DOI:
10.1039/C6RA16066B
(Paper)
RSC Adv., 2016,
6, 85763-85772
Recombinant sFRP4 bound chitosan–alginate composite nanoparticles embedded with silver nanoclusters for Wnt/β-catenin targeting in cancer theranostics†
Received
21st June 2016
, Accepted 1st September 2016
First published on 1st September 2016
Abstract
Targeting a specific pathway aberrantly upregulated in cancer cells has shown immense potential in cancer therapy. Although specific signaling pathways have been previously targeted for cancer therapy, the Wnt pathway has been rarely explored in this pursuit. Also an important facet of cancer theranostics is cellular bio-imaging. Taking into consideration these two critical aspects, we herein report the synthesis, bio-imaging and anti-cell proliferative effect of the recombinant secreted frizzled-related protein-4 (sFRP4) bound with silver nanocluster-embedded chitosan–alginate composite nanoparticles. While bacterially expressed and purified recombinant sFRP4 specifically blocked the aberrant Wnt/β-catenin signaling in cancer cells, the remarkable luminescence of the silver nanoclusters enabled imaging, binding, and uptake studies. Both the protein as well as the nanoparticle component of this multifaceted system were responsible for triggering facile cell death; sFRP4 by inhibiting the Wnt signaling, and silver nanoclusters by the activation of reactive oxygen species. Hereby, a co-therapy module was generated that successfully brought about apoptotic cell death. Therefore, the current module has achieved significant benefits for in vitro cancer theranostics.
Introduction
Major headway in the fields of biology, nanotechnology and electronics has culminated in the emergence of ‘theranostics’ – having potential to facilitate medical diagnosis and therapy. The advent of theranostics has a positive impact on cancer treatment, where various approaches of probing, detection and delivery of chemotherapeutic drugs have been witnessed in recent years. However, targeting specific signaling pathways with recombinant protein-functionalized nanoparticles (NPs) has seldom been accomplished in cancer therapy. Although using recombinant proteins as a signaling modulator presents a challenge in itself; however, their immune acceptance and ability to regulate specific pathways help to surmount the major problem of adverse side-effects of conventional drugs.1
Active targeting has been achieved by specific recognition between antigen–antibody2 in order to inhibit molecular pathways aberrant in cancer, such as HER2, MAPK, Akt, EGF, p53.3,4 In addition, cell surface receptors overexpressed in cancer cells, such as transferrin receptors and folate receptors, have been exploited for targeted delivery of a particular gene, siRNA or drugs.5–8 While delivery of a therapeutic protein with nanocarriers has been deemed prudent,9 the interaction of therapeutic protein with its corresponding cell surface receptor has also been exploited for delivery of the cytotoxic composite nanoparticles.10
One pathway that has rarely been explored for targeting cancer cells is the Wnt pathway.11 This signaling pathway is aberrantly upregulated in most cancer types,12 resulting in the activation of downstream pro-proliferative genes13–15 via stabilization of β-catenin,16 which is a critical molecule central to the Wnt pathway. In non-cancerous cells, it is antagonized by a family of five glycoproteins, called the secreted frizzled-related proteins (sFRPs), which are silenced in cancer cells due to promoter hypermethylation.17 Of the five known sFRPs, sFRP4 is the most recently discovered one that has exhibited tremendous promise in preliminary investigations. Transfection of sFRP4 gene into prostate cancer18 and ovarian cancer19 cells has shown the anti-tumor effect and chemosensitizing property of sFRP4. Exogenous administration of sFRP4 onto keratinocytes has also been reported to demonstrate positive results.20 Furthermore, sFRP4 has been found to chemosensitize glioma stem cells towards conventional chemotherapeutic drugs.21 Although the use of sFRPs for inhibiting the Wnt pathway has a long way to traverse before reaching the clinical stage, certain chemical and biological moieties have been employed for blocking of this pathway in vivo, in independent studies. A chemical inhibitor of cyclooxygenase-2, celecoxib, has been used in combination therapy with a chemotherapeutic drug; the combination has been found to reduce tumor growth by 57% via suppression of Wnt pathway in vivo.22 In another study, a monoclonal antibody, designed to target multiple frizzled receptors, inhibited the growth of various tumor types by blocking the canonical Wnt signaling in human tumor xenograft models.23 However, the effect of recombinant sFRP4 on cancer cells, especially if purified from non-human sources, needs to be further delved into. Moreover, exogenous administration of a protein may involve certain disadvantages, such as rapid loss of protein function, reduced half-life, and changes in protein structure. To circumvent these limitations and to enhance the efficacy of the treatment module, composite NPs could be loaded with the therapeutic protein.
Equipped with the promising reports, we proceeded to examine the co-therapeutic value of recombinant sFRP4 decorated silver nanocluster embedded chitosan-nanoparticles (Ag NC–Chi–Alg NPs). These multifunctional NPs could be designed using the components working in tandem, to target the Wnt pathway and specifically inhibit cancer cells. A visual representation of the concept portrays the crux of this investigation in Fig. 1.
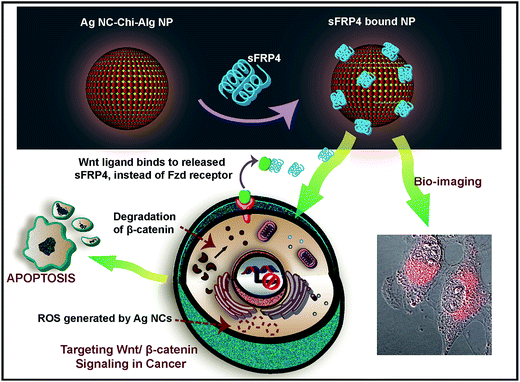 |
| Fig. 1 The essence of this study is portrayed in this schematic. | |
The composite NPs were synthesized by a novel method, to accommodate the size and surface charge requirements of this study. For binding, imaging, and uptake analyses, the extraordinary photoluminescence of Ag NCs was conveniently utilized. Although both gold (Au) and silver (Ag) NCs have attracted tremendous attention of late, Ag NCs hold the added advantage of being an anti-cell proliferative agent. At low doses, silver has been established to exert minimum toxic effects, as evidenced by its applicability in the biological system.24 Ag NCs have displayed immense potential in theranostics by virtue of their unique photophysical properties, arising due to their quantum confinement. Moreover, in this study, the interaction of sFRP4 with Wnt ligands would be expected to facilitate the uptake of the Ag NC–Chi–Alg NPs; thus the cytotoxicity of the Ag NCs would be expected to affect the cancer cells, which express Wnt ligands, more than the non-cancerous cells. Furthermore, as the moiety responsible for targeting, that is sFRP4, can play the dual role of specifically inhibiting cancer cells, the composite would possess superior effectuality.
