DOI:
10.1039/C6RA15404B
(Paper)
RSC Adv., 2016,
6, 71006-71011
Graphene-coated mesoporous Co3O4 fibers as an efficient anode material for Li-ion batteries†
Received
14th June 2016
, Accepted 20th July 2016
First published on 21st July 2016
Abstract
Herein, we have designed graphene-coated porous cobalt oxide fibers (Co3O4@G) using coordination polymers as precursors through calcination followed by a subsequent self-assembly process. The graphene-coated porous cobalt oxide fiber nanostructures not only provide good conductivity, but also prevent the aggregation and volume change of the Co3O4 nanoparticles during lithium storage processes. When serving as anode materials in the lithium-ion batteries, the obtained Co3O4@G composites, exhibit remarkable electrochemical performances, including high specific capacity (∼1303.9 mA h g−1 at a current density of 0.2 A g−1), excellent cycling stability (1153 mA h g−1 retention after 80 cycles at a current density of 0.2 A g−1) and high rate capability.
Introduction
Lithium-ion batteries (LIBs) have been widely applied in portable electronic equipment and electric vehicles and continue to attract wide research interest, due to their high energy and power density, excellent cycling performance, and environmental friendliness.1–5 To fulfill the emerging need, it is still important to explore novel electrode materials with high capacity and excellent cycling performance. Transition metal oxides, such as NiO, Fe2O3, Fe3O4, CoO, Co3O4, MnO, MnO2, Mn2O3, etc.6–13 have drawn great attention because of their higher theoretical capacity (>600 mA h g−1) than that of commercial graphite (372 mA h g−1) as well as the intrinsically enhanced safety.14,15 Among these transition metal oxides, cobalt oxide (Co3O4), which has a theoretical capacity of 890 mA h g−1 according to the reversible conversion reaction of Co3O4 + 8Li+ + 8e− ↔ 3Co + 4Li2O,16–22 has been regarded as a promising anode for LIBs however, large volume change during the Li+ insertion and extraction processes, which will eventually leads to a large irreversible capacity loss and poor cycling stability,23 still hinder its practical application. To overcome these issues, enormous efforts have been devoted to designing different nanostructures of Co3O4 such as nanoparticles,24 nanotube,25 nanoflakes,26 nanocage,27 and nanosheets,28 which can effectively shorten Li+ diffusion length in order to improve the electrochemical performance.
As the low intrinsic electrical conductivity of Co3O4 anodes is the major issue, preparing porous nanostructured Co3O4 with carbonaceous materials is an effective strategy to improve the electrical conductivity.29–33 Coordination polymers are constructed from the coordination of inorganic metal cations and multitopic organic ligands and they have been proved to be a beneficial precursor or sacrificial template to synthesize porous nano/micro-architectured metal oxides.34,35 In addition, graphene, which possesses electrical conductivity, large surface area (2630 m2 g−1), mechanical robustness, and excellent chemical stability, is one of the most appealing matrices for supporting nanoparticles for application in LIBs.31,36 Motivated by this intriguing idea, it is speculated that mesoporous Co3O4 nanowires derived from metal coordination polymer with graphene integument will offer unprecedented electrochemical performances in LIBs.
In this paper, we report a facile strategy to prepare graphene-coating porous cobalt oxide fibers (Co3O4@G). The graphene matrix not only allow easy access of Li+ in the resultant structure but also offer the excellent and stable electrical contact between the active material and electrolytes. It is worth mentioning that the graphene-coating porous hollow structure acts as a compliant buffer to provide sufficient space for accommodating the volume expansion upon Li+ insertion/extraction.36 The obtained Co3O4@G nanocomposites, when used as anode materials in the LIBs, deliver a high specific capacity of ∼1303.9 mA h g−1 at a current density of 0.2 A g−1. At a current density of 0.2 A g−1, a capacity of 1153 mA h g−1 is still maintained after 80 cycles, demonstrating excellent cycling stability as well as high rate capability.
