorobacter. Aminobacter had higher abundance in the OC-bioc-BES and bioc-BES-NAP. The applied voltage plays an important role in enhancing PNP degradation and shaping of the microbial community. The biocathode BES system is proven to show great promise as a treatment for PNP degradation and water reuse, and may be seen as a new treatment for recalcitrant nitroaromatic compounds.
Acknowledgements
This study was supported by National Natural Science Foundation of China (Nos. 31270004, 51422805), the Science Fund for Distinguished Young Scholars of Heilongjiang Province (Grant No. JC201407), the Fundamental Research Funds for the Central Universities (No. HIT.BRETIV. 201319), the State Key Laboratory of Urban Water Resource and Environment (Harbin Institute of Technology) (No. 2013DX01), and National High-Tech R & D Program of China (863 Program) (No. 2011AA060905).
References
- V. Uberoi and S. K. Bhattacharya, Water Environ. Res., 1997, 69, 146–156 CrossRef CAS.
- X. Zhu and J. Ni, Electrochem. Commun., 2009, 11, 274–277 CrossRef CAS.
- H. Liu, T. J. Hu, G. M. Zeng, X. Z. Yuan, J. J. Wu, Y. Shen and L. Yin, Int. Biodeterior. Biodegrad., 2013, 76, 108–111 CrossRef CAS.
- T. Bruning, C. Chronz, R. Thier, J. Havelka, Y. Ko and H. M. Bolt, Occup. Environ. Med., 1999, 41, 144–149 CrossRef CAS.
- Z. I. Bhatti, H. Toda and K. Furukawa, Water Res., 2002, 36, 1135–1142 CrossRef CAS PubMed.
- T. Hirooka, H. Nagase, K. Hirata and K. Miyamoto, Biochem. Eng. J., 2006, 29, 157–162 CrossRef CAS.
- B. A. Donlon, E. Razoflores, J. A. Field and G. Lettinga, Appl. Environ. Microbiol., 1995, 61, 3889–3893 CAS.
- A. J. Wang, H. Y. Cheng, B. Liang, N. Q. Ren, D. Cui, N. Lin, B. H. Kim and K. Rabaey, Environ. Sci. Technol., 2011, 45, 10186–10193 CrossRef CAS PubMed.
- M. Sun, D. D. Reible, G. V. Lowry and K. B. Gregory, Environ. Sci. Technol., 2012, 46, 6174–6181 CrossRef CAS PubMed.
- B. A. Donlon, E. Razo-Flores, G. Lettinga and J. A. Field, Biotechnol. Bioeng., 1996, 51, 439–449 CrossRef CAS PubMed.
- H. Wang and Z. J. Ren, Biotechnol. Adv., 2013, 31, 1796–1807 CrossRef CAS PubMed.
- J. Sun, Z. Bi, B. Hou, Y. Q. Cao and Y. Y. Hu, Water Res., 2011, 45, 283–291 CrossRef CAS PubMed.
- J. Zhang, Y. Zhang, X. Quan, Y. Li, S. Chen, H. Zhao and D. Wang, Biochem. Eng. J., 2012, 63, 31–37 CrossRef CAS.
- F. Kong, A. Wang and H. Y. Ren, Bioresour. Technol., 2015, 175, 624–628 CrossRef CAS PubMed.
- S. M. Strycharz, S. M. Gannon, A. R. Boles, A. E. Franks, K. P. Nevin and D. R. Lovley, Environ. Microbiol. Rep., 2010, 2, 289–294 CrossRef CAS PubMed.
- L. Huang, X. Chai, X. Quan, B. E. Logan and G. Chen, Bioresour. Technol., 2012, 111, 167–174 CrossRef CAS PubMed.
- F. Kong, A. Wang, H. Y. Ren, L. Huang, M. Xu and H. Tao, Bioresour. Technol., 2014, 158, 32–38 CrossRef CAS PubMed.
- F. Aulenta, L. Tocca, R. Verdini, P. Reale and M. Majone, Environ. Sci. Technol., 2011, 45, 8444–8451 CrossRef CAS PubMed.
- S. T. Lohner, D. Becker, K. M. Mangold and A. Tiehm, Environ. Sci. Technol., 2011, 45, 6491–6497 CrossRef CAS PubMed.