Experimental section
Chemicals
All items were purchased from Sigma-Aldrich, unless mentioned otherwise.
Synthesis of Chi–Ag NC–Alg NPs
Ag NCs were engineered by slightly modifying the previous report,25 whereas, the composite NPs were formulated by a novel method of synthesis. Briefly, 200 μl of 0.5% chitosan was dissolved in 2 ml Milli-Q water, to which 5.26 mg of lipoic acid and 5 mg of sodium borohydride were added. The solution was stirred for 30 min, by the end of which, the solution turned brown and the luminescence was observed under UV irradiation. Then 100 μl of 1% sodium alginate was added and pH was adjusted to 7.4. Finally synthesized NPs were centrifuged to remove free clusters.
Expression and purification of GST tagged sFRP4
The cloning, expression, and purification of human sFRP4 have been done following the methods detailed in our previous work.26 Recombinant glutathione S-transferase (GST) tagged protein was purified by affinity chromatography; purified GST-sFRP4 was dialyzed against 10 mM Tris (pH 7.4) and quantified by Bradford assay.
Characterization of Chi–Ag NC–Alg NPs
The Chi–Ag NC–Alg NPs were characterized using fluorescence spectrophotometer (Fluoromax-4) and UV-vis spectrophotometer (Perkin Elmer, Lambda 750). Particle size distribution and surface charge analyses were performed by dynamic light scattering and zeta potential measurements, respectively, using Malvern Zetasizer Nano ZS. MALDI-TOF analysis was done to ascertain the number of silver atoms constituting a single cluster (Bruker). Quantum yield was calculated by an established one-step method.27 For imaging the NPs and sFRP4 bound NPs (henceforth referred to as NPs-sFRP4), samples were drop-casted on carbon-coated copper grids and analyzed with TEM (JEM 2100) at an accelerating voltage of 200 kV. Quantum yield of the Ag NC–Chi–Alg NPs was quantified with the following formula:
here, Q stands for quantum yield, I denotes integrated fluorescence intensity, OD connotes optical density (absorption), n is the refractive index, and R represents fluorescein, which is the reference dye used here.
Binding and release studies of NPs with GST-sFRP4
Binding of fixed amount of NPs and increasing concentrations of sFRP4 was done for 1 h at 37 °C. At the end of it, samples were centrifuged and pellet was redispersed in water. Luminescence of NPs was probed using fluorescence spectrophotometer LS55 Perkin-Elmer, at excitation and emission wavelengths of 425 nm and 650 nm, respectively. Binding percentage was calculated by the formula:
Release profile of sFRP4 from NPs was generated after selecting the concentration of protein at which binding saturation was attained. After the binding of the sFRP4 to the NPs in Tris–HCl buffer for 1 h at 37 °C as before, it was centrifuged to eliminate the unbound sFRP4. The pellet was then redispersed in PBS buffer (pH 7.4) and centrifuged once again to collect the supernatant containing the released protein. The intrinsic fluorescence of the protein thus released in PBS was measured over a time span of 48 h at an emission wavelength of 360 nm, on excitation with 280 nm wavelength of light.
Mammalian cell culture
Human cervical carcinoma cell line (HeLa) was obtained from National Centre for Cell Sciences, India. Cells were cultured in Dulbecco's Modified Eagle's Medium (DMEM), supplemented with 10% FBS, 100 units per ml penicillin, 100 μg ml−1 streptomycin in a 5% carbon dioxide incubator under humid conditions at 37 °C.
Cytotoxicity assays
The effect of sFRP4-NPs on viability of HeLa cells was gauged by trypan blue and MTT (3-(4,5-dimethylthiazol-2-yl)-2,5-diphenyltetrazolium bromide) assays. Cells were seeded at a density of 7 × 104 cells per well in 12-well plate for trypan blue assay and at 7000 cells per well in 96-well plates for MTT assay. Cells were allowed to attach overnight, after which sFRP4, NPs, and NP-sFRP4 were added separately to the cells in serum-free media, in triplicates. NP-sFRP4 were added at concentrations ranging from 0.1–0.75 mg ml−1, while corresponding concentrations of sFRP4 and NPs were also added separately, for comparison.
Trypan blue assay is based on the concept that the trypan blue dye is membrane impermeable; hence, only the cells with damaged membrane would take up the stain, while healthy cells would remain unstained. For this purpose control and treated cells were harvested after the treatment period and mixed in equal volume with 0.4% trypan blue (Invitrogen). This was loaded on a counting chamber and mounted on a Countess-automated cell counter (Invitrogen), which calculated the percentage of live cells.
MTT assay was also performed to determine cell viability, in which MTT (HiMedia, India) was converted to purple formazan crystals soluble in dimethyl sulfoxide (DMSO) by live cells. Multiplate reader (Tecan) was used to measure the absorbance at 550 nm and the background at 655 nm was subtracted. The following formula was used to ascertain the percentage of cell viability:
It should be mentioned here, that while MTT assay gives percentage viability of each treated sample with respect to the untreated control cells, trypan blue provides percentage of live cells in each sample with respect to number of dead cells. Statistical significance was evaluated for both assays.
Estimation of cellular uptake of NPs
To examine the uptake of luminescent Ag NC embedded NPs by the HeLa cells, confocal microscope (LSM 880, Zeiss) was used. Cells were treated with NPs for 2 h and 8 h, before imaging was done. Further, flow cytometric evaluation was done, for which cells were treated with NPs for varying time periods ranging from 0 h to 48 h. On excitation with 488 nm laser of Fluorescence Activated Cell Sorter (FACSCalibur, BD Biosciences, USA), red luminescence of the NPs uptaken by cells was detected in the FL3 channel.