Results and discussion
The (polymer–cobalt) precursor is obtained through a simple hydrothermal method which use cobalt chloride and nitrilotriacetic acid (NTA) as the source (referred as Co–NTA fibers). The morphologies and structures of the Co–NTA fibers are revealed by scanning electron microscopy (SEM) and transmission electron microscopy (TEM), respectively. As shown in Fig. 1a and b, the Co–NTA fibers have smooth surfaces with the average diameter of about 200 nm, which are further confirmed by the TEM images (Fig. 1c and d). The thermal stability of Co–NTA fibers is investigated by thermogravimetric analysis (TGA) at a temperature range from 25 °C to 800 °C with a heating rate of 10 °C min−1 in the air atmosphere (Fig. S1†). The TGA curve shows two major weight loss steps, which might be corresponded first to the loss of physically adsorbed water and then the decomposition of the Co–NTA fibers composites, respectively. The composite is transformed into porous Co3O4 fibers by annealing at 500 °C for 2 h in air. As can be observed from the SEM images (Fig. 2a and b), the overall fiber morphology are well preserved with abundant rough which are consisted of interconnected Co3O4 nanocrystals compared with the smooth precursors. The detailed architecture of the Co3O4 fibers is furthermore investigated by TEM. It can be clearly seen from Fig. 2c that the fibers are composed of interconnected nanoparticles, which are organized into the porous structure probably due to the release of the CO2 gas during the annealing process. The high crystallinity and phase of porous Co3O4 fibers are confirmed by XRD, as shown in Fig. S2,† which is consistent with the pure Co3O4 phase (JCPDS no. 42-1467).24
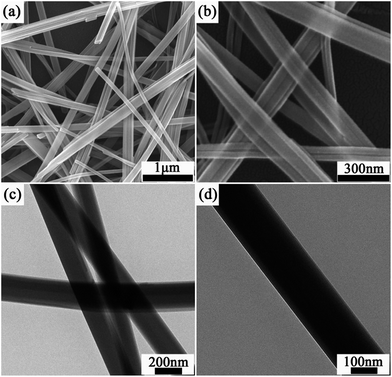 |
| Fig. 1 SEM (a, b) and TEM (c, d) images of the Co–NTA fibers. | |
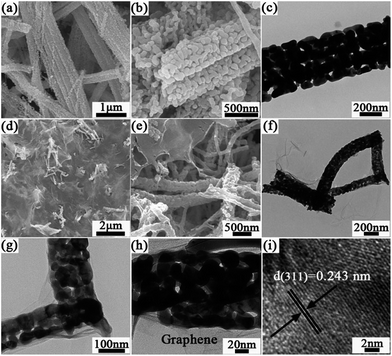 |
| Fig. 2 SEM (a, b) and TEM (c) images of porous Co3O4 fibers. SEM (d, e), TEM (f–h) and high-magnification TEM images (i) of Co3O4@G. | |
The Co3O4@G fibers were fabricated through a self-assembly process with subsequent calcination and the morphologies were analysed by SEM and TEM. In Fig. 2d and e, the Co3O4 fibers uniformly distributed in the graphene matrix and are well wrapped by the corrugated and rough graphene network. Fig. 2f–h show the TEM images of the mesoporous Co3O4@G fibers. The mesoporous Co3O4 are coated with uniform layer of graphene. Such structures can provide the room for the volume variation upon Li+ insertion/extraction. The HRTEM image shows the lattice spacing of 0.243 nm, which is corresponding to the spacing of (311) atomic planes of Co3O4 (Fig. 2i). Such a unique structure of graphene-coating porous cobalt oxide fibers can effectively prevent Co3O4 particles from aggregation and enhance electrode stability. Furthermore, the high conductivity graphene could facilitate fast transport of electron and lithium ions, leading to good rate performance.
The crystallographic structure and composition of the Co3O4@G samples are characterized by using XRD (Fig. 3a). As can be seen, all of the peaks can be indexed to the cubic Co3O4 phase (JCPDS no. 42-1467) without any detectable crystalline impurity, indicating that the Co–NTA precursor has been converted to crystalline Co3O4 completely.37 An additional broad and small diffraction peak appears at around 23° in the XRD patterns, which is indexed into the disorderedly graphene layers.36 Based on the SEM, TEM and XRD results, it can be concluded that the Co3O4@G nanocomposites have been successfully prepared. The mesoporous feature of the Co3O4@G composites is further confirmed by N2 adsorption–desorption analysis (Fig. 3b). The nitrogen adsorption/desorption isotherms curves are classified as type IV isotherm curves with a distinct hysteresis loop at the relative pressure range of 0.45–0.97 P/P0.29 This result indicates that the Co3O4@G composites exhibit mesoporous nature, which is in accordance with the TEM images (Fig. 2g and h). The BET specific surface area of Co3O4@G composites is calculated to be about 27 m2 g−1. The average pore size of Co3O4@G composites is approximately 12.8 nm according to the Barrett–Joyner–Halenda (BJH) mode (Fig. S3†).