- L. Huang, X. Chai, G. Chen and B. E. Logan, Environ. Sci. Technol., 2011, 45, 5025–5031 CrossRef CAS PubMed.
- K. B. Gregory and D. R. Lovley, Environ. Sci. Technol., 2005, 39, 8943–8947 CrossRef CAS PubMed.
- J. C. Thrash, J. I. Van Trump, K. A. Weber, E. Miller, L. A. Achenbach and J. D. Coates, Environ. Sci. Technol., 2007, 41, 1740–1746 CrossRef CAS PubMed.
- B. Liang, H. Y. Cheng, D. Y. Kong, S. H. Gao, F. Sun, D. Cui, F. Y. Kong, A. J. Zhou, W. Z. Liu, N. Q. Ren, W. M. Wu, A. J. Wang and D. J. Lee, Environ. Sci. Technol., 2013, 47, 5353–5361 CrossRef CAS PubMed.
- D. Shen, X. Zhang, H. Feng, K. Zhang, K. Wang, Y. Long, M. Wang and Y. Wang, Bioresour. Technol., 2014, 172, 104–111 CrossRef CAS PubMed.
- J. Shen, X. Xu, X. Jiang, C. Hua, L. Zhang, X. Sun, J. Li, Y. Mu and L. Wang, Water Res., 2014, 67, 11–18 CrossRef CAS PubMed.
- H. C. Tao, X. Y. Wei, L. J. Zhang, T. Lei and N. Xu, J. Hazard. Mater., 2013, 254, 236–241 CrossRef PubMed.
- Z. He and L. T. Angenent, Electroanalysis, 2006, 18, 2009–2015 CrossRef CAS.
- X. Jiang, J. Shen, S. Lou, Y. Mu, N. Wang, W. Han, X. Sun, J. Li and L. Wang, Bioresour. Technol., 2016, 216, 645–652 CrossRef CAS PubMed.
- L. Lu, N. Ren, X. Zhao, H. Wang, D. Wu and D. Xing, Energy Environ. Sci., 2011, 4, 1329–1336 CAS.
- D. R. Lovely and E. J. P. Phillips, Appl. Environ. Microbiol., 1988, 54, 1472–1480 Search PubMed.
- L. Lu, D. Xing and N. Ren, Water Res., 2012, 46, 2425–2434 CrossRef CAS PubMed.
- J. Jia, Y. Tang, B. Liu, D. Wu, N. Ren and D. Xing, Bioresour. Technol., 2013, 144, 94–99 CrossRef CAS PubMed.
- S. Lou, X. Jiang, D. Chen, J. Shen, W. Han, X. Sun, J. Li and L. Wang, RSC Adv., 2015, 5, 27052–27059 RSC.
- J. Shen, Y. Zhang, X. Xu, C. Hua, X. Sun, J. Li, Y. Mu and L. Wang, Water Res., 2013, 47, 5511–5519 CrossRef CAS PubMed.
- Y. Jangir, S. French, L. M. Momper, D. P. Moser, J. P. Amend and M. Y. El-Naggar, Front. Microbiol., 2016, 7, 756 Search PubMed.
- R. P. Revetta, V. Gomez-Alvarez, T. L. Gerke, C. Curioso, J. W. Santo Domingo and N. J. Ashbolt, FEMS Microbiol. Ecol., 2013, 86, 404–414 CrossRef CAS PubMed.
- S. S. Chakravarthy, S. Pande, A. Kapoor and A. S. Nerurkar, Appl. Biochem. Biotechnol., 2011, 165, 260–269 CrossRef CAS PubMed.
- M. Drancourt, C. Bollet, A. Carta and P. Rousselier, Int. J. Syst. Evol. Microbiol., 2001, 51, 925–932 CrossRef CAS PubMed.
- Z. Kimura, K. M. Chung, H. Itoh, A. Hiraishi and S. Okabe, Int. J. Syst. Evol. Microbiol., 2014, 64, 1384–1388 CrossRef CAS PubMed.
- C. L. Chun, R. B. Payne, K. R. Sowers and H. D. May, Water Res., 2013, 47, 141–152 CrossRef CAS PubMed.
Footnote |
† Electronic supplementary information (ESI) available. See DOI: 10.1039/c6ra17446a |
|
This journal is © The Royal Society of Chemistry 2016 |