Western blotting for confirmation of affected signaling pathway
To confirm whether the recombinant sFRP4 was functional and effectively modulated the Wnt pathway as intended, the expression of a central molecule of the canonical Wnt signaling was probed by western blotting. Detection of total β-catenin protein as well as its phosphorylated form was done by respective antibodies. For this purpose, HeLa cells were treated for 24 h with sFRP4, NPs, and NP-sFRP4, respectively. Whole cell lysate containing total cytoplasmic protein was prepared with RIPA buffer (with protease inhibitors) and quantified by Lowry's protein estimation assay. 50 μg of each sample was loaded in a 12% SDS-PAGE (sodium dodecyl sulfate-polyacrylamide gel electrophoresis) and electrophoresed. Thereafter, the total protein was transferred onto an activated PVDF (polyvinylidene difluoride) membrane. Next, the membrane was blocked with BSA (bovine serum albumin, HiMedia, India), after which it was incubated overnight at 4 °C with a primary antibody – β-catenin or phospho (pSer33/pSer37)-β-catenin or endogenous control β-actin. Then the blot was washed five times with PBST (PBS with 1% Tween-20) or TBST (Tris buffered saline with 1% Tween-20) and incubated with a corresponding secondary antibody conjugated with HRP (horseradish peroxidase). Again it was washed five times, before being developed with Chemiluminescent Peroxidase Substrate kit, according to manufacturer's protocol. It should be noted that TBS buffer was used in case of phospho-β-catenin only.
Studying the mode of cell death
To delineate the mode of cell death, FACS-based assays, viz., determination of ROS generation, effect on cell cycle, and annexin V-FITC/PI apoptosis detection were performed. In addition, apoptosis was also deciphered by microscopy-based acridine orange (AO)/ethidium bromide (EB) dual staining and FESEM (Sigma, Zeiss) images.
ROS generation
For determination of reactive oxygen species (ROS) generation, the HeLa cells were treated separately with sFRP4, NPs, and NP-sFRP4 for 4 h. Subsequently, the cells were stained with dichlorofluorescein diacetate (DCFDA) and analyzed by flow cytometry in the FL1 channel.
Cell cycle analysis
For this experiment, the HeLa cells were seeded at a density of 1 × 105 cells per well in a 6-well plate. Cells were incubated with 0.75 mg ml−1 NP-sFRP4, along with corresponding concentrations of sFRP4 and NPs. After 48 h of treatment, the cells were harvested by trypsinization and fixed by adding chilled 70% ethanol dropwise while vortexing. After the fixation step of at least 1 h, the cells were centrifuged to wash off the ethanol. Then the pellet was redispersed in 10 mM PBS, supplemented with 0.4 mg ml−1 DNase-free RNase for 1 h at 37 °C. Thereafter, each sample was incubated with PI at a final concentration of 10 μg ml−1 for 15 min in dark, after which samples were analyzed in the FL2 channel of a flow cytometer (BD FACSCalibur).
Annexin V-FITC/PI apoptosis detection assay
Annexin V-fluorescein isothiocyanate (FITC)/propidium iodide (PI) apoptosis detection kit (BD Biosciences) was used to discriminate between healthy and apoptotic cells. While annexin V binds to phosphatidylserine sites exposed upon membrane flipping, one of the earliest hallmarks of apoptosis, PI can only permeate through membrane compromised cells. Therefore, healthy cells are negative for both FITC (tagged to annexin V) and PI, early apoptotic cells yet to undergo membrane damage are positive for FITC and negative for PI, late apoptotic cells are positive for both, whereas cells stained with only PI represent the very late apoptotic or necrotic population. In this pursuit, the cells were seeded and treated in a manner same as above. The assay was performed according to the manufacturer's protocol.
Dual staining with acridine orange (AO)/ethidium bromide (EB)
AO and EB double staining was done to corroborate on the results acquired in the above experiment. While AO is membrane permeable and can stain the entire cell, PI is membrane impermeable and stains the DNA of damaged cells only. Thus double stained cells denote the apoptotic population, whereas only PI stained cells simply represent cell death. For this experiment, cells were treated in a 96-well plate for 48 h. Then the media was discarded and replaced with PBS containing 2 μg ml−1 AO and 10 μg ml−1 EB in dark. After 5 min, cells were washed with fresh PBS and imaged with an epi-fluorescence microscope (Nikon ECLIPSE TS100).
FESEM analysis
For capturing the image of apoptotic cells, Field Emission Scanning Electron Microscopy (FESEM) analysis was done. To avoid complete disintegration of cellular structure, treatment of the cells with composite NPs was done for only 24 h. The control and treated cells were harvested and fixed with chilled 70% ethanol, following which, they were drop-casted on an aluminium foil wrapped piece of glass slide and allowed to dry. After double coating of the sample with gold film, cells were imaged with FESEM (Sigma, Zeiss).
Results and discussion
Expression and purification of functional GST-sFRP4
GST tagged sFRP4 was expressed in E. coli BL21(DE3) harboring a clone generated in our laboratory. Recombinant sFRP4 was purified using glutathione agarose affinity chromatography (Fig. 2A). Since the key to the therapeutic application of any recombinant human protein expressed in bacterial system is its functionality, we focused on retaining its functionality by ensuring the proper folding of the GST-sFRP4 by gradient dialysis against Tris–HCl (pH 7.4). It should be mentioned here that the activity of sFRP4 is known to be independent of post-translational modifications.28,29 Confirmation of the proper functioning of the recombinant protein was proven by cell based assays and the western blotting experiments described later.