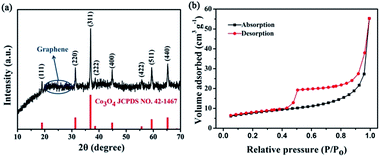 |
| Fig. 3 (a) XRD pattern of porous Co3O4@G composites. (b) Nitrogen adsorption–desorption isotherms of the Co3O4@G nanohybrids. | |
X-ray photoelectron spectroscopy (XPS) analysis was carried out to investigate the surface chemical information of the Co3O4@G composites. As shown in Fig. 4a, three main peaks at about 285.2, 531.5 and 780.6 eV, which correspond to the peaks of C 1s, O 1s and Co 2p, respectively, are observed. The Co 2p XPS spectrum (Fig. 4b) can be divided into two peaks at 795.0 and 779.8 eV with a spin energy separation of 15.2 eV. The two peaks can be ascribed to Co 2p3/2 and 2p1/2, respectively, which indicates the expansion of Co3O4.38 Fig. 4c reveals the XPS spectrum of O 1s, which can be deconvoluted into three peaks centred at 529.8, 531.8 and 533.4 eV, which are related to Co–O, C–O and C
O bonds, respectively.39 The XPS spectrum of C 1s peaks at 284.7, 286.1 and 288.8 eV can be assigned to C–C, C–O and O–C
O bonds, respectively (Fig. 4d).31
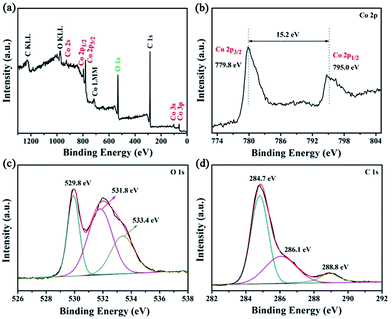 |
| Fig. 4 XPS spectra for the Co3O4@G composites: (a) the survey spectrum and the high resolution spectra for (b) Co 2p, (c) O 1s and (d) C 1s. | |
The initial three cyclic voltammogram (CV) curves of the Co3O4@G compositions are presented in Fig. 5a. In the first cathodic scan, the sharp peak located at around 0.8 V is attributed to the reduction of Co3O4 to metallic cobalt and the formation of Li2O as well as the formation of the solid electrolyte interphase (SEI) film.40 In the subsequent anodic scan, located peak at around 2.1 V appear which can be ascribed to the delithiation reaction and the decomposition of Li2O.41 In the subsequent cycles, the CV curves overlap with each other, exhibiting good reproducibility, which suggests high electrochemical stability of Co3O4@G compositions. The typical charge and discharge profiles of Co3O4@G electrodes at 0.2 A g−1 in the voltage range of 0.01–3.0 V are illustrated in Fig. 5b. In the first cycle, the electrodes of Co3O4@G deliver the specific charge and discharge capacities of 1028.4 and 1303.9 mA h g−1, respectively.42,43 The initial columbic efficiency is as high as 78.9%.44 In Fig. 5a, both charge and discharge curves overlap at the subsequent two cycles, which further confirms the good reversibility of Co3O4@G.