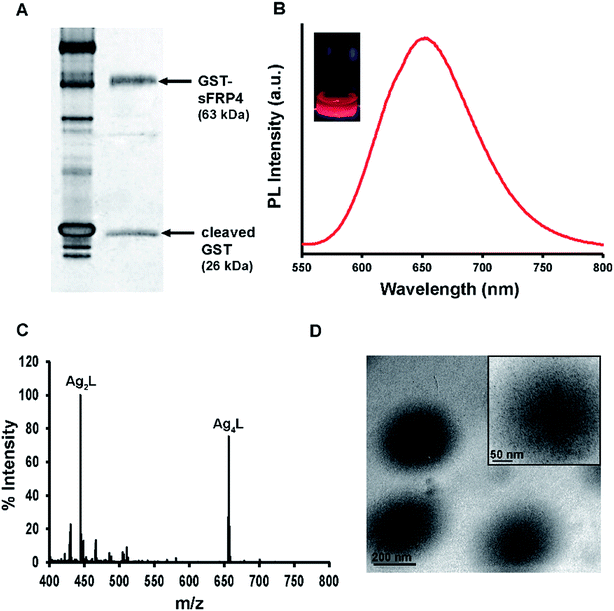 |
| Fig. 2 (A) Discrete single band of GST-sFRP4 corresponding to its legitimate size of 63 kDa was observed in 12% sodium dodecyl sulfate polyacrylamide gel electrophoresis (SDS-PAGE), a cleaved band of GST was also noted. (B) Emission spectrum of Ag NC–Chi–Alg NPs connotes red luminescence (650 nm), when excited with 425 nm wavelength of light. (C) MALDI-TOF spectra depicted few-atom clusters of silver, with two-atom and four-atom cluster formation being most prominent; L denotes the ligand-lipoic acid. (D) TEM images prove conclusively, the formation of nearly uniform spherical NPs of 350–400 nm in diameter; inset shows magnified NP, with distinct Ag NCs. | |
Binding of sFRP4 with Ag NC–chitosan–alginate NPs based on electrostatic interactions
Since the GST-sFRP4 was found to possess an overall positive charge at physiological pH 7.4, as calculated with ExPASy pI calculator, the AgNC–Chi–Alg NPs were tailored to bind to sFRP4 by endowing a net negative charge upon them using the biopolymer alginate, forming the NPs. Zeta potential studies (ESI Fig. S1†) revealed the net surface charge of the as-synthesized NPs to be −27 mV. As sFRP4 affects the Wnt by binding to the extracellular Wnt ligands, sFRP4 was bound onto the surface of the NPs, instead of being encapsulated within. Moreover, to allow the extracellular release of the protein before the uptake of the luminescent NPs, we attempted to promote a slow uptake of the NPs. Hence, in addition to conferring negative charge upon the NPs, their size was also kept relatively large, as illustrated by a hydrodynamic diameter of 774 nm (ESI Fig. S2†).30 Our group had previously reported Chi–Au NC (gold NCs)–Alg NPs maneuvered to bind to sFRP1, a different isoform of sFRP superfamily, for theranostic applications.31
Characterization of Ag NC–Chi–Alg NPs
The so-synthesized Ag NC–Chi–Alg NPs displayed characteristic red luminescence emission spectrum of Ag NCs at 650 nm, when excited by a wavelength of 425 nm (Fig. 2B). The intense photoluminescence was evidenced by reasonably higher quantum yield (11.4%) than many reported Ag NC based composites, making this composite appropriate for theranostic applications.25,32,33 The mass spectra obtained with MALDI-TOF revealed prominent peaks at m/z equal to 444 and 656 (Fig. 2C), corresponding to the complexes [Ag2L + Na+ + H+]+ and [Ag4L + Na+ − 2H+]−, respectively, where L denotes lipoic acid. Formation of the Ag4 cluster is in agreement with a previous investigation, where lipoic acid was used as template for synthesis.25 However, Ag2 complex has also been reported for red emitting Ag NCs.32 Additionally, the UV-vis absorption spectra showed the minor peaks at 334 nm and 432 nm (ESI Fig. S3†), possibly due to the formation of extremely small silver nanoparticles.25
Transmission electron microscopy (TEM) images depicted the formation of spherical NPs with diameters in the range of 350–400 nm (Fig. 2D). The size difference observed between TEM and DLS data was possibly due to swelling of alginate in aqueous environment.34 Notably, the presence of Ag NCs could be distinctly distinguished for both NPs and sFRP4 bound NPs (henceforth referred to as NPs-sFRP4) samples in the magnified TEM images (inset of Fig. 2D and ESI Fig. S4,† respectively). Interestingly, binding of GST-sFRP4 to the NPs did not alter their spherical structure. The maximum binding of 71% was recorded by probing the luminescence of Ag NCs (Fig. 3A). Maintaining the same ratio of protein and NPs, this binding efficiency was reaffirmed by probing the intrinsic fluorescence of protein as well (data not shown). Fluorescence of sFRP4 was also exploited to track its release from the NPs over 48 h, where 65% of the bound protein was released immediately in PBS, which increased gradually upto 96% in 48 h (ESI Fig. S5†). This initial release may be attributed to the change in buffer from Tris–HCl, in which binding was done, to PBS, in which release of the protein from the composite was studied. This trend of burst release at initial time points is consistent with previous studies on protein bound composite nanoparticles.9
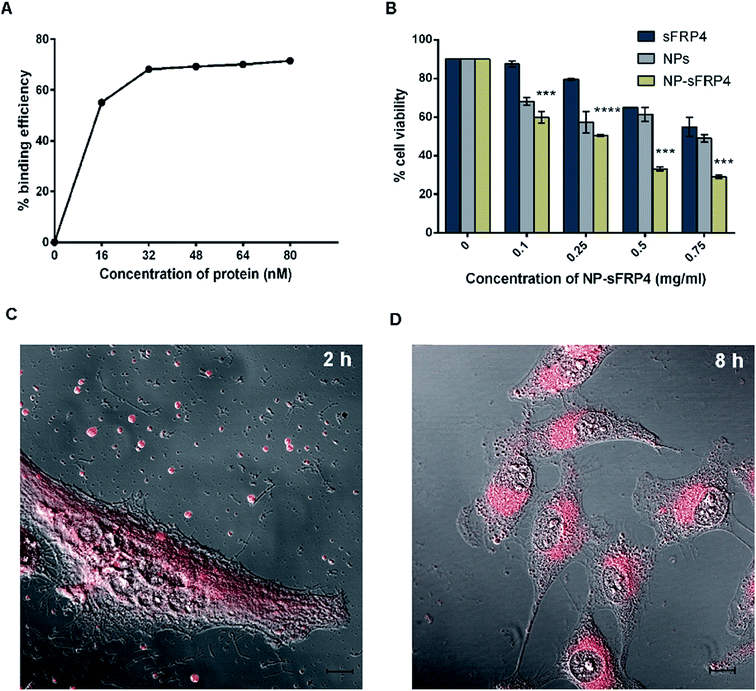 |
| Fig. 3 (A) Binding efficiency was calculated by incubating fixed concentration of NPs with varying concentrations of sFRP4 for 1 h, maximum binding of 71% was obtained, after which saturation was attained. (B) Trypan blue dye exclusion assay demonstrated that NP-sFRP4 was more potent in action than either component individually, as depicted by the percentage of viable cells, with the significance being between NP-sFRP4 and sFRP4 treated cells (‘***’ denotes p value < 0.001 and ‘****’ denotes p < 0.0001). (C and D) Images acquired with confocal microscope showed that in 2 h, luminescent NPs were partially uptaken, with many NPs in the media or on cell surface; in 8 h, almost complete cellular uptake of NPs was observed. Scale bar represents 10 μm. The images represent the luminescence of the as-synthesized Ag NCs. | |
Therapeutic efficacy determined by cell viability assays