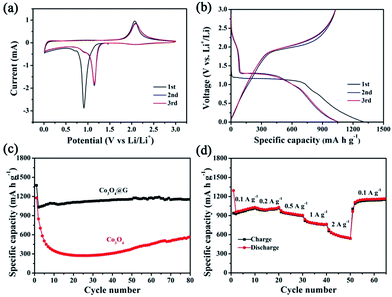 |
| Fig. 5 (a) CV curves of Co3O4@G nanocomposite obtained at a scan rate of 0.1 mV s−1 between 0.01 and 3 V. (b) Charge–discharge curves of Co3O4@G nanocomposite at a constant current density of 0.2 A g−1. (c) Cycling performance and corresponding coulombic efficiency of Co3O4@G nanocomposite measured at 0.2 A g−1. The cycling performance of Co3O4 nanocomposite electrodes is also presented. (d) Rate performance of Co3O4@G nanocomposite electrode at various current densities. | |
Fig. 5c depicts the cycling performance of Co3O4@G and pure Co3O4 at 0.2 A g−1. It is found that the Co3O4@G electrodes exhibit much better electrochemical performances than that of pure Co3O4 after 80 cycles, the capacity of Co3O4@G is as high as 1153 mA h g−1 with a coulombic efficiency of approximately 99% (Fig. S4†), whereas the reversible capacity of pure Co3O4 decreases to 566 mA h g−1. The improved cycling stability and the higher capacity of the Co3O4@G nanocomposites may be due to the synergetic effects of the two components of the composites. Firstly, the graphene matrix not only provides good conductivity but also render intimate electrical contact between the active material and electrolytes. Secondly, the porous structure of Co3O4 fibers can effectively prevent the Co3O4 NPs from agglomerating upon Li+ insertion/extraction. It is also noted that the Co3O4@G electrodes exhibit excellent rate capability, as shown in Fig. 5d. The Co3O4@G nanocomposites achieve capacities of 1029.2, 1029.6, 904.5, 765.7 and 544.3 mA h g−1 at the current densities of 0.1, 0.2, 0.5, 1 and 2 A g−1, respectively. Moreover, the capacity of 1109.3 mA h g−1 can be obtained when the current density restores to 0.1 A g−1, indicating good structural stability and electrochemical reversibility of Co3O4@G electrodes. The morphology of the Co3O4@G electrode after 80 cycles at 0.2 A g−1 was investigated by SEM and the results are shown in Fig. S5,† which confirms the good structural stability of Co3O4@G. Electrochemical impedance spectroscopy (EIS) measurements of Co3O4@G and pure Co3O4 electrodes were performed. Both Nyquist plots (Fig. 6) are composed of a medium-to-high frequency depressed semicircle and a low-frequency straight line, which can be ascribed to the charge-transfer resistance (Rct) and the mass transfer of lithium ions.45,46 Apparently, charge-transfer resistance (Rct) of Co3O4@G electrode is ∼50 Ω smaller than that of the Co3O4 electrode, which confirms that the graphene can provide efficient electron conduction of the overall electrode.
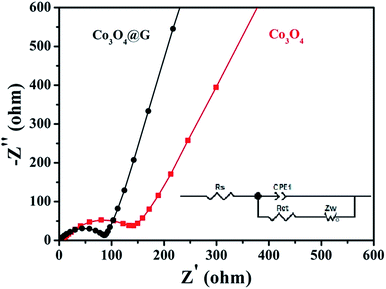 |
| Fig. 6 Electrochemical impedance spectroscopy plots of Co3O4 and Co3O4@G nanohybrids. | |
In conclusion, the improved performance could be attributed to the smart design of graphene-coating porous cobalt oxide fibers. Firstly, the hierarchical graphene serves as a conductive matrix and maintains the structural integrity of the electrode by preventing aggregation of crystalline Co3O4 fibers. Secondly, graphene matrix not only offer direct electrical contact between the active material and the electrically conducting carbon additive, but also acts as a compliant buffer to accommodate the volume variation upon Li+ insertion/extraction.36 Last but not least, the unique porous architecture of interconnected Co3O4 nanocrystals can facilitate the transport of Li+ ions and electrons, thus enhancing the electrochemical reversibility.18
Experimental
All reagents are of analytical purity and used without further purification.
Synthesis of cobalt coordination polymer fibers (Co–NTA)
Typically, 4.76 g of cobalt chloride (CoCl2·6H2O) and 1.2 g of nitrilotriacetic acid (NTA) were added to a mixed solution with 18 mL isopropanol and 54 mL distilled water, and formed a pink solution under magnetic stirring for 30 min. The solution was transferred into a Teflon-lined stainless steel autoclave, and then was sealed and maintained at 180 °C for 6 h. After cooling down to room temperature, the as-prepared product were washed with distilled water and ethanol several times, and dried in a vacuum oven at 80 °C overnight.
Synthesis of cobalt oxide fibers (Co3O4)
In a typical experiment, the porous Co3O4 fibers are obtained through an annealing treatment. The sample was calcined in a muffle oven at 500 °C for 2 h in the air atmosphere. Then the furnace was naturally air cooled to room temperature.
Synthesis of cobalt oxide–graphene nanohybrids (Co3O4@G)
In a typical experiment,30,47 the as-prepared porous Co3O4 fibers (80 mg) were dispersed in 50 mL ethanol by ultrasonication. Then, 0.5 mL of aminopropyltrimethoxysilane (APS) was stepwise added to the above solution with strong stirring for 6 h. The APS-modified porous Co3O4 fibers were obtained by centrifugation and then re-dispersed in 70 mL water under magnetic stirring. After that, 60 mL of GO aqueous solution (0.5 mg mL−1) by a modified Hummers method were added into the suspension with continuous stirring for 6 h. Subsequently, 0.5 mL hydrazine (80 wt%) was added to the precursor solution and kept stirring for 12 h. Finally, the product was centrifuged and washed several times with water, and then dried at 60 °C in a vacuum oven for 12 h to obtain the black Co3O4@G nanohybrids.
Materials characterization
The morphologies and structure of the products were observed using scanning electron microscope (SEM, Hitachi S-4700) and transmission electron microscopy (TEM, Tecnai G220, FEI, American). The high-resolution TEM (HRTEM) was carried out by a Tecnai G2 F20 S-TWIN. The crystalline structure of the products were also carried out by X-ray diffraction (XRD) using an X'Pert-Pro diffractometer (Netherlands PANalytical) with a Cu Kα radiation (λ = 1.540598 Å). X-ray photoelectron spectroscopy (XPS) was recorded on the Escalab250Xi (UK) using a monochromatic AlKα X-ray radiation as the excitation source with a hemispherical electron energy analyzer. The Barrett–Joyner–Halenda (BJH) surface areas were measured at 77 K using an Autosorb-1 system (Quanta Chrome).
Electrochemical measurements
The electrochemical measurements were measured at room temperature using a coin-type half cell (CR 2016). The test electrodes were fabricated by mixing active materials (80 wt%), carbon black (10 wt%), and polyvinylidene fluoride (10 wt%) dissolved in N-methyl-2-pyrrolidone to form a slurry, which was uniformly coated onto a copper foil, and then dried in vacuum at 110 °C for 12 h. The Half cells were performed in an argon-filled glovebox with the presence percent of moisture and oxygen below 0.1 ppm, using Li foil as the counter electrode, Celgard 2300 membrane as the separator, and 1 M LiPF6 in a mixture of ethylene carbonate (EC) and dimethyl carbonate (DMC) in the volume ratio of 1
:
1 as the electrolyte. The charge–discharge tests were carried out in the potential range of 0.01–3 V. The cyclic voltammetry (CV) was obtained in the range of 0.01–3.0 V (vs. Li+/Li) with a scanning rate of 0.1 mV s−1, and the electrochemical impedance spectroscopy (EIS) was carried out over the frequency range of 0.01–100 kHz with an amplitude of 10 mV on CS350 electrochemical workstation.
Conclusions
In summary, graphene-coating porous cobalt oxide fibers (Co3O4@G) have been successfully fabricated through using coordination polymers as precursor by a self-assembly process with subsequent calcination. The as-obtained Co3O4@G nanocomposites exhibit remarkable electrochemical performances, including high specific capacity (∼1303.9 mA h g−1 at a current density of 0.2 A g−1), excellent cycling stability (1153 mA h g−1 retention after 80 cycles at a current density of 0.2 A g−1) and high rate capability. Interestingly, this strategy can be expanded to developing other graphene-coating mesoporous metal compounds composites as an anode material for next generation of high-performance lithium-ion batteries (LIBs).
Acknowledgements
This research was supported by the National Natural Science Foundation of China (No. 21373006), and the Priority Academic Program Development of Jiangsu Higher Education Institutions.
Notes and references
- J. M. Tarascon and M. Armand, Nature, 2001, 414, 359–367 CrossRef CAS PubMed.
- H. Li, Z. X. Wang, L. Q. Chen and X. J. Huang, Adv. Mater., 2009, 21, 4593–4607 CrossRef CAS.
- H. Zhang, X. Yu and P. V. Braun, Nat. Nanotechnol., 2011, 6, 277–281 CrossRef CAS PubMed.
- Y. Liu, M. Clark, Q. Zhang, D. Yu, D. Liu, J. Liu and G. Cao, Adv. Energy Mater., 2011, 1, 194–202 CrossRef CAS.
- V. Etacheri, R. Marom, R. Elazari, G. Salitra and D. Aurbach, Energy Environ. Sci., 2011, 4, 3243–3262 CAS.