The therapeutic impact of the NP-sFRP4 was tested on cervical cancer cells (HeLa), which was the primary objective of this work. Although sFRP4 transfection has been reported to exert anti-proliferative effect,35 it is a challenge to ensure that a bacterially expressed human protein retains its functionality. Initial experiments were done to assess viability of treated cells by trypan blue dye exclusion36 (Fig. 3B) and MTT ((3-(4,5-dimethylthiazol-2-yl)-2,5-diphenyltetrazolium bromide, ESI Fig. S6†)) assays and to confirm the functionality of the purified GST-sFRP4. Further, cytotoxicity of the NPs bestowed by the presence of Ag NCs37 was also evident. More importantly, trypan blue assay demonstrated the cumulative effects of sFRP4 and NPs for treatment with sFRP4 bound NPs. At the highest concentration of treatment, viability was significantly lower for NP-sFRP4 (29%), than sFRP4 (55%) or NPs (49%). While 0.75 mg ml−1 of NPs (containing 22.8 μg ml−1 of silver) was required to reach the IC50 value, only 0.25 mg ml−1 NP-sFRP4 (containing only 7.6 μg ml−1 silver) successfully brought about the same effect.38 These data implied the development of a successful co-therapeutic regime.
Cellular uptake of luminescent NPs assessed by confocal microscopy and flow cytometry
The cytotoxic effects of the Ag NC embedded NPs attributed to their gradual uptake by the HeLa cells, as divulged by simultaneous confocal microscopy and flow cytometric analysis (ESI Fig. S7†). The images acquired using confocal microscope illustrated that after 2 h, luminescent NPs were in the process of being uptaken, with NPs prominently visible outside the cells as well (Fig. 3C). Z-Stacking of this image clearly divulged that NPs remained in the media and on the surface of the cell (ESI Fig. S8†), indicating that the active uptake of the NPs was continuing even after 2 h. However in 8 h, the luminescent NPs were almost completely uptaken by the cells (Fig. 3D). From this experiment, we may surmise that the gradual uptake of the NPs would ensure that there was sufficient time for the release of the protein outside the cells, before the NPs were uptaken and Ag NCs began to exert their activity on the cells. The observation was concurrent with the FACS-based quantitative analysis, which connote cellular uptake of NPs over a period of several hours, which is desirable for the release of sFRP4 outside the cells, where it is functional. However, the clusters appear to have been degraded in 48 h, evinced by the lack of luminescence in the cells at the time. Hence, the bright luminescence of these Ag NCs makes them suitable for probing and imaging in biological systems.
Targeting the Wnt/β-catenin signaling by recombinant sFRP4
As sFRPs are known to block the Wnt pathway in non-cancerous cells, the same effect would be expected in cancer cells if sFRP expression was induced in any manner. With this intent, the mechanism behind the anti-proliferative activity of recombinant sFRP4 was elucidated by examining the downstream Wnt signal cascade. Western blotting experiments were performed to probe the expression of β-catenin, a central molecule of the Wnt signal cascade (Fig. 4A). The sFRP4-treated and NP-sFRP4-treated HeLa cells exhibited a 1.4-fold and 1.67-fold reduction in expression of total β-catenin protein, respectively, denoting inhibition of the downstream Wnt signaling.39 Furthermore, an upregulation in the phosphorylated form of β-catenin in treated cells signified proteasome mediated degradation of β-catenin.40 However, this phosphorylated form was not observed in cells treated with NP-sFRP4, due to potent action of the Ag NCs, which could also initiate apoptosis, where transient expression of phosphorylated β-catenin could not be detected. The NP-sFRP4 treatment showed greater cell apoptosis through β-catenin via Wnt signaling pathway as well as by known anti-cancer effect of Ag NCs present in Ag NC–chitosan–alginate NPs. Quantitative representations of fold-change of β-catenin and phospho-β-catenin as obtained by the ImageJ software have been provided in the ESI Fig. S9A and B,† respectively. These results provide categorical evidence in favor of the role of the GST-sFRP4 in antagonizing the Wnt/β-catenin pathway. Hence we may attribute the anti-proliferative effect of sFRP4 to the degradation of β-catenin, which is known to downregulate the Wnt downstream pro-proliferative genes.41,42
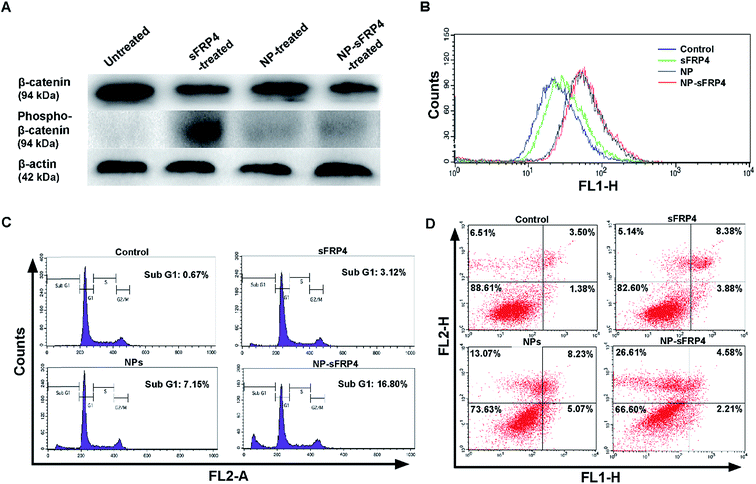 |
| Fig. 4 (A) Targeting of the canonical Wnt/β-catenin signaling with functional sFRP4 was proven by western blotting. β-Catenin was significantly downregulated in sFRP4 and NP-sFRP4 treated HeLa cells, compared to untreated cells; phosphorylated β-catenin, that is, β-catenin marked for degradation, was upregulated in sFRP4-treated cells, however NP-sFRP4 treated cells did not appear to have expressed phospho-β-catenin, possibly due to the presence of potent NPs or due to extremely short half-life of phosphorylated proteins. β-Actin antibody was used as endogenous control. (B) NP- and NP-sFRP4-treated cells showed significant ROS generation in FACS-based assay, and sFRP4-treated samples generated ROS to a lesser extent. (C) Cell cycle analysis using PI recorded a significant increase in sub-G1 population in cells treated with NP-sFRP4, as compared to either sFRP4 or NPs individually, indicating apoptosis. (D) Annexin V-FITC/PI apoptosis detection assay also depicted a significantly higher population of apoptotic cells in NP-sFRP4-treated cells. These experiments demonstrated the co-therapeutic potential of sFRP4 and NPs in this nanosystem. | |
Cytotoxic effect of Ag NC embedded NPs
After elucidating the cause of cell growth inhibition by GST-sFRP4, the mode of effect exerted by the NPs was investigated. Treatment with the Ag NC embedded NPs generated ROS43 that directly correlated with cell death (Fig. 4B).44 However, treatment with protein alone also resulted in ROS generation, albeit much less than that by Ag NCs. Indeed, onset of apoptosis was documented in FESEM images, depicting membrane blebbing and shrinkage of the cells treated with composite NPs for 24 h (ESI Fig. S10†).45 This experiment, along with the above western blotting data were in direct agreement with the results obtained in the cell viability assays. Thus, while Ag NCs induced cell death by ROS generation, recombinant sFRP4 arrested cell growth by blocking the Wnt signaling. Hereby these two components together constituted the co-therapy module, which has been further mechanistically studied.
Co-therapeutic efficacy of sFRP4 bound NPs
In present clinical regime, combination therapy plays a key role in cancer treatment. To overcome the undesirable side-effects of the chemical drugs currently available in the market, novel co-therapy regimes that can specifically target cancer cells are in the process of being developed. In the present study, we focused on the co-therapeutic potential of the NP-sFRP4 nanosystem. Several flow cytometry-based and microscopy-based assays helped to demonstrate the mechanism of cell death in co-therapy module. FACS-based analysis of cell cycle using PI (Fig. 4C) unveiled that the sub-G1 population, which may be considered as an indicator of apoptosis, was significantly higher in NP-sFRP4-treated cells (16.8%), as compared to control (0.67%), sFRP4-treated (3.12%), or NP-treated cells (7.15%). The Ag NC–chitosan–alginate NPs were found to arrest the cells in sub-G1 stage, again indicating apoptosis, which was five-fold compared to sFRP4 treatment, and two-fold as compared to NP treatment. The silver component has been documented in literature to bring about cell death. In this report, we have demonstrated that the NPs exert a cytotoxic effect on cells via ROS generation. Thus affected cells, undergoing cell death (DNA content < 2n) have appeared in the sub-G1 stage in the cell cycle analysis.
Further, FACS based annexin V-FITC/PI assay was done to establish apoptosis as the mechanism of cell death (Fig. 4D). The percentage of apoptotic cells was significantly higher in NP-sFRP4-treated cells (33.4%), versus that of control (11%), sFRP4-treated cells (17.5%), and NP-treated cells (26%). These results are in accordance with a previous report, which showed that restoration of expression of sFRP4 in mesothelioma cells induced apoptotic cell death.46 Also, silver nanoparticles have been proven to induce apoptosis in cancer cells.47
Furthermore, visual corroboration of apoptosis was done by dual staining with AO and EB (ESI Fig. S11†). These images demonstrated that while treatment with the NPs alone showed sufficient number of EB-stained apoptotic cells, co-therapy significantly increased the number of apoptotic cells.
Our investigation initiates an innovative regimen in cancer theranostics, where recombinant therapeutic sFRP4 bound Ag NC–Chi–Alg NPs induced apoptosis in cervical cancer cells via Wnt/β-catenin targeted cancer therapy. Moreover, the intense luminescence of the Ag NCs was utilized in imaging, uptake, and binding analyses. This report lays the foundation for in vivo studies, which could pave the way for clinical applications of the recombinant sFRP4 bound Ag NC–Chi–Alg NPs. Various composite NPs have been investigated in in vivo models with diagnostic as well as therapeutic intensions.48 Composite NPs carrying therapeutic payload have also been documented in literature.49 Although Ag NCs have been previously used for in vivo biological imaging, composite NPs embedded with Ag NCs and carrying a biological macromolecule for the purpose of cancer theranostics has not been designed before. As Wnt pathway inhibitors, viz. chemical inhibitor22 and monoclonal antibody,23 have been successfully employed to combat cancer in mouse models and patient samples, we hypothesize that sFRP4 may also prove to be a potential candidate for clinical usage.
Conclusion
In summary, we have fabricated novel multifaceted theranostic Ag NC–chitosan–alginate NPs bound to recombinant GST-sFRP4 to target downstream molecule of Wnt signaling in cancer cells. While chitosan and alginate could tune the size and surface charge of NPs, the remarkable luminescence of the Ag NCs enable tracking, uptake, and imaging, thus imparting theranostic properties. Moreover, the low toxicity of the Ag NCs50 ensure that they may be possibly employed for in vivo therapy, unlike many other toxic nanoclusters.51 The most intriguing aspect of this work is targeting the central molecule of active Wnt pathway in cancer cells with recombinant purified sFRP4 bound NPs. Interestingly, the bacterially expressed human sFRP4 retained its functionality post purification and binding, evidenced by its anti-proliferative activity and β-catenin regulation downstream of Wnt signaling. Therapeutic efficacy of the NP-sFRP4 has the potential to engender a new strategy for cancer co-therapy, where the advantages of protein therapy could be implemented to reduce the dosage of Ag NCs. In addition, this luminescence based platform could lay the foundation for incorporating dynamic perspectives to the area of cancer theranostics. The independent successes of inhibitors of the Wnt pathway22,23 as well as the noble metal nanoclusters in biological imaging50,52,53 documented in in vivo models have led us to believe that this multifunctional system holds great promise for clinical translation.