- Z. C. Bai, Z. C. Ju, C. L. Guo, Y. T. Qian, B. Tang and S. L. Xiong, Nanoscale, 2014, 6, 3268–3273 RSC.
- B. Z. Yu, X. L. Liu, H. G. Zhang, G. Y. Jing, P. Ma, Y. Luo, W. M. Xue, Z. Y. Ren and H. M. Fan, J. Mater. Chem. A, 2015, 3, 16544–16550 CAS.
- Y. Chen, B. H. Song, M. Li, L. Lu and J. M. Xue, Adv. Funct. Mater., 2014, 24, 319–326 CrossRef CAS.
- X. Guan, J. W. Nai, Y. P. Zhang, P. X. Wang, J. Yang, L. R. Zheng, J. Zhang and L. Guo, Chem. Mater., 2014, 26, 5958–5964 CrossRef CAS.
- D. H. Ge, H. B. Geng, J. Q. Wang, J. W. Zheng, Y. Pan, X. Q. Cao and H. W. Gu, Nanoscale, 2014, 6, 9689–9694 RSC.
- Y. M. Sun, X. L. Hu, W. Luo, F. F. Xia and Y. H. Huang, Adv. Funct. Mater., 2013, 23, 2436–2444 CrossRef CAS.
- L. F. Chen, Z. H. Huang, H. W. Liang, Q. F. Guan and S. H. Yu, Adv. Mater., 2013, 25, 4746–4752 CrossRef CAS PubMed.
- Y. J. Zhang, Y. Yan, X. Y. Wang, G. Li, D. R. Deng, L. Jiang, C. Y. Shu and C. R. Wang, Chem.–Eur. J., 2014, 20, 6126–6130 CrossRef CAS PubMed.
- X. H. Rui, H. T. Tan and Q. Y. Yan, Nanoscale, 2014, 6, 9889–9924 RSC.
- Y. Huang, X. Huang, J. Lian, D. Xu, L. Wang and X. Zhang, J. Mater. Chem., 2012, 22, 2844–2847 RSC.
- P. Poizot, S. Laruelle, S. Grugeon and J. M. Tarascon, J. Electrochem. Soc., 2002, 149, A1212–A1217 CrossRef CAS.
- X. W. Lou, D. Deng, J. Y. Lee and L. A. Archer, J. Mater. Chem., 2008, 18, 4397–4401 RSC.
- J. Mujtaba, H. Y. Sun, G. Y. Huang, K. Mølhave, Y. G. Liu, Y. Y. Zhao, X. Wang, S. M. Xu and J. Zhu, Sci. Rep., 2016, 6, 20592 CrossRef CAS PubMed.
- Z. S. Wu, W. C. Ren, L. Wen, L. B. Gao, J. P. Zhao, Z. P. Chen, G. M. Zhou, F. Li and H. M. Cheng, ACS Nano, 2010, 4, 3187–3194 CrossRef CAS PubMed.
- H. L. Wang, N. Mao, J. Shi, Q. G. Wang, W. H. Yu and X. Wang, ACS Appl. Mater. Interfaces, 2015, 7, 2882–2890 CAS.
- Y. L. Tan, Q. M. Gao, C. X. Yang, K. Yang, W. Q. Tian and L. H. Zhu, Sci. Rep., 2015, 5, 12382 CrossRef CAS PubMed.
- M. V. Reddy, G. V. Subba Rao and B. V. R. Chowdari, Chem. Rev., 2013, 113, 5364–5457 CrossRef CAS PubMed.
- J. Jiang, Y. Li, J. Liu, X. Huang, C. Yuan and X. W. D. Lou, Adv. Mater., 2012, 24, 5166–5180 CrossRef CAS PubMed.
- D. L. Wang, Y. C. Yu, H. He, J. Wang, W. D. Zhou and H. D. Abruña, ACS Nano, 2015, 9, 1775–1781 CrossRef CAS PubMed.
- Z. T. Cui, S. G. Wang, Y. H. Zhang and M. H. Cao, Electrochim. Acta, 2015, 182, 507–515 CrossRef CAS.
- S. Q. Chen, Y. F. Zhao, B. Sun, Z. M. Ao, X. Q. Xie, Y. Y. Wei and G. X. Wang, ACS Appl. Mater. Interfaces, 2015, 7, 3306–3313 CAS.