Acknowledgements
The financial supports of the Department of Biotechnology Programme Support, (BT/PR13560/COE/34/44/2015), and partial support of the Department of Biotechnology (DBT-NER/Health/47/2015), and the Department of Electronics and Information Technology, (No. 5(9)/2012-NANO (Vol. II)) are greatly acknowledged. Authors acknowledge the support of the Centre for Nanotechnology and the Central Instruments Facility, IIT Guwahati. Authors also deeply appreciate the assistance of Ms Anushree Dutta and Mr Uday Narayan Pan.
References
- B. Leader, Q. J. Baca and D. E. Golan, Nat. Rev. Drug Discovery, 2008, 7, 21–39 CrossRef CAS PubMed.
- T. Stuchinskaya, M. Moreno, M. J. Cook, D. R. Edwards and D. A. Russell, Photochem. Photobiol. Sci., 2011, 10, 822–831 CAS.
- C. D. Mock, B. C. Jordan and C. Selvam, RSC Adv., 2015, 5, 75575–75588 RSC.
- Y. Wang, J. Chen and J. Irudayaraj, ACS Nano, 2011, 5, 9718–9725 CrossRef CAS PubMed.
- E. R. Camp, C. Wang, E. C. Little, P. M. Watson, K. F. Pirollo, A. Rait, D. J. Cole, E. H. Chang and D. K. Watson, Cancer Gene Ther., 2013, 20, 222–228 CrossRef CAS PubMed.
- M. Zheng, P. Zhao, Z. Luo, P. Gong, C. Zheng, P. Zhang, C. Yue, D. Gao, Y. Ma and L. Cai, ACS Appl. Mater. Interfaces, 2014, 6, 6709–6716 CAS.
- J. Y. Yhee, S. J. Lee, S. Lee, S. Song, H. S. Min, S. W. Kang, S. Son, S. Y. Jeong, I. C. Kwon, S. H. Kim and K. Kim, Bioconjugate Chem., 2013, 24, 1850–1860 CrossRef CAS PubMed.
- S. Balasubramanian, A. R. Girija, Y. Nagaoka, S. Iwai, M. Suzuki, V. Kizhikkilot, Y. Yoshida, T. Maekawa and S. D. Nair, Int. J. Nanomed., 2014, 9, 437–459 Search PubMed.
- S. Banerjee, A. K. Sahoo, A. Chattopadhyay and S. S. Ghosh, RSC Adv., 2013, 3, 14123–14131 RSC.
- N. Chaubey, A. K. Sahoo, A. Chattopadhyay and S. S. Ghosh, Biomater. Sci., 2014, 2, 1080–1089 RSC.
- J. N. Anastas and R. T. Moon, Nat. Rev. Cancer, 2013, 13, 11–26 CrossRef CAS PubMed.
- H. Suzuki, D. N. Watkins, K. W. Jair, K. E. Schuebel, S. D. Markowitz, W. D. Chen, T. P. Pretlow, B. Yang, Y. Akiyama, M. Van Engeland, M. Toyota, T. Tokino, Y. Hinoda, K. Imai, J. G. Herman and S. B. Baylin, Nat. Genet., 2004, 36, 417–422 CrossRef CAS PubMed.
- O. Tetsu and F. McCormick, Nature, 1999, 398, 422–426 CrossRef CAS PubMed.
- T. Zhang, T. Otevrel, Z. Gao, Z. Gao, S. M. Ehrlich, J. Z. Fields and B. M. Boman, Cancer Res., 2001, 61, 8664–8667 CAS.
- T. C. He, A. B. Sparks, C. Rago, H. Hermeking, L. Zawel, L. T. da Costa, P. J. Morin, B. Vogelstein and K. W. Kinzler, Science, 1998, 281, 1509–1512 CrossRef CAS PubMed.
- P. Neth, C. Ries, M. Karow, V. Egea, M. Ilmer and M. Jochum, Stem Cell Rev., 2007, 3, 18–29 CrossRef CAS PubMed.
- R. Surana, S. Sikka, W. Cai, E. M. Shin, S. R. Warrier, H. J. Tan, F. Arfuso, S. A. Fox, A. M. Dharmarajan and A. P. Kumar, Biochim. Biophys. Acta, 2014, 1845, 53–65 CAS.
- L. G. Horvath, S. M. Henshall, J. G. Kench, D. N. Saunders, C. S. Lee, D. Golovsky, P. C. Brenner, G. F. O'Neill, R. Kooner, P. D. Stricker, J. J. Grygiel and R. L. Sutherland, Clin. Cancer Res., 2004, 10, 615–625 CrossRef CAS PubMed.
- U. Saran, F. Arfuso, N. Zeps and A. Dharmarajan, BMC Cell Biol., 2012, 13, 25 CrossRef CAS PubMed.
- R. Maganga, N. Giles, K. Adcroft, A. Unni, D. Keeney, F. Wood, M. Fear and A. Dharmarajan, Biochem. Biophys. Res. Commun., 2008, 377, 606–611 CrossRef CAS PubMed.
- S. Warrier, S. K. Balu, A. P. Kumar, M. Millward and A. Dharmarajan, Oncol. Res., 2013, 21, 93–102 CrossRef PubMed.
- M. Wickstrom, C. Dyberg, J. Milosevic, C. Einvik, R. Calero, B. Sveinbjornsson, E. Sanden, A. Darabi, P. Siesjo, M. Kool, P. Kogner, N. Baryawno and J. I. Johnsen, Nat. Commun., 2015, 6, 8904 CrossRef PubMed.