- Y. Wang, B. F. Wang, F. Xiao, Z. G. Huang, Y. J. Wang, C. Richardson, Z. X. Chen, L. F. Jiao and H. T. Yuan, J. Power Sources, 2015, 298, 203–208 CrossRef CAS.
- M. Y. Son, J. H. Kim and Y. C. Kang, Electrochim. Acta, 2014, 116, 44–50 CrossRef CAS.
- X. N. Leng, S. F. Wei, Z. H. Jiang, J. S. Lian, G. Y. Wang and Q. Jiang, Sci. Rep., 2015, 5, 16629 CrossRef CAS PubMed.
- G. Huang, F. F. Zhang, X. C. Du, Y. L. Qin, D. M. Yin and L. M. Wang, ACS Nano, 2015, 2, 1592–1599 CrossRef PubMed.
- H. B. Geng, Y. Y. Guo, X. G. Ding, H. W. Wang, Y. F. Zhang, X. L. Wu, J. Jiang, J. W. Zheng, Y. G. Yang and H. W. Gu, Nanoscale, 2016, 8, 7688–7694 RSC.
- Y. M. Chen, L. Yu and X. W. Lou, Angew. Chem., Int. Ed., 2016, 55, 1–5 CrossRef.
- D. F. Qiu, G. Bu, B. Zhao, Z. X. Lin, L. Pu, L. J. Pan and Y. Shi, Mater. Lett., 2014, 119, 12–15 CrossRef CAS.
- R. B. Wu, X. K. Qian, K. Zhou, J. Wei, J. Lou and P. M. Ajayan, ACS Nano, 2014, 6, 6297–6303 CrossRef PubMed.
- H. W. Song, L. S. Shen and C. X. Wang, J. Mater. Chem. A, 2014, 2, 20597–20604 CAS.
- X. L. Yang, K. C. Fan, Y. H. Zhu, J. H. Shen, X. Jiang, P. Zhao, S. R. Luan and C. Z. Li, ACS Appl. Mater. Interfaces, 2013, 5, 997–1002 CAS.
- C. S. Yan, G. Chen, X. Zhou, J. X. Sun and C. D. Lv, Adv. Funct. Mater., 2016, 26, 1428–1436 CrossRef CAS.
- B. Wang, X. Y. Lu, Y. Y. Tang and W. W. Ben, ChemElectroChem, 2016, 3, 55–65 CrossRef CAS.
- Z. S. Wu, W. C. Ren, L. Wen, L. B. Gao, J. P. Zhao, Z. P. Chen, G. M. Zhou, F. Li and H. M. Cheng, ACS Nano, 2010, 4, 3187–3194 CrossRef CAS PubMed.
- H. Huang, W. J. Zhu, X. Y. Tao, Y. Xia, Z. Y. Yu, J. W. Fang, Y. P. Gan and W. K. Zhang, ACS Appl. Mater. Interfaces, 2012, 4, 5974–5980 CAS.
- L. Li, G. M. Zhou, X.-Y. Shan, S. F. Pei, F. Li and H.-M. Cheng, J. Power Sources, 2014, 255, 52–58 CrossRef CAS.
- B. Wang, X.-Y. Lu and Y. Tang, J. Mater. Chem. A, 2015, 3, 9689–9699 CAS.
- X. Li, G. L. Xu, F. Fu, Z. Lin, Q. Wang, L. Huang, J. T. Li and S. G. Sun, Electrochim. Acta, 2013, 96, 134–140 CrossRef CAS.
- Y. J. Chen, M. Zhuo, J. W. Deng, Z. Xu, Q. H. Li and T. H. Wang, J. Mater. Chem. A, 2014, 2, 4449–4456 CAS.
- G. M. Zhou, D.-W. Wang, L. Li, N. Li, F. Li and H.-M. Cheng, Nanoscale, 2015, 5, 1576–1582 RSC.
- X. J. Xu, S. M. Ji, M. Z. Gu and J. Liu, ACS Appl. Mater. Interfaces, 2015, 7, 20957–20964 CAS.
- W. B. Luo, S. L. Chou, Y. C. Zhai and H. K. Liu, J. Mater. Chem. A, 2014, 2, 4927–4931 CAS.
Footnote |
† Electronic supplementary information (ESI) available. See DOI: 10.1039/c6ra15404b |
|
This journal is © The Royal Society of Chemistry 2016 |