- A. Gurney, F. Axelrod, C. J. Bond, J. Cain, C. Chartier, L. Donigan, M. Fischer, A. Chaudhari, M. Ji, A. M. Kapoun, A. Lam, S. Lazetic, S. Ma, S. Mitra, I.-K. Park, K. Pickell, A. Sato, S. Satyal, M. Stroud, H. Tran, W.-C. Yen, J. Lewicki and T. Hoey, Proc. Natl. Acad. Sci. U. S. A., 2012, 109, 11717–11722 CrossRef CAS PubMed.
- H. Jiang, G. Xu, Y. Sun, W. Zheng, X. Zhu, B. Wang, X. Zhang and G. Wang, Chem. Commun., 2015, 51, 11810–11813 RSC.
- B. Adhikari and A. Banerjee, Chem. Mater., 2010, 22, 4364–4371 CrossRef CAS.
- A. Ghoshal and S. S. Ghosh, Mol. Cell. Biochem., 2016, 418, 119–135 CrossRef CAS PubMed.
- J. R. Lakowicz, Principles of Fluorescence Spectroscopy, Springer, New York, 2006 Search PubMed.
- S. E. Jones and C. Jomary, BioEssays, 2002, 24, 811–820 CrossRef CAS PubMed.
- C. E. Dann, J. C. Hsieh, A. Rattner, D. Sharma, J. Nathans and D. J. Leahy, Nature, 2001, 412, 86–90 CrossRef CAS PubMed.
- A. Albanese, P. S. Tang and W. C. Chan, Annu. Rev. Biomed. Eng., 2012, 14, 1–16 CrossRef CAS PubMed.
- A. Ghoshal, U. Goswami, A. K. Sahoo, A. Chattopadhyay and S. S. Ghosh, ACS Biomater. Sci. Eng., 2015, 1, 1256–1266 CrossRef CAS.
- I. Diez, M. Pusa, S. Kulmala, H. Jiang, A. Walther, A. S. Goldmann, A. H. Muller, O. Ikkala and R. H. Ras, Angew. Chem., Int. Ed., 2009, 48, 2122–2125 CrossRef CAS PubMed.
- I. Diez and R. H. A. Ras, Nanoscale, 2011, 3, 1963–1970 RSC.
- C. Remuñán-López and R. Bodmeier, J. Controlled Release, 1997, 44, 215–225 CrossRef.
- A. Y. Lee, B. He, L. You, S. Dadfarmay, Z. Xu, J. Mazieres, I. Mikami, F. McCormick and D. M. Jablons, Oncogene, 2004, 23, 6672–6676 CrossRef CAS PubMed.
- A. Raza, V. Kohila and S. S. Ghosh, J. Gene Med., 2015, 17, 132–139 CrossRef CAS PubMed.
- J. Li, X. Zhong, F. Cheng, J.-R. Zhang, L.-P. Jiang and J.-J. Zhu, Anal. Chem., 2012, 84, 4140–4146 CrossRef CAS PubMed.
- R. Foldbjerg, D. A. Dang and H. Autrup, Arch. Toxicol., 2011, 85, 743–750 CrossRef CAS PubMed.
- A. Y. Lee, B. He, L. You, S. Dadfarmay, Z. Xu, J. Mazieres, I. Mikami, F. McCormick and D. M. Jablons, Oncogene, 2004, 23, 6672–6676 CrossRef CAS PubMed.
- T. Berndt, T. A. Craig, A. E. Bowe, J. Vassiliadis, D. Reczek, R. Finnegan, S. M. Jan De Beur, S. C. Schiavi and R. Kumar, J. Clin. Invest., 2003, 112, 785–794 CrossRef CAS PubMed.
- J. Zhang, A. J. Gill, J. D. Issacs, B. Atmore, A. Johns, L. W. Delbridge, R. Lai and T. P. McMullen, Hum. Pathol., 2012, 43, 1044–1050 CrossRef CAS PubMed.
- H.-Y. Jung, S. Jun, M. Lee, H.-C. Kim, X. Wang, H. Ji, P. D. McCrea and J.-I. Park, Mol. Cell, 2013, 52, 193–205 CrossRef CAS PubMed.
- X. Yuan, M. I. Setyawati, A. S. Tan, C. N. Ong, D. T. Leong and J. Xie, NPG Asia Mater., 2013, 5, e39 CrossRef CAS.
- D. Guo, L. Zhu, Z. Huang, H. Zhou, Y. Ge, W. Ma, J. Wu, X. Zhang, X. Zhou, Y. Zhang, Y. Zhao and N. Gu, Biomaterials, 2013, 34, 7884–7894 CrossRef CAS PubMed.
- S. Elmore, Toxicol. Pathol., 2007, 35, 495–516 CrossRef CAS PubMed.
- B. He, A. Y. Lee, S. Dadfarmay, L. You, Z. Xu, N. Reguart, J. Mazieres, I. Mikami, F. McCormick and D. M. Jablons, Cancer Res., 2005, 65, 743–748 CAS.
- P. Sanpui, A. Chattopadhyay and S. S. Ghosh, ACS Appl. Mater. Interfaces, 2011, 3, 218–228 CAS.
- A. P. Subramanian, S. K. Jaganathan and E. Supriyanto, RSC Adv., 2015, 5, 72638–72652 RSC.
- J. H. Lee, K. Lee, S. H. Moon, Y. Lee, T. G. Park and J. Cheon, Angew. Chem., Int. Ed., 2009, 48, 4174–4179 CrossRef CAS PubMed.
- S. Gao, D. Chen, Q. Li, J. Ye, H. Jiang, C. Amatore and X. Wang, Sci. Rep., 2014, 4, 4384 Search PubMed.
- L. Wu and X. Qu, Chem. Soc. Rev., 2015, 44, 2963–2997 RSC.
- X. Wu, X. He, K. Wang, C. Xie, B. Zhou and Z. Qing, Nanoscale, 2010, 2, 2244–2249 RSC.
- J.-M. Liu, J.-T. Chen and X.-P. Yan, Anal. Chem., 2013, 85, 3238–3245 CrossRef CAS PubMed.
Footnote |
† Electronic supplementary information (ESI) available. See DOI: 10.1039/c6ra16066b |
|
This journal is © The Royal Society of Chemistry 2016 |